- 1Power Grid Planning Research Center, Guangxi Power Grid, Nanning, Guangxi, China
- 2Energy Development Research Institute, China Southern Power Grid, Guangzhou, Guangdong, China
- 3School of Energy and Power Engineering, Huazhong University of Science and Technology, Wuhan, China
The deployment of energy storage systems can play a role in peak and frequency regulation, solve the issue of limited flexibility in cleaner power systems in China, and ensure the stability and safety of the power grid. This paper presents a comprehensive environmental impact analysis of a lithium iron phosphate (LFP) battery system for the storage and delivery of 1 kW-hour of electricity. Quantities of copper, graphite, aluminum, lithium iron phosphate, and electricity consumption are set as uncertainty and sensitivity parameters with a variation of [90%, 110%]. The results show that global warming potential is 9.08E+01 kg CO2 eq. and fossil resource use is 1.21E+03 MJ, with uncertainty ranges of [8.54E+01, 9.23E+01] and [1.15E+03, 1.23E+03], respectively. Electricity consumption during the manufacture and installation process is the greatest contributor to climate change (CO2 eq. emissions), accounting for 39.71% and largely owing to non-renewable sources, followed by cathode materials at 27.85% and anode materials at 18.36%. The disposal and recycling process offers emission reductions but requires an additional 1.17% use of fossil resources. Sobol T indices for the quantity of electricity are the highest for acidification, climate change, fossil resource use, and ionizing radiation. By considering the pathway of China’s electricity mix from 2020 to 2050 under the Paris Agreement’s 2° target, the potential for environmental emission reduction in the system is evaluated. The results show that the greener electricity mix could lead to a 24.59% reduction in acidification impact, a 35.74% reduction in climate change impact, a 33.24% reduction in fossil resource use, and a 44.13% reduction in ionizing radiation impact. This study offers a comprehensive view of the environmental impact reductions associated with the lithium iron phosphate battery and its industry.
1 Introduction
Moving toward carbon neutrality has become a consensus among major countries in the world. In recent years, the development of energy storage technology has become a key point in the global transition to sustainable energy systems. This is particularly relevant in the context of China, a nation that has been rapidly expanding its renewable energy capacity (Kersey et al., 2022). The integration of energy storage solutions is essential for managing the intermittency of renewable energy sources such as solar and wind power. With the in-depth implementation of the dual-carbon goal and energy revolution, China’s energy storage technology and industry have gained momentum (Shen et al., 2019), which can be reflected by several key developments: active research in energy storage technology, rapid growth in the scale of the energy storage market, growing interest from the capacity market, increasing maturity of the energy storage industry supply chain, and escalating policy support for energy storage.
Among various energy storage technologies, lithium iron phosphate (LFP) (LiFePO4) batteries have emerged as a promising option due to their unique advantages (Chen et al., 2009; Li and Ma, 2019). Lithium iron phosphate batteries offer several benefits over traditional lithium-ion batteries, including a longer cycle life, enhanced safety, and a more stable thermal and chemical structure (Ouyang et al., 2015; Olabi et al., 2021). These attributes make them particularly suitable for large-scale energy storage applications, which are crucial in China, given its significant growth in renewable energy deployment. The stability and longevity of LiFePO4 batteries can lead to more reliable and efficient energy storage systems, which are vital for ensuring a consistent energy supply in the face of fluctuating renewable energy sources (Miller et al., 2018). Although the advantages of lithium iron phosphate batteries are clear, it is important to evaluate their environmental impacts (Sullivan and Gaines, 2010; Dehghani-Sanij et al., 2019).
