- 1Samueli School of Engineering, University of California Los Angeles, Los Angeles, CA, United States
- 2Lenfest Center for Sustainable Energy and Department of Environmental Engineering, Columbia University, New York, NY, United States
- 3Center on Global Energy Policy, Columbia University, New York, NY, United States
- 4Department of Biology, University of New Mexico, Albuquerque, NM, United States
- 5Department of Civil Engineering and Engineering Mechanics, Columbia University, New York, NY, United States
- 6Department of Mechanical Engineering, University of Michigan, Ann Arbor, MI, United States
- 7Department of Civil, Construction and Environmental Engineering, University of New Mexico, Albuquerque, NM, United States
- 8Department of Chemical and Biomolecular Engineering, University of Pennsylvania, Philadelphia, PA, United States
Today, the built environment, including infrastructure for tunnels, bridges, highways, subways, railroads, harbors, buildings and airports, is responsible for a significant portion of the energy consumption, natural resource utilization, waste generation as well as CO2 and other environmentally harmful emissions in the United States and around the world. There is no silver bullet solution to achieve the ambitious goal of zero carbon buildings and a city infrastructure with significantly reduced CO2 emissions. Thus, multifaceted solutions should be developed. Another challenge associated with the built environment is aging and a large economic burden to upgrade and maintain the outdated infrastructure. The current status of the U.S. built environment is far below sustainable condition. Rapidly deteriorating infrastructure that must be replaced provides us with the unique opportunity to rethink where and how we should live in the future. In addition, current challenges related to economic and societal inequality in the United States and other global communities also force us to re-evaluate how humanity is connected and how we share resources for a sustainable and healthy future while keeping the Earth safe. The engineering solutions for our future built environment include, but are not limited to, the design and synthesis of new infrastructure materials with low carbon intensity, the development of new manufacturing options and technologies, and the integration of innovative functionalities into building envelopes.
Introduction
Today, the built environment, including infrastructure for tunnels, bridges, highways, subways, railroads, harbors, buildings and airports, is responsible for a significant portion of the energy consumption, natural resource utilization, waste generation as well as CO2 and other environmentally harmful emissions in the United States and around the world. Currently, buildings account for roughly 39% of the energy-related global carbon footprint (Junglaus et al., 2021). Among the most populous countries, the United States has the highest percent of its population living in urban areas at about 82% with 300 cities having populations over 100,000 people (US Census Bureau, 2021). Therefore, it is evident that climate change and CO2 mitigation strategies cannot be discussed without acknowledging contributions from cities and buildings.
In the United States, New York City and New York State for the first time announced a legislation that will limit CO2 emissions from buildings. As part of New York City’s Climate Mobilization Act, Local Law 97 was enacted on 19 May 2019, which will cap annual CO2 emissions from “covered buildings” (i.e., >25,000 square feet) starting in 2024. This cap is designed to decrease over time and will level out in 2050 to an annual average of less than 1.4 kg of CO2e per square foot, whereas on average building emissions in NYC are at approximately 5.4 kg of CO2e per square foot (Govt. of NYC, 2019). Many cities in other parts of the world are also making similar commitments to significantly reduce their carbon footprints. Such a strong commitment towards climate change mitigation is critical and important for our global sustainable future. However, comprehensive solutions and effective strategies to support this ambitious, rapid CO2 reduction plan for cities’ buildings and infrastructure are significantly lacking. As shown in Figure 1, disruptive and transformative technological advancements for the next-generation built environment are essential to achieving CO2 reduction goals, while envisioning a better and more equitable future for both people and our environment.
