- Department of Civil Engineering, Faculty of Engineering and the Built Environment, Tshwane University of Technology, Pretoria, South Africa
The tangible effects of climate change and the influence of environmental factors on climate have driven a shift towards cleaner and more sustainable energy sources. This study investigates the effects of biochar and zeolites in the anaerobic digestion (AD) and co-digestion processes of cassava wastewater (CW) with livestock manure, aiming to assess their impact on methane (CH4) and carbon dioxide (CO2) production, as well as process stability. The study employs design of experiments, analysis of variances, design parameter sensitivity, and differential analysis to explore how varying concentrations of biochar and zeolites influence key production parameters and optimize the overall process. Results indicate that increasing biochar and zeolite concentrations produce alternating effects on CO2 and CH4 generation, with CW significantly contributing to CH4 production. Livestock manure shows similar effects on both gases, necessitating calculated trade-offs in prioritizing one over the other. Findings provide insights into enhancing biogas production and waste management, contributing to sustainable bioenergy and wastewater treatment practices. Numerical results demonstrate that increased biochar concentrations in the co-digestion mixtures increased CO2 and CH4 production rates respectively by an approximate factor of 2, contributing 18% and 5% overall effects respectively. Similarly, zeolite concentrations contributed 9% and 5% overall effects to the production rates of CO2 and CH4 respectively, boosting production rates by factors of 1 and −2. The study’s significance lies in its potential to improve biogas yield and process efficiency, fostering advancements in renewable energy and environmental management.
1 Introduction
The demand for effective waste management, environmental pollution prevention, and the need for transition to sustainable green energy is steadily rising globally. This demand is driven by the changing climate, and expanding population resulting in a continuous and increasing flow of organic waste generated daily across diverse sectors of human activities—agriculture, municipalities, industries, and households. Anaerobic digestion technology—an age-long, low-cost technology—has been profitably applied to simultaneously reduce organic waste and produce renewable energy. Sevillano et al. (2021), highlighted several studies reporting the many benefits of anaerobic digestion in terms of energy and by-products from bio refineries. Anaerobic Digestion (AD) technology utilizes biological processes to break down organic matter without oxygen, facilitated by microorganisms (Batstone et al., 2002; Gujer and Zehnder, 1983; Gunnerson and Stuckey, 1986). The AD process usually occurs in an oxygen-free environment and involves the sequential activity of different microbial groups. The primary outcome of anaerobic digestion is the production of biogas, a mixture of methane and carbon dioxide. The AD process typically involves four critical stages; Hydrolysis (Complex organic materials are broken down into simpler compounds by enzymes); Acidogenesis (Organic acids and simpler compounds are further broken down into volatile fatty acids, alcohols, and other intermediate products); Acetogenesis (Volatile fatty acids are converted into acetic acid, hydrogen, and carbon dioxide) and Methanogenesis (Methanogenic microorganisms convert acetic acid, hydrogen, and carbon dioxide into methane and carbon dioxide) (Meegoda et al., 2018; Tang S. et al., 2023). Bioenergy production and recovery from organic wastes through AD processes offer a low-cost sustainable strategy for valorising various kinds of waste. While the general concept and science of AD has long been established in literature, there are still several ongoing studies focusing on improving different specific aspects of AD processes and exploring the biodegradability of different kinds of biomasses and feedstocks, such as food wastes (Paranjpe et al., 2023; Pilarska et al., 2023; Xu et al., 2018), lignocellulosic agricultural wastes (Karrabi et al., 2023; Lallement et al., 2023; Yan et al., 2017), animal manure (Silwadi et al., 2023; Song et al., 2023); and wastewater sludge (Di Capua et al., 2020; Nguyen et al., 2021). Various strategies have been applied to improve the overall AD process efficiency, yield and quality of bioenergy production from organic waste. Strategies such as; Pre-treatment of feedstock (Paudel et al., 2017; Soltanian et al., 2020; Zheng et al., 2014), mixing and homogeneity of substrate (Lindmark et al., 2014), optimal temperature (Buffière et al., 2018), inoculum to substrate ratio (Rajput and Sheikh, 2019), and by studying various microbial interactions (Tabatabaei et al., 2020) and synergy to improve the overall AD processes.
More recently, co-digestion and use of additives and nanoparticles (Achi et al., 2020; Hassanein et al., 2019; Jadhav et al., 2021; Kumar S. S. et al., 2021; Wang et al., 2023) have been applied to reduce the effects of inhibitors and enhance microbial activities, by providing an enabling environment for microbial interactions through the four stages of AD. Anaerobic co-digestion (AcoD), which refers to the simultaneous digestion of multiple organic substrates, has gained attention due to its potential to enhance biogas production and waste management efficiency (Karki et al., 2021; Khan et al., 2023; Xie et al., 2018). The (AcoD) process involves combining different organic materials, such as sewage, slurry, animal manure, lignocellulosic biomass and food wastes, to optimize methane yield and improve the overall performance of anaerobic digestion. Co-digestion has been found to address the low nutrient levels in certain wastes, which are insufficient for standalone anaerobic digestion, making it a viable option for improving methane yield (Abbas et al., 2023; Chow et al., 2020; Siddique and Wahid, 2018). Mathematical models have been developed to optimize anaerobic co-digestion processes, focusing on parameters such as co-substrate/inoculum ratio, C/N ratio, and temperature to enhance methane production and volatile solids reduction (Ghaleb et al., 2020). Studies have demonstrated the benefits of co-digestion with various substrates, including cheese whey, slaughterhouse waste, and microalgae, in improving biogas yield and biodegradation efficiency (Ferdeș et al., 2023; González et al., 2022; Kunatsa and Xia, 2022; Rincón et al., 2018). Furthermore, the addition of high-strength waste materials and wastewater, such as industrial wastewater, landfill leachate, cassava wastewater, waste activated sludge and food waste, has been explored for economic feasibility and enhanced biogas production. Co-digestion has also been shown to improve buffering capacity and methane production, particularly when combining substrates with low pH. Additionally, the co-digestion of carbon-rich substrate with nitrogen-rich substrate has been found to balance the C/N ratio of the substrate, benefiting the anaerobic digestion process (González et al., 2022; Wang et al., 2024). Beyond the potential economic benefits derived from high-strength wastes, there is an urgent need to prevent organic pollution and eutrophication resulting from poor management of high-strength industrial wastewaters in many developing countries (Achi et al., 2020).