The production and disposal of these batteries involve a variety of processes that could potentially have significant environmental impacts. These include the extraction of raw materials, manufacturing processes, energy consumption during usage, and the management of end-of-life batteries. Understanding the full environmental impact of LiFePO4 batteries is crucial for assessing their sustainability and guiding policy and decision-making in the energy sector. Sadhukhan and Christensen (2021) conducted a life cycle environmental analysis of lithium-ion batteries, analyzing their life cycle environmental impact hotspots, battery energy storage system (BESS) sustainability hotspots, and ways to improve renewable electricity infrastructure; however, sensitivity analysis was not included in the research. Tan (2017) comparatively analyzed the life cycle GHG emissions of four battery energy storage technologies, namely, lead–acid batteries (PbA), lithium-ion batteries (Li-ion), sodium–sulfur batteries (NaS), and vanadium redox batteries (VFBs), and emphasized that BESS should be placed in power system application scenarios and analyzed with a systematic approach. Han et al. (2023) conducted life cycle environmental analysis of three important electrochemical energy storage technologies, namely, lithium iron phosphate battery (LFPB), nickel cobalt manganese oxide battery (NCMB), and vanadium redox battery (VFRB). They developed a cradle-to-grave life cycle analysis model to validate the carbon reduction benefits of electrochemical energy storage technologies based on a case study of energy stored per megawatt-hour; however, but they did not consider the recycling process. Rahman et al. (2021) developed a life cycle assessment model for battery storage systems and evaluated the life cycle greenhouse gas (GHG) emissions of five battery storage systems and found that the lithium-ion battery storage system had the highest life cycle net energy ratio and the lowest GHG emissions for all four stationary application scenarios studied. However, several studies neglected the disposal stage of the system, and few studies focused on the uncertainty and sensitivity analysis to identify the variations in the total results and sensitivity of the parameters (Liang et al., 2017; Wang et al., 2017). In this study, the comprehensive environmental impacts of the lithium iron phosphate battery system for energy storage were evaluated. The contributions of manufacture and installation and disposal and recycling stages were analyzed, and the uncertainty and sensitivity of the overall system were explored. In addition, this study explored the emission reduction potential of the system by 2050 and hoped to present a complete analysis for the storage and delivery of 1 kW-hour (kWh) of electricity from the lithium iron phosphate battery system to the grid.
2 Methods
This study employed the process-based life cycle assessment method to evaluate the environmental impacts of the lithium iron phosphate battery. Life cycle assessment was conducted using the Brightway2 package in Python (Mutel, 2017). The life cycle model consists of activity units composed of exchanged activities, with no intersection of biosphere and technosphere activities, while emissions and material and energy inputs are modeled as exchanges within the technosphere and exchanges between the technosphere and the biosphere. The former constitutes the technosphere matrix A, and the latter constitutes the techno-biosphere matrix B. Brightway2 calculates the life cycle impacts using the following equation:
where g is the life cycle outcome matrix, f is the final demand matrix, B is the techno-biosphere matrix, and A is the technosphere matrix.
2.1 Goal and scope
For the system boundary of this study, the lithium iron phosphate battery system mainly includes manufacture and disposal and recycling. The transportation of raw materials to the battery manufacturing facility was included in the manufacturing process. The process flow diagram (Zhang et al., 2015) is shown in Figure 1.
The defined functional unit for this study is the storage and delivery of one kW-hour (kWh) of electricity from the lithium iron phosphate battery system to the grid. The environmental impact results of the studied system were evaluated based on it.
2.2 Life cycle impact assessment
The impact assessment method selected was environmental footprint (EF) at midpoint level, with the version being EF 3.0. The assessed impact categories of this study were chosen based on the characteristics of the battery system as well as considering the comprehensiveness of the environmental indicators (see Table 1). Normalization was conducted by dividing the results by the normalization factors, and the normalization factors for the impact categories in EF 3.0 were referenced from Crenna et al. (2019).
2.3 Uncertainty and sensitivity analysis
Uncertainty and sensitivity analysis were conducted considering key parameters which may have varieties in the scope of this study. As shown in Table 2, the triangle- density function was employed for the quantity of materials and the parameters of triangular distribution are the most likely value, minimum and maximum value. The linear distribution was selected for quantity of electricity because it’s hard to estimate its amount during the life cycle stage in manufacture, transport, installation, disposal and recycling.