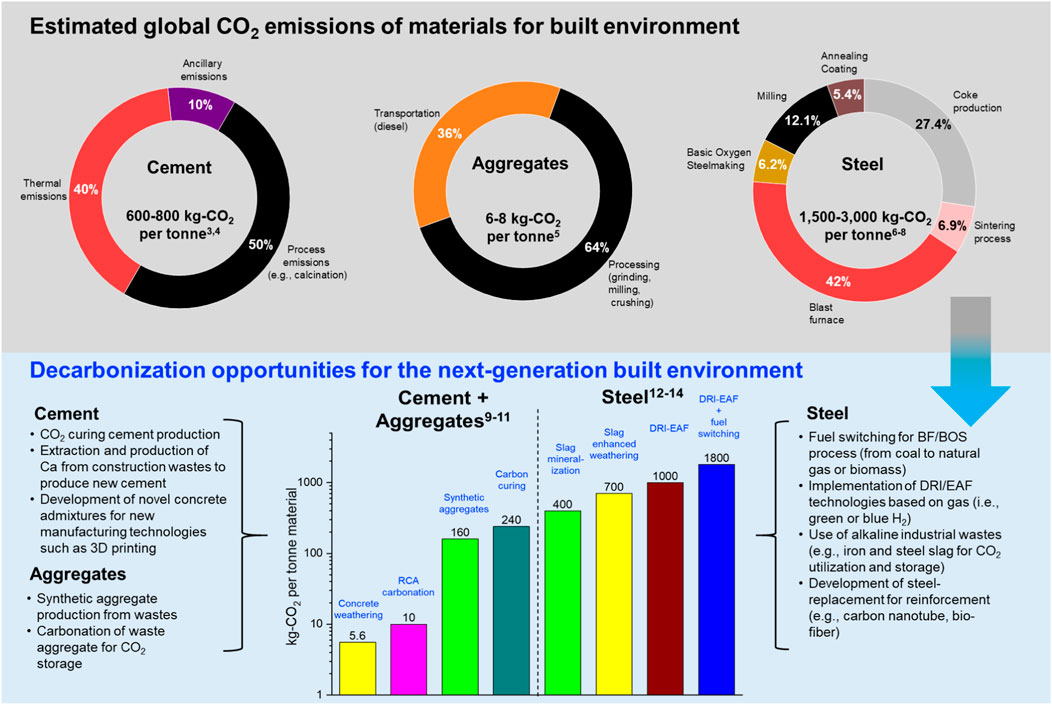
FIGURE 1. Global CO2 emissions associated with cement, aggregates and steel in the built environment and multifaceted opportunities for their decarbonization technologies (Pardo and Moya, 2013; Meddah, 2017; Lehne and Preston, 2018; Mayes et al., 2018; World Steel Association, 2019; Hoffman et al., 2020; Holappa, 2020; Zhang et al., 2020; Gerres et al., 2021; Ho et al., 2021; IEA, 2022; Rosa et al., 2022).
There is no silver bullet solution to achieve the ambitious goal of zero carbon buildings and a city infrastructure with significantly reduced CO2 emissions. Thus, multifaceted solutions should be developed. Another challenge associated with the built environment is aging and a large economic burden to upgrade and maintain the outdated infrastructure (ASCE, 2021). The current status of the U.S. built environment is far below sustainable condition (Figure 2). Rapidly deteriorating infrastructure that must be replaced provides us with the unique opportunity to rethink where and how we should live in the future. Governmental regulation, policies, and new standards that pertain to infrastructure replacement are critical to advancing a low-carbon agenda in the built environment. Some recent progress in this area includes the Infrastructure Investment and Jobs Act (2021) in the U.S., the Resolution on Sustainable and Resilient Infrastructure (UN Environment Assembly, 2019), and the European Union Green Infrastructure Strategy (2019). In addition, current challenges related to economic and societal inequality in the United States and other global communities also force us to re-evaluate how humanity is connected and how we share resources for a sustainable and healthy future while keeping the Earth safe.