Cassava wastewater (CW) is a typical example of a high-strength wastewater, with high concentrations of chemical oxygen demand (COD), biochemical oxygen demand (BOD), and total solids (TS), as well as a low pH (Peres et al., 2019; Zurita and Vymazal, 2023). Stabilization ponds, aerobic systems, and anaerobic digestion (AD) systems, have been utilized for the management of CW in certain regions, including Thailand, Brazil, Vietnam, India, and Nigeria (Kumar S. et al., 2021). Cassava is globally recognized for its paramount significance as the most extensively cultivated root crop in tropical regions. It consistently plays a crucial role in ensuring food security and holds the sixth position among the world’s most important crops (Otekunrin and Sawicka, 2019). Additionally, cassava serves as a primary staple for more than 500 million people in Africa. Renowned for its resilience to drought and adaptability to challenging environments, cassava emerges as a vital strategy for climate change adaptation (Mupakati and Tanyanyiwa, 2017). Anaerobic digestion is currently being explored as a sustainable technology that can be deployed towards managing the huge streams of untreated wastes from cassava production. The anaerobic digestion of CW has raised concerns due to its low nitrogen concentration and rapid acidification (low pH) observed during AD process (Palma et al., 2018). Co-digestion with a nitrogen-rich substrate, such as animal manure, could decrease the carbon-to-nitrogen (C:N) ratio and provide buffering capacity for stabilizing the pH in order to increase methane (CH4) production.
In general, the use of additives and nanoparticles to enhance synergistic microbial interactions between different microbial species, through direct interspecies electron transfer (DIET), has received tremendous attention. Additives such as biochars, hydrochars, zeolites, trace metals, modified zeolites and biochars, conductive materials and nanoparticles (NPs), have contributed significantly in increased methane production by promoting microbial growth through electron transfer, preventing the accumulation of toxic concentrations of ammonia, encouraging the formation of biofilm/anaerobic granular sludge, providing nutrient supplements, removing H2S, sequestering CO2, and incorporating bio-augmentation (Arif et al., 2018; Hassanein et al., 2019). The application of additives and conductive materials in AD of cassava wastewater has not been sufficiently explored. A major research gap in the application of additives and conductive materials in AD of cassava wastewater is the lack of comprehensive studies on the stability and efficiency of these materials under varying operational conditions. Addressing this gap is crucial for optimizing the practical and sustainable application of AD technologies in real-world settings. To achieve sustainability in the management and utilization of cassava wastes and wastewater, through AD processes, there is a need to improve cassava wastewater AD system performance and predict specific outcomes in bioenergy and production, including the development of guidelines and parameters that will aid in scaling up from pilot to large scale cassava AD systems to meet local energy needs in many developing countries.
This research will build on existing literature that demonstrates the potential of biochar in optimizing anaerobic digestion (Fagbohungbe et al., 2017; Osman et al., 2023; Wei et al., 2020). Previous studies have shown that biochar can catalyze anaerobic digestion, mitigate ammonia inhibition, and enhance methane production from various organic wastes, including primary sludge and food wastes (Cai et al., 2016; Wei et al., 2020). Additionally, biochar has been found to improve microbial activity in anaerobic digestion systems and enhance nutrient availability for plant growth (Deenik and Cooney, 2016). These findings provide a strong rationale for investigating the role of biochar in the co-digestion of cassava wastewater with livestock manure. Furthermore, the study will explore the potential of zeolites in influencing the microbial and enzymatic transformations during anaerobic digestion. Zeolites have been reported to improve anaerobic digestion by influencing microbial activities, adsorbing ammonia, and supporting methanogenic activity (Montalvo et al., 2012; Paritosh et al., 2020; Tang C. C. et al., 2023). These references provide a basis for investigating the impact of zeolites in the co-digestion process and their potential to enhance biogas production from cassava wastewater and livestock manure. The experimental design involves batch experiments to assess the performance of biochar and zeolite in anaerobic digestion and co-digestion systems.
This study contributes to the understanding of the synergistic effect of biochars and zeolites on anaerobic digestion and co-digestion processes involved biogas production and methane yield, particularly within the context of additives such as cassava wastewater and livestock manure, [Dairy manure (DM) and Poultry Litters (PL)]. By synthesizing the findings from previous studies, and also applying parameter sensitivity analysis, the research will provide valuable insights into the application of biochars and zeolites for sustainable biogas production and waste management.
2 Materials and methods
2.1 Preparation of cassava wastewater and livestock manure substrates
Cassava tubers were acquired from a local market, manually peeled, and soaked for 5 days in the laboratory with 1 L/kg of deionized water, replicating the traditional cassava processing steps for “fufu” production—an African dish derived from fermented cassava paste. After manual squeezing, the resulting cassava wastewater (CW) was collected for the experiments. Key characteristics of the substrate and experimental design are detailed in Tables 1, 2. The CW exhibited COD levels ranging from 29.8 to 33.4 g/L, volatile solids (VS) of 17.3 g/kg, total solids (TS) of 17.8 g/kg, and a pH of 5.5.
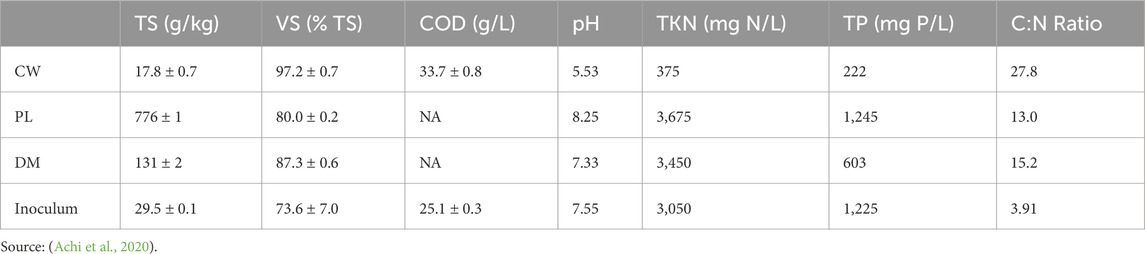
Table 1. Characterization of substrates: poultry litter (PL), dairy manure (DM), cassava wastewater (CW), and the inoculum source [The parameters include total solids (TS), volatile solids (VS), chemical oxygen demand (COD), pH, total Kjeldahl nitrogen (TKN), total phosphorus (TP), and the carbon-to-nitrogen ratio (C:N)].
The characteristics of the CW substrate used in this experiment is limited by the processing conditions, considering that the fermentation was done under a controlled laboratory environment which only tried to replicate the traditional process.
The co-substrate dairy manure (DM) and Poultry Litter (PL) was collected from a livestock farm. The Poultry litter (PL) sourced from a poultry broiler farm consisted of poultry droppings and beddings from wood shavings. Both manure substrates were collected onsite and stored at 4°C until utilized. The inoculum for the experiment was derived from the digestate of a complete mixed wastewater sludge digester and was stored at 4°C before application.