The uncertainty analysis was conducted using the Monte Carlo method, in which 1,000 samples were randomly repeated from the probability distributions to simulate the results. The P10 and P90 values were calculated to serve as the lower and upper limits, respectively, of the uncertainty analysis. There is a 10% chance that the simulation outcome will be equal to or below the P10 value and a 90% chance that it will be below the P90 value. The sensitivity analysis for the system was employed based on one-at-a-time variation and the variance-based Sobol method, with the former focusing on local sensitivity and the latter on global sensitivity (Zhang et al., 2015). The total order Sobol’s index evaluates the contribution of variance from all coefficients, considering their interactions, to the total variance.
3 Life cycle inventories
3.1 Manufacture and installation
The manufacture and installation stage compromises electrode manufacturing, and cell production involves the assembly and finishing processes of the LFP battery. Batteries are constructed using sets of cathode (LFP cathode) and anode (graphite anode) electrodes, which are layered atop each other before being assembled into cells. A separator, specifically a porous membrane, is positioned between the electrodes to facilitate the flow of lithium ions. During the charging phase, lithium ions migrate from the cathode to the liquid electrolyte, passing through the separator’s micropores to reach the graphite anode within the cells. During the charging phase, lithium ions migrate from the cathode through the separator’s micropores and the liquid electrolyte to the graphite anode. Conversely, during discharge, the Li+ ions travel back to the cathode. The form of the cell is the prismatic cell, and the studied process for producing lithium iron phosphate is the solid state process. The prismatic cell was chosen due to its lower cost compared to both the cylindrical and pouch cell types (Mahamud and Park, 2022). The synthesis of solid-state lithium iron phosphate necessitates the use of lithium, iron, and phosphorous compounds. The general reaction is
Life cycle inventories in the manufacture and installation stage are listed, as shown in Tables 3–9. These inventories include considerations of both material and energy inputs and outputs. Data were referenced from the ecoinvent database, version 3.9.1, and other research studies (Wernet et al., 2016; Hao et al., 2017; Liu, 2022).
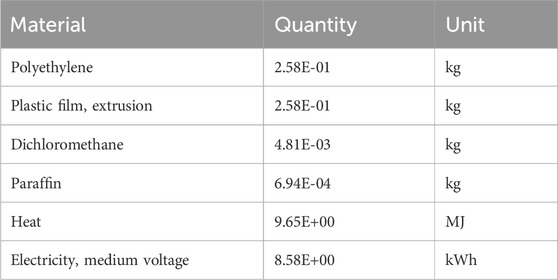
TABLE 8. Material list of the battery separator (Deimede and Elmasides, 2015; Yin et al., 2019).
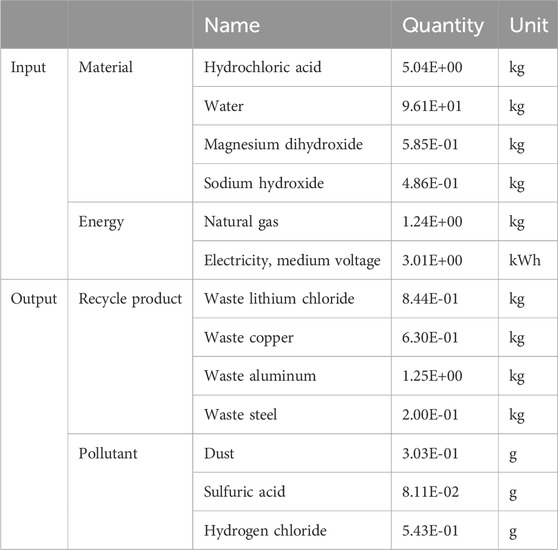
TABLE 9. Input and output data of treating 1 kWh LFP battery (Shu et al., 2021).