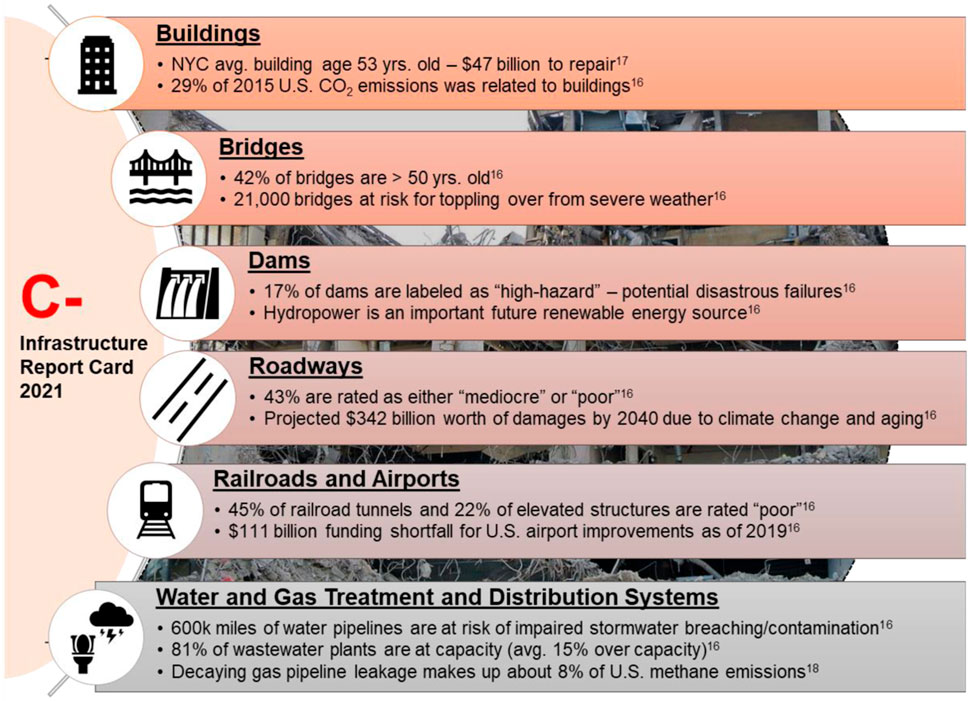
FIGURE 2. Status of aging U.S. infrastructure, which will put an enormous strain on our future sustainable economy and security. (Data sourced from: American Society of Civil Engineers (ASCE), 2021; Statista (c/o Merrill Lynch, CUF), 2014; Blaton et al., 2021).
The engineering solutions for our future built environment include, but are not limited to, the design and synthesis of new infrastructure materials with low carbon intensity, the development of new manufacturing options and technologies, and the integration of innovative functionalities into building envelopes. There are a number of challenges and unique opportunities to consider and explore for maximizing the utilization and storage of carbon emissions in the built environment. Figure 3 provides a vision of the future age of the built environment within the circular economy through the development and deployment of innovative and highly integrated resource recovery, advanced manufacturing and CO2 utilization technologies.
Infrastructure materials with lower carbon intensity
Conventional construction materials such as Ordinary Portland Cement (OPC) have a high carbon intensity (Meddah, 2017; IEA, 2022). When compared to conventionally mined coarse aggregates, such as sand and gravel, that do not require energy-intensive processing. OPC has a much higher footprint of nearly 90x per ton of material. Further, the shortage of resources for construction materials, such as sand aggregates, has already been reported in many regions including developing countries where population growth is significant. Supplementary cementitious materials (SCMs) such as fly ash from coal-fired power plants are already being incorporated into concrete to partially replace cement, reducing the mining of raw minerals while providing multiple environmental benefits including solid waste utilization (Rosa et al., 2022). Given the massive global consumption of concrete, a major requirement of any alternative cement or raw material for clinker is that it is abundantly, consistently, and geographically widely available.
Recently, emerging technologies are being proposed which include the production of Mg-based cement (in contrast to OPC, which is a Ca-based cement). Mg is abundantly available in the Earth’s crust and seawater, and methods to harvest carbon-free raw materials for clinker production could be achieved through electrochemical pathways. Successful efforts have been reported on producing Mg-based cements from wastewater treatment sludge (Qin et al., 2022). Waste concrete aggregates also serve as another area for decarbonization. In contrast to mined coarse aggregates, such as sand and gravel, waste aggregate materials from construction and demolition waste (C&DW) can capture CO2 via carbon mineralization, ranging from 0.087–0.44 tons CO2/ton of aggregate (Sick et al., 2022). Recent projections show that by 2050, upcycled waste aggregates could facilitate the capture of up to 6 Gt CO2/year (optimistic case) (Sick et al., 2022). These carbonated aggregates could then be recirculated into the building supply chain as useful materials or stored for deep CO2 capture.
Furthermore, there is active on-going research to investigate a wide range of other cement replacements. Recently, alkali-activated systems (or geopolymers) have gained popularity, and are entirely composed of clays, fly ash, slag, and other SCMs, thereby completely eliminating the need for cement. Additionally, replacement of cement pastes with precipitated calcium carbonates derived from captured CO2 could also be a viable decarbonization pathway (Williams et al., 2023a; 2023b; Zhao et al., 2023a; 2023b). However, there are issues associated with the early-age properties (i.e., early stiffening and fast setting), which introduces challenges during placement and subsequently limits the use of alkali-activated systems in practice. It is critical that workability, mechanical strength, functionalities, new construction techniques, energy efficiency and overall sustainability from cradle-to-grave all be considered during the development of any next-generation infrastructure material.