2.2 Selection of biochars and zeolites
The co-treatments of biochar (B-Char) and clinoptilolite zeolite (ZEO) were introduced into the cassava wastewater (CW). The biochar (B-Char) was derived from corn stover through pyrolysis under an oxygen-free atmosphere at 500°C for 10 min (ArtiCHAR, Prairie City, Iowa, United States). The biochar particles ranged from 841 mm to <74 mm, possessing volatile solids (VS) and total solids (TS) of 690 and 980 g/kg, respectively. The zeolite used was high-purity 97% clinoptilolite zeolite from Amargosa Valley (Nye county, NV, United States), presenting as granules with an angular shape and gray color, and having a pore diameter between 4.0 and 7.0 angstroms.
2.3 Experimental design
Following the methods outlined by Moody et al. (2011), a batch digestion experiment, employing the biochemical methane potential (BMP) test, was done at the Water Quality Laboratory of the University of Maryland’s Department of Environmental Science and Technology (ENST) in College Park, MD, United States. Before commencing the BMP tests, the total solids (TS) and volatile solids (VS) for CW, PL, DM, and the inoculum was determined and used to establish co-substrate ratios based on VS.
Before incubation, each reactor’s headspace underwent a 3-min purge with N2 to establish anaerobic conditions. The reactors were promptly sealed with a rubber septum and positioned on a shaker (120 rpm) within a controlled environmental chamber at 35°C for 48 days. Daily biogas volume was measured through volumetric displacement, employing a graduated, gas-tight 50 mL glass syringe inserted through the rubber septum. Biogas production was quantified volumetrically under normal temperature and pressure conditions using a glass gas-tight syringe, calibrated to atmospheric pressure. All methane (CH4) production values are reported under standard temperature and pressure conditions (1 atm and 20°C) (Achi et al., 2020).
2.4 Data generation
The parametric analysis of the effects of the inputs of the biogas production was guided by design of experiments based on a random combination of its input parameters. The use of design of experiments is hinged on its convenience and effectiveness in determining the individual or interactive effects of influential factors in a data set as well as in finding out the best operating fits of sets of data into a fitness function. Consequently, quantities of the five main ingredients of the biogas production process and a sixth quantity, Time, were computed as input factors of the process while the outputs of the process were the quantities of Carbon Dioxide (CO2) and Bio-Methane (CH4) generated. The resulting matrix was a Design of Experiment (DOE) matrix of 6 design variables factors of 3 – 5 levels to each combine randomly to produce multiple datasets of 2 responses as shown in Table 2.
For the avoidance of skewness in the sampling of the data space, with the hindsight of the broader objectives which require determining the design variable(s) that affect and possibly optimize the yield of corresponding response, a test of coverage was carried out on the experimental design parameters using the optimal latin hypecube sampling technique which tests and allows multiple sampling of data points, and through which more combinations can be studied for each factor. Hence, providing the comparative advantage of allowing a flexible selection in the number of designs greater than the number of factors to run. The result is a quadrant of evenly spread experiment points within the n-dimensional space defined by the n number of factors as shown in the scatter plot in Figure 1.
The scheme of highly randomized experimental parameter combinations generated from the sampling process is shown in Table 3, consequent upon which the results of the experiment conducted with each run of combinations are documented in the response columns for further computation and processing.
2.5 Data collection and analysis
The Concentrations of total solids (TS) and volatile solids (VS) for all samples were determined following the standard methods for the examination of water and wastewater (Rice et al., 2012) – TS using Method 2540B and VS using Method 2540E. The pH of substrates and inoculum was measured using an Accumet AB 15 pH meter (Fisher Scientific, Hampton, NH). Total Kjeldahl nitrogen (TKN) and total phosphorus (TP) samples were analyzed utilizing a Lachat autoanalyzer (Quikchem 8500, Hach Company, Loveland, CO, United States) with QuikChem methods 13-107-06-2-D for TKN and 13115-01-1-B for TP. The concentration of chemical oxygen demand (COD) was measured using a Hach DR 5000 spectrophotometer (Hach Company, Loveland, CO, United States).
Biogas content analysis for methane (CH4) and carbon dioxide (CO2) was conducted by injecting a 0.10 mL gas sample through a luer-lock, gas-tight syringe into an Agilent HP 7890 A gas chromatograph (Agilent Technologies, Santa Clara, CA, United States). The gas chromatograph was equipped with a thermal conductivity detector (TCD) and a single HP porous layer open tubular (PLOT) Q column, with an injection temperature of 250°C, a detector temperature of 250°C, an oven temperature of 60°C, and conveyed using helium gas at a flow rate of 8.6 mL/min (Hassanein et al., 2019). The carbon content of the CW, PL, DM, and inoculum were calculated using the equation (Adams et al., 1951), where % Carbon = % VS/1.8.
Where
R = C/N ratio; Qn = mass of material n (“as is”, or “wet weight”); Cn = carbon (%); Nn = nitrogen (%); Mn = moisture content (%) of material n.
3 Results and discussions
3.1 Response model
In order to adequately approximate the effect of each input parameter of the biogas production process on the outcomes, a numerical approach was deployed (Figure 2), to help in reducing the volume, cost and time incurable from the repetitive experimental evaluation that is characteristic of such a parametric study. Thus, linear regression methods were applied to the response data set of the experimental design to generate a response surface distribution fit into a surrogate empirical model representative and predictive of the experimental biogas production process. The corresponding empirical surrogate for the two biogas products under consideration, CO2 and CH4 are presented in Equations 1, 2 as;
Where
The R2 values, obtained from the R-squared error analyses method is given by the expression.
Where N,
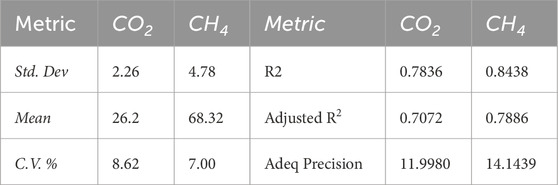
Table 4. Fit statistics of the response surrogate models as computed from the analyses of variance (ANOVA).