3.2 Disposal and recycling
The environmental impacts of this process were calculated using the following equation:
where
Recycling lithium iron phosphate batteries is crucial for their life cycle management, especially considering the environmental implications of battery waste. Among the various recycling techniques (Nordelöf et al., 2019), the hydrometallurgy method is operable at ambient temperature and pressure and achieves high metal selectivity and reaction efficiency, which is more suitable for extracting lithium from retired LFP batteries (Wang et al., 2022). In this recycling process, LiFePO4 batteries are discharged, disassembled, and crushed to extract lithium iron phosphate powder. Subsequently, this powder undergoes heating, pulping, acid leaching, transformation, and alkalization to eliminate impurities. Finally, the resultant purified lithium chloride solution is filtered and used as a recycled product, facilitating the recovery of lithium from decommissioned LiFePO4 batteries. The input and output data of the recycling process are listed in Table 8 (Shu et al., 2021). Moreover, recycled products are assumed to have a loss rate of 90%.
4 Results and discussion
4.1 Environmental impact results
As shown in Table 10, for the function unit of the system, the climate change impact of the LFP battery is 9.08E+01 kg CO2 eq., and resource use from fossils is 1.21E+03 MJ. The top three normalized impact categories are eutrophication (terrestrial), ecotoxicity (freshwater), and ionizing radiation, which indicate that the system has relatively high impacts on these indicators at a global scale and should be paid more attention to. The result of eutrophication (terrestrial) is 1.22E+00 kg N eq, with anode accounting for 47.99% and electricity accounting for 26.03%. Although the cathode’s emission factor is higher than that of the anode in the ecoinvent database, the mass relationship is reversed, which may partially explain the result. The system has 7.17E+03 CTUe ecotoxicity in freshwater, and the anode has the highest share of 83.24%, largely attributed to copper and copper foil. For ionizing radiation, the result is 8.87E+00 kBq U-235 eq, with electricity being the largest contributor at 58.93%. The uncertainty analysis, represented by P10 and P90, indicates the possible range of each impact category. The ranges of resource use (minerals and metals) and ecotoxicity are [-8.97%, +8.77] and [-9.15%, +7.83%], respectively, indicating higher uncertainties. However, ozone depletion has a relatively small level of uncertainty, ranging from −1.13% to +0.89%.
4.2 Contribution analysis of global warming potential
This section examines the climate change impact of the manufacturing and installation processes for the studied LFP battery system, which is identified as an important concern. Figure 2 illustrates the contributions of various components and energy consumption to global warming potential (the indicator of the climate change impact). The largest contributor to global warming potential (GWP) is electricity, accounting for 39.71% of the total impact, and the result is consistent with the literature (Oliveira et al., 2015). This suggests that energy consumed during manufacturing and installation, primarily from non-renewable energy sources, is the most significant factor in the carbon footprint of LFP battery production. Renewable energy and nuclear power generation have smaller emission factors compared to grid power generation now, so promoting the application of renewable energy and nuclear power generation systems is key to reducing emissions in this hot spot. This implication is similar to results from a previous study (Bawankar et al., 2023). The second-largest contribution is the cathode material, which is 27.85%. The contribution of aluminum/aluminum foil reaches 20.58%, while that of lithium iron phosphate is 7.27%. This may be due to the fact that both the quantity and impact factor of aluminum/aluminum foil in the cathode material are higher than those of lithium iron phosphate. The manufacturing and installation of cathode materials is considered energy-intensive and involves high-temperature synthesis processes that lead to GWP. The contribution of anode materials is lower than that of cathode materials, accounting for 18.46% of GWP. Among anode materials, graphite causes more CO2 eq. emissions (10.89%), which is partly because of its higher GHG emissions. In addition, the battery separator accounts for 8.15%, the electrolyte accounts for 5.75%, and the plastic film accounts for the least at only 0.09%. The results show that the most effective way to reduce the GWP of LFP cells is to target greener power sources and improve production efficiency, especially for cathode and anode materials, which together constitute nearly half of the total impact. Additionally, improving the environmental footprint of battery separators and electrolyte production may help further reduce GWP.