CO2 utilization and construction materials
While construction materials have high carbon intensities, they also offer unique opportunities to directly and indirectly reduce CO2 emissions. Over time, the alkaline base (e.g., calcium-bearing phases) within the concrete of buildings and infrastructure naturally reacts with CO2 in air thereby leading to their conversion into solid carbonates (e.g., CaCO3). This reaction, akin to natural silicate weathering, is useful at re-capturing a portion of the carbon which was emitted during the manufacture of the cement (Rosa et al., 2022). There are also engineered pathways by which CO2 may be actively utilized to lower the carbon intensity of construction materials. An example is the use of CO2 for concrete curing. It has been found that curing concrete in a CO2 environment significantly reduces the curing time and temperature while producing stronger concrete (Lehne and Preston, 2018).
Another approach is the direct conversion of CO2 to solid carbonates. These technologies are based on carbon mineralization, which leads to greener construction materials by reacting CO2 with Ca- and Mg-bearing silicate minerals and/or industrial alkaline wastes such as iron and steel slag, red mud, waste-to-energy ashes, mine tailings and waste concrete (Smit et al., 2014; Lehne and Preston, 2018; Mayes et al., 2018; Williams et al., 2024). Since these waste materials are highly heterogeneous and chemically and physically complex compared to conventional raw minerals such as limestone, innovative chemistries and reaction pathways are essential for the effective production of carbon mineralization construction materials with high performance. While the chemical reactions are more complex, there are a number of important benefits of such technologies. Alkaline industrial wastes are generally co-generated with CO2 by the same point source (e.g., iron and steel manufacturing plants and cement plants). Hence, the co-location of CO2 and alkaline solid wastes provides a unique opportunity for effective CO2 utilization while eliminating CO2 transportation. Further, these industrial wastes as well as other unconventional resources including mine tailings often contain other valuable materials such as energy-relevant critical minerals including rare earth elements (REEs). In some cases, heavy metals exist in solid wastes. These valuable or hazardous elements could be recovered during the production of carbonates from alkaline industrial wastes through the use of a pH-swing process. This is commonly performed during two-step leaching and carbonation to raise the pH from the acidic leaching conditions to the basic carbonation conditions; during this pH increase, REEs and heavy metals can be recovered or captured from the reaction liquor (Williams et al., 2024). Thus, the final carbonate products will be free of any REEs or heavy metals. Carbon mineralization to produce carbonates ex-situ also offers opportunities for carbon capture and storage (CCS), whereby excess carbonates could be stored underground in structures such as reclaimed mines (Smit et al., 2014). Therefore, through systematic design of carbon mineralization, the step-wise separation of various high-value components from waste streams is possible further improving the overall sustainability of the developed technologies.
Replacement and decarbonization of steel for structural construction materials
Steel is another important construction material but also with a high carbon footprint (i.e., primary iron and steel production generates 3.5% of global CO2 emissions) (Pardo and Moya, 2013; Hoffman et al., 2020). It has been considered that replacing steel in construction will not be feasible due to its great strength and ductility. However, several novel materials have recently been developed and tested as alternatives. Fiber-Reinforced Polymer and Engineered Cementitious Composites have been suggested as good alternatives to steel reinforcement in concrete. However, the current technology for fabricating these materials is heavily dependent on petroleum resources. Alternatively, bio-fibers, and CO2-based polymer fibers, carbon fibers, and carbon nanotubes provide desired structural advantages and reduced carbon footprint. For example, a recent study demonstrated that carbon nanotubes can be synthesized from seaweeds contaminated with plastic, effectively upcycling the waste materials in a low-carbon manner (Williams et al., 2023c). In addition to the reduction in CO2 emissions, bio-fibers and CO2-derived materials can provide improved performance in terms of corrosion resistivity. The development of these new materials will also have a great impact on the design of future buildings and construction techniques. Unfortunately, the strength and versatility of steel makes it hard to completely replace. As shown previously in Figure 1, there are a multitude of process alternatives which could be used to assist in steel decarbonization. These include fuel switching from coke and coal to gas and biomass, utilizing electric arc furnaces coupled, and the direct reduction of iron with renewable hydrogen (Mayes et al., 2018; Holappa, 2020; Gerres et al., 2021). Ex-situ carbon mineralization to capture CO2 through the carbonation or enhanced weathering of steel slags, could also be a promising method for steel decarbonization and upcycling of wastes in a tandem process (Smit et al., 2014; Mayes et al., 2018; Holappa, 2020).