Figure 3A presents the plots of the predicted and actual experiment data corresponding to each response. The close proximity of the data points corresponds to the results predicted by the empirical surrogates and the actual experiment to the diagonal line. The diagonal represents a perfect correlation where predicted values equal the actual values. The generality of the points falls on or close enough to the diagonal line indicating model reliability and lending credence to its validity. Figure 3B presents the distribution of residual values on normal plots in the form of studentized residuals, which is the ratio of the residuals to the estimated standard deviation of each residual. The normal distribution of the points of the plot which appreciably align with the diagonal demonstrates the adequacy of the fitness models as approximators of the experimental outcomes. Figure 3C presents the plot of the Cook’s distance which describes point(s) within the design space that may be over or under-predicted, with large residuals capable of distorting the results of the model’s prediction. Such points are outliers of the dataset often required to be checked and recomputed. As illustrated in the figure, all points are below the Cooks distance index
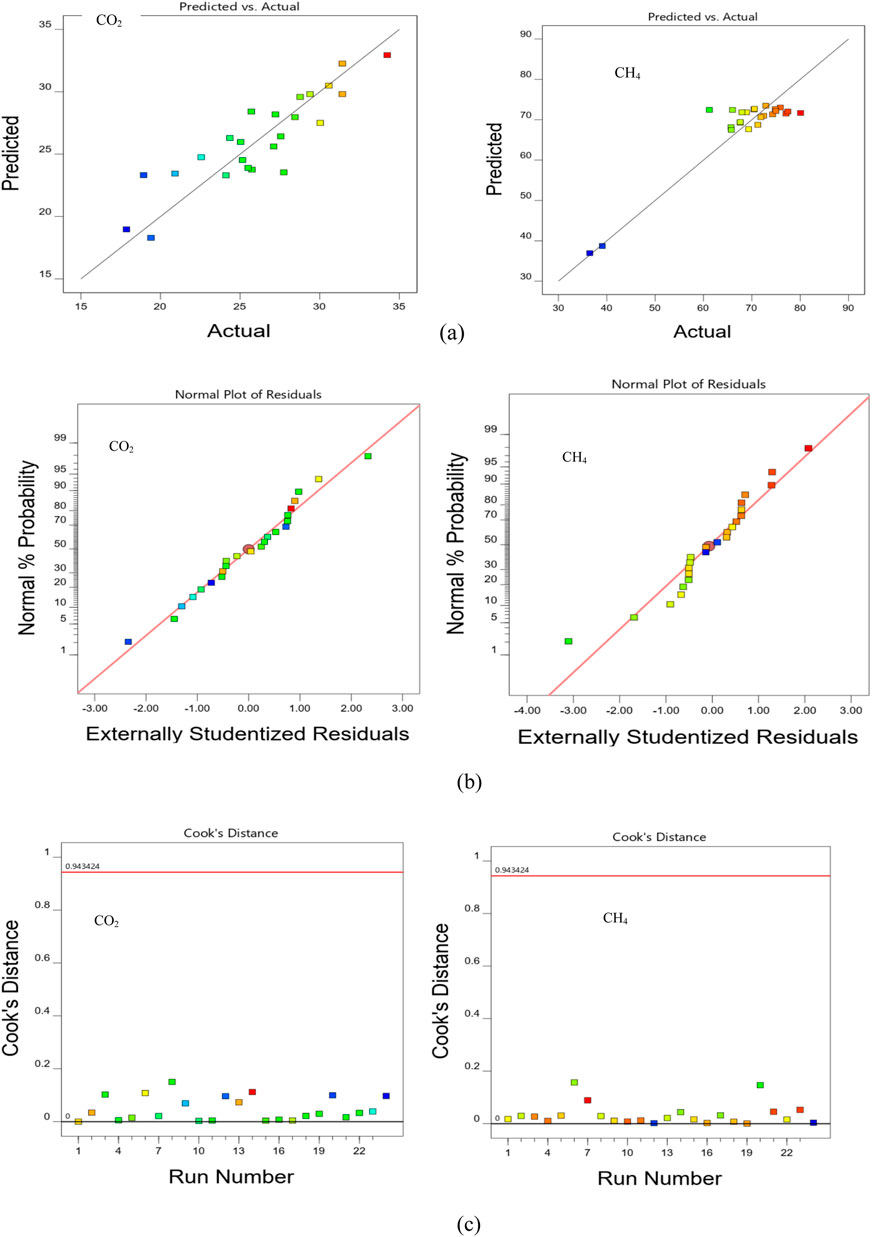
Figure 3. Verification of response fits by (A) analysis of residuals (B) normality and (C) Cook’s distance metrics.
3.2 Results–analysis of significance and effects of biogas production inputs on outcomes
Table 5 presents the F and P values, which are indicators of design significance, derived from the analysis of variance of the response datasets. The F values are derived from the ratio of the mean square value of the respective variable to that of the corresponding residual mean square value. By statistical benchmarks, F values greater than 4, as revealed in parameters A, C, D, and E are indicators of significance on the model response for CO2, while parameters A, D and E are also reported to be significant on the model response for CH4.
Similarly, the P values which are also significance indicators, representing the probability values suggesting design variable effects on the model, and by statistical benchmarks of less than the default. Value of 0.05, affirms the significance of the names inputs parameters on the respective responses. Thus in this case, this infers that the major contributors to the production of CO2 in the biogas production are the inputs Cassava Water, Bio-Char, Poultry Litter and Dairy Manure as represented respectively by indicators A, C, D and E, while the major contributors to the production of CH4 are Cassava Water, Poultry Litter and Dairy Manure only.
While the analysis of variance provides information on WHAT design parameters are significance on the model outcomes, it does not provide information on HOW they affect the generation of the products. Figures 4A–F which are 3D surface response graphs generated from the plot of the combination of input parameters provide such information. From Figures 4A, B, both cassava water and zeolite contribute to the yield of more CO2 and CH4 at constant values of other inputs. At constant quantities of zeolite, increasing cassava water input increases the yield of both products as indicated by the ascending slope of the respective graphs from left to right as the quantity of cassava water increases. This is due to its rich organic content, high biodegradability and the presence of fermentable substrates. Cassava wastewater contains high levels of organic matter, including starch, cellulose, hemicellulose, and other complex organic compounds (Khongkliang et al., 2015; Peres et al., 2019), and supported by the high volatile solids as can be seen earlier in (Table 1) which characterized cassava wastewater substrates. However, while the production of CO2 increases with increasing zeolite, the rate of production of CH4 decreases as the quantity of zeolite input is increased exclusively. This can be attributed to the increase in total solids concentration which potentially reduced the free available water affecting the transport of metabolites and nutrient in the vicinity of zeolite particles and associated microorganisms. Hence, elevated quantities of zeolite may elevate the apparent viscosity of the medium, impeding the efficient mass transfer between the substrate and the microorganisms crucial for the process, thereby slowing down the overall process. Moreover, alterations in the NH₄⁺–NH₃ equilibrium could further impact the effectiveness of the process (Milán et al., 2001). The synergistic effect of zeolite during anaerobic digestion of organic substrate and its ability to reduce inhibition due to ammonia during AD, and also serve as porous medium for microbial attachment, has been extensively reported (Montalvo et al., 2012; Tang C. C. et al., 2023). The trend observed is consistent with the observation and findings from (Milán et al., 2001) where the concentration of zeolite exceeding 6 g/L negatively affected the accumulated methane production. In our study, after keeping the quantity of zeolite input constant while increasing cassava water input we observed an increase in the production of methane. The degree of steepness of the CH4 graph also indicates that cassava water may be a major influencing ingredient in the generation of methane from the biogas production process.