4.3 Sensitivity analysis
Figure 3 displays the one-at-a-time sensitivity analysis of five key parameters. The analysis reveals that Q-Ele is the most sensitive parameter affecting acidification, climate change, fossil resource use, ionizing radiation, and ozone depletion, with result variations in the ranges of [96.41%, 103.70%], [95.45%, 104.63%], [95.93%, 103.67%], [93.80%, 106.10%], and [99.77%, 100.27%], respectively. For ecotoxicity and resource use of minerals/metals, Q-copper emerges as the most influential parameter, showing result variations of [84.69%, 115.34%] and [83.76%, 115.63%], respectively.
Table 11 shows the total Sobol indices for the system across the five sensitivity parameters under eight life cycle impacts. It can be seen from Table 11 that the Sobol indices (Sobol T) for CC and REF have similar sensitivity results, primarily to changes in Q-Ele, with indices of 82.75% and 84.27%, respectively. The order of sensitivity from the strongest to the weakest is Q-Ele, followed by Q-Al, Q-C, Q-Cu, and Q-LIP. Both ECO and REM exhibit similar trends, showing that Q-Cu has the highest sensitivity. Furthermore, the corresponding Sobol T indices for Q-Cu are 97.29% and 99.26%. There is relatively low sensitivity to other parameters, meaning that variations in these parameters result in only minor changes to the system’s environmental impacts. ODP is most affected by changes in Q-Al and Q-LIP, the Sobol T of which are 48.02% and 39.20%, respectively. This could be partially attributed to the fact that aluminum, aluminum foil, and LIP have higher impact factors in ODP. The variations in IR are mainly determined by Q-Ele, with much lower sensitivity to other parameters.
4.4 Scenario analysis
According to the results in Section 4.2, electricity constitutes the system’s most emission-intensive component. This section examines the potential for environmental emission reductions, considering changes in China’s electricity mix, and shows the changes in the contribution of the disposal and recycling stage. The structure of the electricity mix in the C2°C scenario is based on the forecast of the Institute of Climate Change and Sustainable Development (ICCSD), which is shown in Table 12. The C2°C scenario is defined as the scenario for achieving the emission reduction target by 2050, in line with the Paris Agreement’s target of limiting global temperature rise to 2°C (ICCSD, 2021).
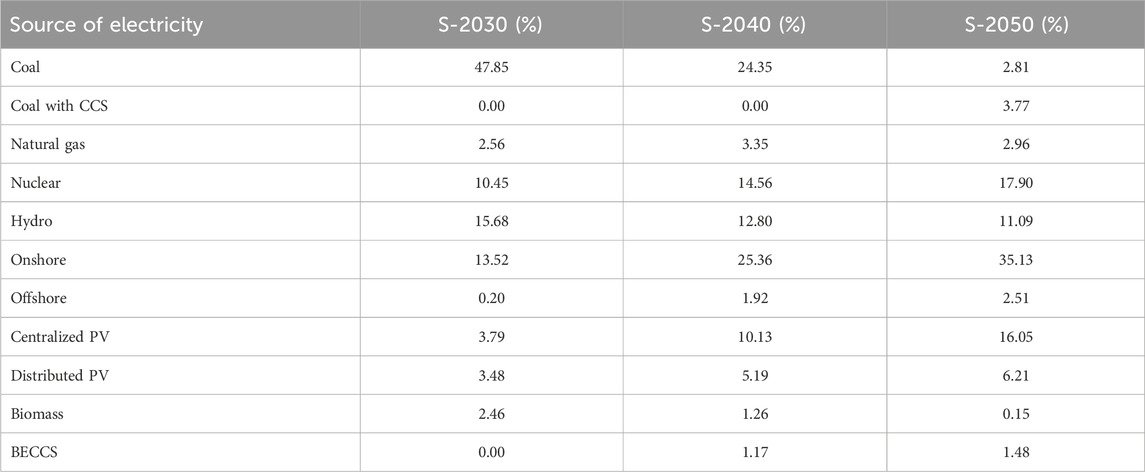
TABLE 12. Forecast of electricity mix of China under C2°C scenario (ICCSD, 2021).