Functionalized buildings and infrastructure
Multi-functional materials include solar cell roofing materials and windows for on-site energy harvesting, built-in energy storage materials and systems, phase-changing materials for improved heat modulation, and pollution-capturing building materials for indoor air quality control are just a few of the examples of how building materials can serve as more than just physical structure. Functional materials might also include sensing and computational capabilities, and thus, enable smart infrastructure maintenance. These added functionalities will allow buildings and infrastructure to be actively integrated into our energy systems, to have improved longevity and sustainability, and will provide intra and inter-connectivity to buildings and cities.
The functionalization of building materials can also impact human and environmental health. It has been reported that low indoor humidity is closely linked to increased influenza virus transmission in the wintertime (e.g., COVID-19). The high concentration of volatile organic compounds (VOCs) and fine particulates in indoor air have been suggested as one of the causes of the rapid increase in lung cancer occurrences in non-smoking populations. Thus, there are on-going efforts to develop unique air cleaning systems such as smart nanostructured materials that have strong adhesion capabilities to capture fine particles in air, and filter materials that can simultaneously capture CO2 and VOCs while controlling humidity. These materials may be directly integrated into buildings and related infrastructure. The design and development of these innovative functionalized materials should be one of the key areas of R&D for achieving a sustainable and healthy built environment.
Buildings and infrastructure as an interactive interface between humans and the environment
Once constructed, the built environment continues to consume significant energy throughout its lifetime to accommodate occupants and their activities. The integration of environmental sensors, data collection and analysis for feature extraction, and demand response control should be key factors to improving a building’s sustainability. This requires modeling of the building envelope, together with a deep understanding of demand response signals and control. Sensors can measure the indoor air quality and external environmental conditions, with the data integrated to inform how we can better use the buildings, all while monitoring our environment and public health. Novel sensor designs include paintable sensors and wallpaper sensors, as well as sensors directly integrated into building materials. Self-reporting materials that can be non-destructively and rapidly tested to assess performance and subsequent interventions would also be important for future building design. These interactive interfaces among the built environment, humans and the environment will work together to create a unique digital built environment to effectively connect them and improve the efficiency and quality of buildings.
Additive and lean manufacturing technologies for the built environment
The development of new construction materials, functional materials, sensors and control systems, combined with their incorporation into buildings now calls for new ways of manufacturing and construction. Additive and lean manufacturing technologies will play important roles in creating our future buildings and infrastructure by allowing a holistic design of structures while minimizing raw material requirements and related waste. The interplay between new materials and construction techniques should be carefully investigated to achieve synergistic improvement in the overall energy efficiency and performance of our built environment.
For the first time in decades, we might be able to reimagine our construction methods and materials all together. We could be able to use advanced 3-D printing methods to print and place reinforcements and concrete together in a layered approach, or even completely alter the construction “ink” and abandon concrete and steel as we know them and use novel structural enhancers, such as carbon nanotubes. This new construction ink might also incorporate smart components that allow for sensing damage at an early stage, and self-healing properties that provide a level of longevity and sustainability that do not exist today. The opportunity before us is in the creation of a new construction paradigm in a carbon-constrained world. In addition, a number of innovative robotic systems are also being developed to minimize the use of raw materials in buildings and to allow the construction of buildings and infrastructure in extreme environments including underwater and on other planets. Virtual reality is used in architecture, engineering and construction and new manufacturing technologies continue to help fill economic productivity and efficiency gaps.
Circular life cycle of buildings and infrastructure
The CO2 emissions of buildings and infrastructure do not start with the completion of their construction and first use and occupancy. Rather, it starts with the mining of raw materials and generally ends with the disposal of demolished structures. As previously discussed, there are emerging efforts in producing construction materials from waste concrete. This is a great step towards the materials circularity of construction materials, but it may also be accompanied by better demolition technologies. The current practice of building demolition is not designed to produce well-separated waste streams that can allow effective recovery of materials. Thus, additive and lean manufacturing approaches should be employed not only in the construction and its use and operation phases, but also in the demolition and dismantling steps (e.g., prefabricated elements allowing effective separations). It is also important that these technological developments be assessed to accurately evaluate the cradle-to-grave environmental impacts of buildings and related infrastructure. Unfortunately, life cycle analyses can only be as good as the available data. Data science including effective data collection should be further utilized in environmental impact studies to provide improved guidelines for construction and end-of-life issues of our built environment. Discussions on climate relevant timescales and the permanency of embodied carbon are also essential to addressing the fate of CO2 utilized in a built environment at the end of a building’s lifetime.