Figures 4C, D provide information on the effect of Poultry litter and Dairy manure variations on the respective yield of CO2 and CH4 when quantities of other inputs are kept constant. Both inputs show the tendency to linearly increase the yield of any of the products as their quantities are increased, either independently or simultaneously. More interestingly though, the steeper slope presented by the CH4 response plot appears to be an indication of a greater influence of both inputs on the yield of CH4 as compared to that of CO2. This may not be unconnected with the high nitrogen content of animal manure as seen previously in Table 1. The addition of animal dung to a carbon-rich substrate in anaerobic co-digestion has been shown to enhance the methane yield and digestion parameters, leading to improved biogas production efficiency (Renggaman et al., 2021). This improvement is attributed to the synergistic effects of combining animal dung with carbon-rich substrates, which can enhance the overall biodegradability and methane potential of the mixture (Rabii et al., 2021).
Figures 4E, F also presents the influence of “Biochar” and “Time” on the yield of CO2 and CH4 in the biogas production setup. While increasing the biochar content of the inputs tends to generate a relatively slight increase in the production of CO2, the yield appears to decrease with increased time of curation of the input mixture. The highest yield of CO2 appears to be possibly generated in this instance by the highest possible quantity of biochar input but in the least time provided. The opposite however, seems to be the case as indicated in the response of CH4 generation. Increased input of biochar appears to be detrimental to the yield in CH4 going by the downward slope of its response graph from left to right, whereas, allowing the mixture extended time periods contribute to further generation of CH4. This is similar to the observation made with respect to zeolite where excessive addition of porous additives caused an increase in total solids concentration, possibly diminishing the presence of free available water and impeding the transport of metabolites and nutrients in the proximity of biochar particles. Generally, the addition of biochar to anaerobic digestion can have varying effects on methane production. While some studies have shown that appropriate addition of biochar can significantly promote methanogenesis (Achi et al., 2020), others have indicated that high doses of biochar can negatively affect methane yield (Fan et al., 2022). According to previous reports, the alkaline functional groups on the surface of biochar can improve the buffering capacity and stability of AD process (Qi C. et al., 2021; Qi Q. et al., 2021), effectively alleviate acid accumulation and provide a stable pH environment for methanogenic microorganisms. However, it is important to highlight that anaerobic digestion (AD) requires a neutral environment. Excessive biochar presence can elevate the pH level of the digesting slurry, consequently hampering the activity of anaerobic microorganisms (Cui et al., 2021).
While the response surface plots seem to have explained how the various biogas production ingredients may affect the yield of each of the products, the extent of their influence and the hierarchy of their effects as shown in Figure 5 is of equivalent interest. The analysis of variance and surface response may have informed us about the significance or lack thereof, of the inputs, a further analysis of the differentials of the responses to the individual design variables was carried out. By simply computing the ratios of the change
Figure 6 presents the degree of influence of each input on the corresponding output. While increasing every other parameter except the amount of time utilized in the process will lead to an increase in the yield of CO2, increasing the quantity of zeolite and time can be detrimental to the production of CH4 The only way to simultaneously generate more CO2 and less CH4 appears to be by increasing the quantity of zeolite and biochar supplied into the process while keeping other inputs constant while providing an extended time for curation seems to be the only way to retard the generation of CO2. Dairy manure and Poultry litter apparently have near same effects or influence on the generation of both CO2 and CH4, thus for an application of a biogas production process that supports the simultaneous yield of both products, increasing the quantities of dairy manure and poultry litter fed into the plant becomes imperative. While Cassava water may not be substantially influential in producing CO2, it is remarkably significant to the production of CH4. Thus, considering the inferences derived from Figures 5, 6, the hierarchy of influence of the various inputs factors, in order of decreasing influence, on the generation of the respective responses is presented in the Table 6 below.
4 Conclusion
In this parametric study, Cassava wastewater (CW) showed positive results as a viable substrate for biomethane production, and increasing the amount of CW directly correlates with an increase in CH4 production due to its rich organic content, high biodegradability and the presence of fermentable substrates. The addition of Zeolites and biochar supported the increased yield of CO2 and CH4, although the exclusive increase in the quantity of zeolite or biochar reduced the rate of production of CH4, due to the increase in total solids concentration which potentially reduced the free available water affecting the transport of metabolites and nutrient in the vicinity of zeolite or biochar particles and associated microorganisms. Co-digestion of carbon-rich substrate CW with Nitrogen-rich animal dung enhance the methane yield and digestion parameters, leading to improved biogas production efficiency, due to a balanced Carbon-Nitrogen ratio. In general, for all treatment mixes, an increase in time showed a decrease in CO2 and a steady increase in CH4. This study has the potential for a far-reaching practical application in the development of optimized AD systems for cassava wastewater treatment in agricultural and industrial settings. By incorporating specific additives and conductive materials, these systems can be tailored to maximize biogas production, reduce waste, and lower the environmental impact. This can provide a sustainable energy source for rural communities and industries, contributing to energy self-sufficiency and reducing reliance on conventional fossil fuels.
Further studies should focus on developing efficient mechanism for the separation, capture and purification of the CO2 component of biogas for sustainable use in food industries and for agriculture. There is a need for further studies to investigate how biochars from different lignocellulosic agricultural residues, produced at varying operating parameters can contribute in optimizing biogas production from cassava wastes. Furthermore, a comprehensive investigation is needed to understand the physicochemical attributes of biochar and interactions with microbial consortia, that significantly enhance the overall AD process.
Data availability statement
The raw data supporting the conclusions of this article will be made available by the authors, without undue reservation.
Author contributions
CA: Conceptualization, Data curation, Investigation, Methodology, Software, Validation, Visualization, Writing–original draft, Writing–review and editing. WK: Funding acquisition, Project administration, Resources, Supervision, Writing–original draft, Writing–review and editing. JS: Funding acquisition, Project administration, Resources, Supervision, Writing–original draft, Writing–review and editing. JN: Funding acquisition, Project administration, Resources, Supervision, Writing–original draft, Writing–review and editing. FF: Data curation, Formal Analysis, Investigation, Methodology, Software, Validation, Visualization, Writing–original draft, Writing–review and editing.
Funding
The author(s) declare that financial support was received for the research, authorship, and/or publication of this article. Department of Civil Engineering, Faculty of Engineering and the Built Environment, Tshwane University of Technology.
Acknowledgments
The authors appreciate the financial support received from the Department of Civil Engineering, Faculty of Engineering and the Built Environment, Tshwane University of Technology, Pretoria, South Africa towards this research work. The first author acknowledges the Water Quality Laboratory of the University of Maryland’s Department of Environmental Science and Technology (ENST) at the University of Maryland, College Park, United States.
Conflict of interest
The authors declare that the research was conducted in the absence of any commercial or financial relationships that could be construed as a potential conflict of interest.