Certification, regulation, and future policies for sustainable buildings and infrastructure
The construction industries are very conservative and recalcitrant to change. This is understandable considering the importance of buildings and infrastructure related to safety. Unfortunately, we have seen too many times how a failure of infrastructure, such as large dams, which can destroy human lives and the environment. Thus, the certification of new materials and construction techniques should be carried out with the highest rigorousness, while decarbonizing the built environment. However, the standardization process of inclusion of novel materials into conventional built environment building-blocks themselves prevents an additional barrier. Standards change slowly, and government regulators are often weary and slow to approve the use of alternatives (e.g., the use of novel fillers, such as carbonates, in cement at high replacement levels). Appropriate regulations and policies play critical roles in the development of environmental technologies and systems. The low-carbon fuel standard mandate enacted in California in 2007, the 45Q tax credit announced in 2018, and the passage of the Infrastructure Investment and Jobs Act (2021) and the Inflation Reduction Act (2022) as well as Energy Earthshots, expanding 45Q access in new CCUS activities, are good examples of environmental policies within the U.S. that accelerate sustainable development of new technologies. Additional global examples include the European Union (EU) Green Infrastructure Strategy (2019), the EU Climate Law (2021), and the UN Resolution on Sustainable and Resilient Infrastructure (2019). Active and effective communications among all stakeholders including academic researchers, industries and government as well as the public will be important for the development of key regulations and policies needed for our sustainable built environment and cities.
Future societal and economic impacts in regional and global communities
As renewable energy continues to grow in our energy mix, it will allow us to develop improved ways to utilize our resources while minimizing carbon intensity not only in the power sector but also in all other industries including construction. Different regions around the world would undergo distinct stages of their economic developments for the next decades, and thus, it would impact their efforts towards sustainable built environment. In addition to climate change, regional and global communities are experiencing other strong changes such as resource scarcity, re-urbanization, demographic and social changes, and increased inequality in the global and local distribution of resources. All these factors will influence where and how we should live in the future. The next-generation built environment for the subsequent 100 years and beyond should be designed and developed carefully considering healthy humanity, global connectivity, technological advances and artificial intelligence, and environmental sustainability, while effectively addressing our changing climate.
Summary and outlook
Increased building efficiency remains an important goal for the built environment and both scholarship and industries have put this into policy and practice. What is increasingly clear is that the built environment provides several major new opportunities for carbon reduction: novel materials with significantly lower life-cycle footprints, CO2 emissions reduction, and opportunities to take up and bind carbon indefinitely. Recent technological advancements now allow the substitution of biological or atmospheric carbon for fossil organic or mineral carbon, leading to direct CO2 removal and storage in the built environment. These new technologies could transform the carbon-emitting industry into the carbon-storing industry as illustrated in this article. To achieve this promise, these technologies must mature, including the growth of supply chains, manufacturing bases, and incorporation into building and construction standards. Acceptance by practitioners, engineers, architects, and artisans of the built environment is essential, as is the commercial and economic viability and codification through policy. This work in its entirety will define the environment where humans live and work for decades and possibly centuries to come. The case for innovation has rarely been so strong.
Data availability statement
The original contributions presented in the study are included in the article/supplementary material, further inquiries can be directed to the corresponding author.
Author contributions
A-HP: Conceptualization, Funding acquisition, Project administration, Resources, Writing–original draft, Writing–review and editing. JW: Data curation, Writing–original draft, Writing–review and editing. JF: Writing–original draft, Writing–review and editing. DH: Writing–original draft, Writing–review and editing. SK: Writing–original draft, Writing–review and editing. VS: Writing–original draft, Writing–review and editing. MT: Writing–original draft, Writing–review and editing. JW: Writing–original draft, Writing–review and editing.
Funding
The author(s) declare that financial support was received for the research, authorship, and/or publication of this article. This publication is supported by Lenfest Center for Sustainable Energy at Columbia University, Global CO2 Initiative at University of Michigan, NSF AccelNet SCO2RE (CBET 1927336), NSF AccelNet 3DConcrete (Award #2020095), NYSERDA (Agreement#: 0000185059), and Korea Institute of Energy Technology Evaluation and Planning (KETEP) grant funded by the Korea government (MOTIE) (No. 2018855000).
Conflict of interest
The authors declare that the research was conducted in the absence of any commercial or financial relationships that could be construed as a potential conflict of interest.
The author(s) declared that they were an editorial board member of Frontiers, at the time of submission. This had no impact on the peer review process and the final decision.
Publisher’s note
All claims expressed in this article are solely those of the authors and do not necessarily represent those of their affiliated organizations, or those of the publisher, the editors and the reviewers. Any product that may be evaluated in this article, or claim that may be made by its manufacturer, is not guaranteed or endorsed by the publisher.
References
American Society of Civil Engineers. (2021). A comprehensive assessment of America’s infrastructure. Available at: https://infrastructurereportcard.org/ (Accessed October 11, 2022).
Blanton, E. M., Lott, M., and Smith, K. (2021). Investing in the U.S. natural gas pipeline system to support net-zero targets. NewYork, NY, USA: Columbia University.
Gerres, T., Lehne, J., Mete, G., Chenk, S., and Swalec, C. (2021). Green steel production: how G7 countries can help change the global landscape. LeadIt 6.
Govt. of Nyc. (2019). Local laws of the city of New York for the year 2019. https://www1.nyc.gov/assets/buildings/local_laws/ll97of2019.pdf (Accessed March 15, 2022).
Ho, H. J., Iizuka, A., Shibata, E., Tomita, H., Takano, K., and Endo, T. (2021). Utilization of CO2 in direct aqueous carbonation of concrete fines generated from aggregate recycling: influences of the solid–liquid ratio and CO2 concentration. J. Clean. Prod. 312, 127832. doi:10.1016/J.JCLEPRO.2021.127832
Hoffman, C., Van Hoey, M., and Zeumer, B. (2020). Decarbonization in steel. McKinsey and Co. New York, NY, USA, Available at: https://www.mckinsey.com/industries/metals-and-mining/our-insights/decarbonization-challenge-for-steel (Accessed July 10, 2022).
Holappa, L. (2020). A general vision for reduction of energy consumption and CO2 emissions from the steel industry. Metals 10 (9), 1117. doi:10.3390/MET10091117
International Energy Agency. (2022). Cement. Available at: https://www.iea.org/reports/cement (Accessed June 15, 2023).
Jungclaus, M., Esau, R., Olgyay, V., and Rempher, A. (2021). Reducing embodied carbon in buildings: low-cost, high-value opportunities. Rocky Mountain Institute. Colorado, CO, USA, Available at: http://www.rmi.org/insight/reducing-embodied-carbon-in-buildings.
Lehne, J., and Preston, F. (2018). Making concrete change innovation in low-carbon cement and concrete. Chatham House Report. Chathamhouse, London, UK, Available at: https://www.chathamhouse.org/2018/06/making-concrete-change-innovation-low-carbon-cement-and-concrete (Accessed July 5, 2021).
Mayes, W. M., Riley, A. L., Gomes, H. I., Brabham, P., Hamlyn, J., Pullin, H., et al. (2018). Atmospheric CO2 sequestration in iron and steel slag: consett, county durham, United Kingdom. Environ. Sci. Technol. 52 (14), 7892–7900. doi:10.1021/acs.est.8b01883
Meddah, M. S. (2017). Recycled aggregates in concrete production: engineering properties and environmental impact. MATEC Web Conf. 101, 1–8. doi:10.1051/matecconf/201710105021
Pardo, J. A. N., and Moya, K. V. (2013). Prospective scenarios on energy efficiency and CO2 emissions in the EU iron and steel industry. Available at: https://s1hcifs01/DEMProfiles/19230/Downloads/ld1a25543enn.pdf,
Qin, J., Zhu, H., He, Z., Yu, X., Shi, J., Lu, J., et al. (2022). Recycling of water treatment sludge into magnesium potassium phosphate cement component by a combination of silica fume. J. Build. Eng. 51, 104308. doi:10.1016/J.JOBE.2022.104308
Rosa, L., Becattini, V., Gabrielli, P., Andreotti, A., and Mazzotti, M. (2022). Carbon dioxide mineralization in recycled concrete aggregates can contribute immediately to carbon-neutrality. Resour. Conserv. Recycl. 184, 106436. doi:10.1016/J.RESCONREC.2022.106436
Sick, V., Stokes, G., and Mason, F. C. (2022). CO2 utilization and market size projection for CO2-treated construction materials. Front. Clim. 4, 96. doi:10.3389/fclim.2022.878756
Smit, B., Park, A. H. A., and Gadikota, G. (2014). The grand challenges in carbon capture, utilization, and storage. Front. Energy Res. 2, 55. doi:10.3389/fenrg.2014.00055
Statista (2014). Average age of New York city infrastructure as of 2014. NewYork, NY, USA: Merrill Lynch, CUF.