The reviewer OA declared a shared affiliation with the authors to the handling editor at the time of review.
Publisher’s note
All claims expressed in this article are solely those of the authors and do not necessarily represent those of their affiliated organizations, or those of the publisher, the editors and the reviewers. Any product that may be evaluated in this article, or claim that may be made by its manufacturer, is not guaranteed or endorsed by the publisher.
References
Abbas, Y., Yun, S., Mehmood, A., Shah, F. A., Wang, K., Eldin, E. T., et al. (2023). Co-digestion of cow manure and food waste for biogas enhancement and nutrients revival in bio-circular economy. Chemosphere 311, 137018. doi:10.1016/j.chemosphere.2022.137018
Achi, C. G., Hassanein, A., and Lansing, S. (2020). Enhanced biogas production of cassava wastewater using zeolite and biochar additives and manure co-digestion. Energies 13 (2), 491. doi:10.3390/en13020491
Adams, R. C., Bennett, F. M., Dixon, J. K., Lough, R. C., Maclean, F. S., and Martin, G. I. (1951). The utilization of organic wastes in NZ. N. Z. Eng. 6 (11), 396–424.
Arif, S., Liaquat, R., and Adil, M. (2018). Applications of materials as additives in anaerobic digestion technology. Renew. Sustain. Energy Rev. 97, 354–366. doi:10.1016/j.rser.2018.08.039
Batstone, D. J., Keller, J., Angelidaki, I., Kalyuzhnyi, S. V., Pavlostathis, S. G., Rozzi, A., et al. (2002). The IWA anaerobic digestion model no 1 (ADM1). Water Sci. Technol. 45 (10), 65–73. doi:10.2166/wst.2002.0292
Buffière, P., Dooms, M., Hattou, S., and Benbelkacem, H. (2018). The hydrolytic stage in high solids temperature phased anaerobic digestion improves the downstream methane production rate. Bioresour. Technol. 259, 111–118. doi:10.1016/j.biortech.2018.03.037
Cai, J., He, P., Wang, Y., Shao, L., and Lü, F. (2016). Effects and optimization of the use of biochar in anaerobic digestion of food wastes. Waste Manag. and Res. 34 (5), 409–416. doi:10.1177/0734242x16634196
Chow, W. L., Chong, S., Lim, J. W., Chan, Y. J., Chong, M. F., Tiong, T. J., et al. (2020). Anaerobic co-digestion of wastewater sludge: a review of potential co-substrates and operating factors for improved methane yield. Processes 8 (1), 39. doi:10.3390/pr8010039
Cui, Y., Mao, F., Zhang, J., He, Y., Tong, Y. W., and Peng, Y. (2021). Biochar enhanced high-solid mesophilic anaerobic digestion of food waste: cell viability and methanogenic pathways. Chemosphere 272, 129863. doi:10.1016/j.chemosphere.2021.129863
Deenik, J. L., and Cooney, M. J. (2016). The potential benefits and limitations of corn cob and sewage sludge biochars in an infertile Oxisol. Sustainability 8 (2), 131. doi:10.3390/su8020131
Di Capua, F., Spasiano, D., Giordano, A., Adani, F., Fratino, U., Pirozzi, F., et al. (2020). High-solid anaerobic digestion of sewage sludge: challenges and opportunities. Appl. Energy 278, 115608. doi:10.1016/j.apenergy.2020.115608
Fagbohungbe, M. O., Herbert, B. M., Hurst, L., Ibeto, C. N., Li, H., Usmani, S. Q., et al. (2017). The challenges of anaerobic digestion and the role of biochar in optimizing anaerobic digestion. Waste Manag. 61, 236–249. doi:10.1016/j.wasman.2016.11.028
Fan, Q., Shao, Z., Guo, X., Zhou, Q., and Qiu, L. (2022). Process optimization of the anaerobic co-digestion of Alternanthera philoxeroides and corn straw enhanced by biochar and Fe3O4.
Ferdeș, M., Paraschiv, G., Ionescu, M., Dincă, M. N., Moiceanu, G., and Zăbavă, B. Ș. (2023). Anaerobic Co-digestion: a way to potentiate the synergistic effect of multiple substrates and microbial diversity. Energies 16 (5), 2116. doi:10.3390/en16052116
Ghaleb, A. A. S., Kutty, S. R. M., Ho, Y.-C., Jagaba, A. H., Noor, A., Al-Sabaeei, A. M., et al. (2020). Response surface methodology to optimize methane production from mesophilic anaerobic co-digestion of oily-biological sludge and sugarcane bagasse. Sustainability 12 (5), 2116. doi:10.3390/su12052116
González, R., Peña, D. C., and Gómez, X. (2022). Anaerobic co-digestion of wastes: reviewing current status and approaches for enhancing biogas production. Appl. Sci. 12 (17), 8884. doi:10.3390/app12178884
Gujer, W., and Zehnder, A. J. (1983). Conversion processes in anaerobic digestion. Water Sci. Technol. 15 (8–9), 127–167. doi:10.2166/wst.1983.0164
Gunnerson, C. G., and Stuckey, D. C. (1986). “Anaerobic digestion: principles and practices for biogas systems,” in World Bank technical paper (Washington, DC (USA): International Bank for Reconstruction and Development).
Hassanein, A., Lansing, S., and Tikekar, R. (2019). Impact of metal nanoparticles on biogas production from poultry litter. Bioresour. Technol. 275, 200–206. doi:10.1016/j.biortech.2018.12.048
Jadhav, P., Muhammad, N., Bhuyar, P., Krishnan, S., Abd Razak, A. S., Zularisam, A. W., et al. (2021). A review on the impact of conductive nanoparticles (CNPs) in anaerobic digestion: applications and limitations. Environ. Technol. and Innovation 23, 101526. doi:10.1016/j.eti.2021.101526
Karki, R., Chuenchart, W., Surendra, K. C., Shrestha, S., Raskin, L., Sung, S., et al. (2021). Anaerobic co-digestion: current status and perspectives. Bioresour. Technol. 330, 125001. doi:10.1016/j.biortech.2021.125001
Karrabi, M., Ranjbar, F. M., Shahnavaz, B., and Seyedi, S. (2023). A comprehensive review on biogas production from lignocellulosic wastes through anaerobic digestion: an insight into performance improvement strategies. Fuel 340, 127239. doi:10.1016/j.fuel.2022.127239
Khan, M., Chuenchart, W., Surendra, K. C., and Khanal, S. K. (2023). Applications of artificial intelligence in anaerobic co-digestion: recent advances and prospects. Bioresour. Technol. 370, 128501. doi:10.1016/j.biortech.2022.128501
Khongkliang, P., Kongjan, P., and Sompong, O. (2015). Hydrogen and methane production from starch processing wastewater by thermophilic two-stage anaerobic digestion. Energy Procedia 79, 827–832. doi:10.1016/j.egypro.2015.11.573
Kumar, S., Kumar, R., and Pandey, A. (2021). Current developments in biotechnology and bioengineering: strategic perspectives in solid waste and wastewater management. Elsevier.
Kumar S. S., S. S., Ghosh, P., Kataria, N., Kumar, D., Thakur, S., Pathania, D., et al. (2021). The role of conductive nanoparticles in anaerobic digestion: mechanism, current status and future perspectives. Chemosphere 280, 130601. doi:10.1016/j.chemosphere.2021.130601
Kunatsa, T., and Xia, X. (2022). A review on anaerobic digestion with focus on the role of biomass co-digestion, modelling and optimisation on biogas production and enhancement. Bioresour. Technol. 344, 126311. doi:10.1016/j.biortech.2021.126311
Lallement, A., Peyrelasse, C., Lagnet, C., Barakat, A., Schraauwers, B., Maunas, S., et al. (2023). A detailed database of the chemical properties and methane potential of biomasses covering a large range of common agricultural biogas plant feedstocks. Waste 1 (1), 195–227. doi:10.3390/waste1010014
Lindmark, J., Thorin, E., Fdhila, R. B., and Dahlquist, E. (2014). Effects of mixing on the result of anaerobic digestion. Renew. Sustain. Energy Rev. 40, 1030–1047. doi:10.1016/j.rser.2014.07.182
Meegoda, J. N., Li, B., Patel, K., and Wang, L. B. (2018). A review of the processes, parameters, and optimization of anaerobic digestion. Int. J. Environ. Res. Public Health 15 (10), 2224. doi:10.3390/ijerph15102224
Milán, Z., Sánchez, E., Weiland, P., Borja, R., Martın, A., and Ilangovan, K. (2001). Influence of different natural zeolite concentrations on the anaerobic digestion of piggery waste. Bioresour. Technol. 80 (1), 37–43. doi:10.1016/s0960-8524(01)00064-5
Montalvo, S., Guerrero, L., Borja, R., Sánchez, E., Milán, Z., Cortés, I., et al. (2012). Application of natural zeolites in anaerobic digestion processes: a review. Appl. Clay Sci. 58, 125–133. doi:10.1016/j.clay.2012.01.013
Moody, L. B., Burns, R. T., Bishop, G., Sell, S. T., and Spajic, R. (2011). Using biochemical methane potential assays to aid in co-substrate selection for co-digestion. Appl. Eng. Agric. 27 (3), 433–439. doi:10.1016/j.renene.2023.04.125
Mupakati, T., and Tanyanyiwa, V. I. (2017). Cassava production as a climate change adaptation strategy in Chilonga Ward, Chiredzi District, Zimbabwe. Jàmbá J. Disaster Risk Stud. 9 (1), 348–410. doi:10.4102/jamba.v9i1.348
Nguyen, V. K., Chaudhary, D. K., Dahal, R. H., Trinh, N. H., Kim, J., Chang, S. W., et al. (2021). Review on pretreatment techniques to improve anaerobic digestion of sewage sludge. Fuel 285, 119105. doi:10.1016/j.fuel.2020.119105
Osman, A. I., Lai, Z. Y., Farghali, M., Yiin, C. L., Elgarahy, A. M., Hammad, A., et al. (2023). Optimizing biomass pathways to bioenergy and biochar application in electricity generation, biodiesel production, and biohydrogen production. Environ. Chem. Lett. 21 (5), 2639–2705. doi:10.1007/s10311-023-01613-2
Otekunrin, O. A., and Sawicka, B. (2019). Cassava, a 21st century staple crop: how can Nigeria harness its enormous trade potentials. Acta Sci. Agric. 3 (8), 194–202. doi:10.31080/asag.2019.03.0586
Palma, D., Fuess, L. T., de Lima-Model, A. N., da Conceição, K. Z., Cereda, M. P., Tavares, M. H. F., et al. (2018). Using dolomitic limestone to replace conventional alkalinization in the biodigestion of rapid acidification cassava processing wastewater. J. Clean. Prod. 172, 2942–2953. doi:10.1016/j.jclepro.2017.11.118
Paranjpe, A., Saxena, S., and Jain, P. (2023). A review on performance improvement of anaerobic digestion using co-digestion of food waste and sewage sludge. J. Environ. Manag. 338, 117733. doi:10.1016/j.jenvman.2023.117733
Paritosh, K., Yadav, M., Chawade, A., Sahoo, D., Kesharwani, N., Pareek, N., et al. (2020). Additives as a support structure for specific biochemical activity boosts in anaerobic digestion: a review. Front. Energy Res. 8, 88. doi:10.3389/fenrg.2020.00088
Paudel, S. R., Banjara, S. P., Choi, O. K., Park, K. Y., Kim, Y. M., and Lee, J. W. (2017). Pretreatment of agricultural biomass for anaerobic digestion: current state and challenges. Bioresour. Technol. 245, 1194–1205. doi:10.1016/j.biortech.2017.08.182
Peres, S., Monteiro, M. R., Ferreira, M. L., do Nascimento Junior, A. F., and de Los Angeles Perez Fernandez Palha, M. (2019). Anaerobic digestion process for the production of biogas from cassava and sewage treatment plant sludge in Brazil. BioEnergy Res. 12, 150–157. doi:10.1007/s12155-018-9942-z
Pilarska, A. A., Kulupa, T., Kubiak, A., Wolna-Maruwka, A., Pilarski, K., and Niewiadomska, A. (2023). Anaerobic digestion of food waste—a short review. Energies 16 (15), 5742. doi:10.3390/en16155742
Qi, C., Wang, R., Jia, S., Chen, J., Li, Y., Zhang, J., et al. (2021). Biochar amendment to advance contaminant removal in anaerobic digestion of organic solid wastes: a review. Bioresour. Technol. 341, 125827. doi:10.1016/j.biortech.2021.125827
Qi, Q., Sun, C., Zhang, J., He, Y., and Tong, Y. W. (2021). Internal enhancement mechanism of biochar with graphene structure in anaerobic digestion: the bioavailability of trace elements and potential direct interspecies electron transfer. Chem. Eng. J. 406, 126833. doi:10.1016/j.cej.2020.126833
Rabii, A., Koupaie, E. H., Aldin, S., Dahman, Y., and Elbeshbishy, E. (2021). Methods of pretreatment and their impacts on anaerobic codigestion of multifeedstocks: a review. Water Environ. Res. 93 (12), 2834–2852. doi:10.1002/wer.1636
Rajput, A. A., and Sheikh, Z. (2019). Effect of inoculum type and organic loading on biogas production of sunflower meal and wheat straw. Sustain. Environ. Res. 29, 4–10. doi:10.1186/s42834-019-0003-x
Renggaman, A., Choi, H. L., Sudiarto, S. I. A., Febrisiantosa, A., Ahn, D. H., Choung, Y. W., et al. (2021). Biochemical methane potential of swine slaughter waste, swine slurry, and its codigestion effect. Energies 14 (21), 7103. doi:10.3390/en14217103
Rice, E. W., Bridgewater, L., and Association, A. P. H. (2012). Standard methods for the examination of water and wastewater, 10. Washington, DC: American public health association.