U.S. Census Bureau. (2021). City and town population totals: 2010-2019, incorporated places of 50,000 or more. Available at: https://www.census.gov/data/tables/time-series/demo/popest/2020s-total-cities-and-towns.html (Accessed July 5, 2022).
Williams, J. M., Nitzsche, M. P., Bromberg, L., Qu, Z., Moment, A. J., Hatton, T. A., et al. (2023c). Hybrid thermo-electrochemical conversion of plastic wastes commingled with marine biomass to value-added products using renewable energy. Energy Environ. Sci. 16, 5805–5821. doi:10.1039/d3ee02461j
Williams, J. M., Zhao, D., Moon, S., Kawashima, S., Park, A. H. A., and Moment, A. J. (2023b). Stabilization of pure vaterite during carbon mineralization: defining critical activities, additive concentrations, and gas flow conditions for carbon utilization. Cryst. Growth Des. 23, 8103–8115. doi:10.1021/acs.cgd.3c00835
Williams, J. M., Zhao, D., Zhang, N., Chin, A., Kawashima, S., and Moment, A. J. (2023a). Directed synthesis of aragonite through semi-continuous seeded crystallization methods for CO2 utilization. CrystEngComm 25 (43), 6050–6066. doi:10.1039/d3ce00809f
Williams, J. M., Zhao, D., Zhang, N., Zheng, A., Greenbaum, S. G., Kawashima, S., et al. (2024). Calcium carbonate and reactive silica recovery from waste cement: the influence of processing parameters on upcycled material properties and carbon intensity. Chem. Eng. J. 482, 149013. doi:10.1016/J.CEJ.2024.149013
World Steel Association. (2019). World steel in figures 2019; world steel association: Brussels, Belgium. Available at: https://worldsteel.org/wp-content/uploads/2019-World-Steel-in-Figures.pdf (Accessed August 5, 2021).
Zhang, N., Duan, H., Miller, T. R., Tam, V. W. Y., Liu, G., and Zuo, J. (2020). Mitigation of carbon dioxide by accelerated sequestration in concrete debris. Renew. Sustain. Energy Rev. 117, 109495. doi:10.1016/J.RSER.2019.109495
Zhao, D., Williams, J. M., Li, Z., Park, A. H. A., Radlińska, A., Hou, P., et al. (2023a). Hydration of cement pastes with calcium carbonate polymorphs. Cem. Concr. Res. 173, 107270. doi:10.1016/j.cemconres.2023.107270
Keywords: carbon dioxide, infrastructure, climate change, construction materials, circular economy, functionalized building
Citation: Park A-HA, Williams JM, Friedmann J, Hanson D, Kawashima S, Sick V, Taha MR and Wilcox J (2024) Challenges and opportunities for the built environment in a carbon-constrained world for the next 100 years and beyond. Front. Energy Res. 12:1388516. doi: 10.3389/fenrg.2024.1388516
Received: 19 February 2024; Accepted: 07 March 2024;
Published: 18 March 2024.
Edited by:
Youngjune Park, Gwangju Institute of Science and Technology, Republic of KoreaReviewed by:
Yunseok Lee, Georgia Institute of Technology, United StatesSeokyoon Moon, Seoul National University of Science and Technology, Republic of Korea
Copyright © 2024 Park, Williams, Friedmann, Hanson, Kawashima, Sick, Taha and Wilcox. This is an open-access article distributed under the terms of the Creative Commons Attribution License (CC BY). The use, distribution or reproduction in other forums is permitted, provided the original author(s) and the copyright owner(s) are credited and that the original publication in this journal is cited, in accordance with accepted academic practice. No use, distribution or reproduction is permitted which does not comply with these terms.
*Correspondence: Ah-Hyung Alissa Park, YXBhcmtAc2Vhcy51Y2xhLmVkdQ==