Rincón, B., Fernández-Rodríguez, M. J., de la Lama-Calvente, D., and Borja, R. (2018). “The influence of microalgae addition as co-substrate in anaerobic digestion processes,” in Microalgal biotechnology (IntechOpen Riejeka), 59–90.
Sevillano, C. A., Pesantes, A. A., Peña Carpio, E., Martínez, E. J., and Gómez, X. (2021). Anaerobic digestion for producing renewable energy—the evolution of this technology in a new uncertain scenario. Entropy 23 (2), 145. doi:10.3390/e23020145
Siddique, M. N. I., and Wahid, Z. A. (2018). Achievements and perspectives of anaerobic co-digestion: a review. J. Clean. Prod. 194, 359–371. doi:10.1016/j.jclepro.2018.05.155
Silwadi, M., Mousa, H., Al-Hajji, B. Y., Al-Wahaibi, S. S., and Al-Harrasi, Z. Z. (2023). Enhancing biogas production by anaerobic digestion of animal manure. Int. J. Green Energy 20 (3), 257–264. doi:10.1080/15435075.2022.2038608
Soltanian, S., Aghbashlo, M., Almasi, F., Hosseinzadeh-Bandbafha, H., Nizami, A.-S., Ok, Y. S., et al. (2020). A critical review of the effects of pretreatment methods on the exergetic aspects of lignocellulosic biofuels. Energy Convers. Manag. 212, 112792. doi:10.1016/j.enconman.2020.112792
Song, Y., Qiao, W., Westerholm, M., Huang, G., Taherzadeh, M. J., and Dong, R. (2023). Microbiological and technological insights on anaerobic digestion of animal manure: a review. Fermentation 9 (5), 436. doi:10.3390/fermentation9050436
Tabatabaei, M., Aghbashlo, M., Valijanian, E., Panahi, H. K. S., Nizami, A.-S., Ghanavati, H., et al. (2020). A comprehensive review on recent biological innovations to improve biogas production, Part 2: mainstream and downstream strategies. Renew. Energy 146, 1392–1407. doi:10.1016/j.renene.2019.07.047
Tang, C. C., Zhang, B.-C., Yao, X.-Y., Sangeetha, T., Zhou, A.-J., Liu, W., et al. (2023). Natural zeolite enhances anaerobic digestion of waste activated sludge: insights into the performance and the role of biofilm. J. Environ. Manag. 345, 118704. doi:10.1016/j.jenvman.2023.118704
Tang, S., Wang, Z., Lu, H., Si, B., Wang, C., and Jiang, W. (2023). Design of stage-separated anaerobic digestion: principles, applications, and prospects. Renew. Sustain. Energy Rev. 187, 113702. doi:10.1016/j.rser.2023.113702
Wang, P., Li, X., Li, Y., Su, Y., Wu, D., and Xie, B. (2023). Enhanced anaerobic digestion performance of food waste by zero-valent iron and iron oxides nanoparticles: comparative analyses of microbial community and metabolism. Bioresour. Technol. 371, 128633. doi:10.1016/j.biortech.2023.128633
Wang, S., Wei, Z., and Wang, L. (2024). Improving slaughterhouse byproducts utilization via anaerobic digestion, composting, and rendering. Renew. Sustain. Energy Rev. 189, 113881. doi:10.1016/j.rser.2023.113881
Wei, W., Guo, W., Ngo, H. H., Mannina, G., Wang, D., Chen, X., et al. (2020). Enhanced high-quality biomethane production from anaerobic digestion of primary sludge by corn stover biochar. Bioresour. Technol. 306, 123159. doi:10.1016/j.biortech.2020.123159
Xie, S., Higgins, M. J., Bustamante, H., Galway, B., and Nghiem, L. D. (2018). Current status and perspectives on anaerobic co-digestion and associated downstream processes. Environ. Sci. Water Res. and Technol. 4 (11), 1759–1770. doi:10.1039/c8ew00356d
Xu, F., Li, Y., Ge, X., Yang, L., and Li, Y. (2018). Anaerobic digestion of food waste–Challenges and opportunities. Bioresour. Technol. 247, 1047–1058. doi:10.1016/j.biortech.2017.09.020
Yan, H., Zhao, C., Zhang, J., Zhang, R., Xue, C., Liu, G., et al. (2017). Study on biomethane production and biodegradability of different leafy vegetables in anaerobic digestion. Amb. Express 7, 27–29. doi:10.1186/s13568-017-0325-1
Zeng, Y., Guo, Q., Hu, X., Lu, J., Fan, X., Wu, H., et al. (2024). Improving the signal-to-noise ratio of axial displacement measurements of microspheres based on compound digital holography microscopy combined with the reconstruction centering method. Sensors 24 (9), 2723. doi:10.3390/s24092723
Zheng, Y., Zhao, J., Xu, F., and Li, Y. (2014). Pretreatment of lignocellulosic biomass for enhanced biogas production. Prog. Energy Combust. Sci. 42, 35–53. doi:10.1016/j.pecs.2014.01.001
Keywords: anaerobic co-digestion, porous additives, conductive materials, parametric analysis, bioenergy, organic waste
Citation: Achi CG, Kupolati WK, Snyman J, Ndambuki JM and Fameso FO (2024) Investigating the effects of biochars and zeolites in anaerobic digestion and co-digestion of cassava wastewater with livestock manure. Front. Energy Res. 12:1386550. doi: 10.3389/fenrg.2024.1386550
Received: 15 February 2024; Accepted: 21 October 2024;
Published: 01 November 2024.
Edited by:
Shiv Prasad, Indian Agricultural Research Institute (ICAR), IndiaReviewed by:
Shiplu Sarker, NTNU, NorwayOlatunji Abolusoro, Tshwane University of Technology, South Africa
Anoop Singh, Ministry of Science and Technology, India
Copyright © 2024 Achi, Kupolati, Snyman, Ndambuki and Fameso. This is an open-access article distributed under the terms of the Creative Commons Attribution License (CC BY). The use, distribution or reproduction in other forums is permitted, provided the original author(s) and the copyright owner(s) are credited and that the original publication in this journal is cited, in accordance with accepted academic practice. No use, distribution or reproduction is permitted which does not comply with these terms.
*Correspondence: C. G. Achi, YWNoaWNnQHR1dC5hYy56YQ==