The scatter plots in Figure 4 depict the contribution of the disposal and recycling process to the overall environmental impacts of manufacturing and installation. In the baseline scenario, the disposal and recycling process offers environmental benefits in most impact categories, as indicated by the dark red points are in the negative region. However, for REF and ODP, the environmental burdens from disposal cannot be mitigated by recycling. This suggests that methods used in disposal and recycling are important to reduce material and energy consumption and increase the recycling rate of the material, thereby decreasing overall environmental influences. Changes in the electricity mix have little influence on the contribution of the disposal and recycling process because energy consumption at this stage is relatively small compared to that during the manufacture and installation stage.
According to the bar graph in Figure 4, a cleaner electricity system could significantly reduce GHG emissions to 69.50% by 2040 and 64.26% by 2050. In addition, AC is projected to decrease to 75.41%, REF to 66.76%, and IR to 55.87% between 2020 and 2050. However, REM in 2050 shows an increase of 4.48% compared to the baseline scenario. This is partly due to the greater proportion of electricity generated from photovoltaic and wind sources, as the equipment for these sources requires more metals and minerals. In addition, ODP exhibits minimal variations, which may be attributed to the limited contribution of electricity to this impact.
5 Conclusion
This study has presented a detailed environmental impact analysis of the lithium iron phosphate battery for energy storage using the Brightway2 LCA framework. The results of acidification, climate change, ecotoxicity, energy resources, eutrophication, ionizing radiation, material resources, and ozone depletion were calculated. Uncertainty and sensitivity analysis were conducted to identify how variations in the quantities of copper, graphite, aluminum, lithium iron phosphate, and electricity consumption affect the results. A scenario analysis was carried out, considering the dynamic path of the electricity mix in China until 2050.
The study evaluates that the storage and delivery of one kW-hour (kWh) of electricity from the lithium iron phosphate battery system could cause 9.08E+01 kg CO2 eq. emissions and use 1.21E+03 MJ fossil resources. Eutrophication (terrestrial), ecotoxicity (freshwater), and ionizing radiation are three important impact categories for the LFP battery system with higher normalization values. The disposal and recycling process is important for mitigating the environmental impacts of acidification, climate change, freshwater ecotoxicity, terrestrial eutrophication, and mineral and metal use, offering environmental benefits ranging from −46.60% to −11.59%. However, this process exerts an environmental burden of 101.84% on ozone depletion. The top three contributors to climate change are electricity consumption and cathode and anode production and installation, with contributions of 39.71%, 27.85%, and 18.46%, respectively. In the C2°C scenario, the electricity system composed of more renewable energy and nuclear energy could significantly reduce GHG emissions, with 30.50% emission reductions by 2040 and 35.74% reductions by 2050.
The analysis reveals that under one-at-a-time variations, electricity consumption is the most sensitive parameter affecting acidification, climate change, fossil resource use, ionizing radiation, and ozone depletion. When the parameters change simultaneously and interact with each other, the Sobol T indices of electricity consumption rank first in acidification, climate change, terrestrial eutrophication, and ionizing radiation, with results of 57.82%, 82.75%, 84.27%, and 96.38%, respectively. However, for ozone depletion, Q-Al dominates the Sobol T indices, partially due to its high-influence factor in ODP.
For further reducing the environmental impacts, progress in disposal and recycling methods for lithium iron phosphate batteries is needed to reduce emissions from disposal inputs and increase the recycling rate. Employing cleaner energy sources during the life cycle stages of LFP batteries is also an effective measure. Future studies can explore the life cycle assessment of variable renewable energy and energy storage combined systems to better understand the environmental impacts of the operation and maintenance phases of lithium iron phosphate batteries for energy storage.
Data availability statement
The original contributions presented in the study are included in the article/Supplementary material; further inquiries can be directed to the corresponding authors.
Author contributions
XL: conceptualization, project administration, validation, writing–original draft, writing–review and editing, data curation, and funding acquisition. WM: funding acquisition, supervision, writing–original draft, and writing–review and editing. MY: writing–review and editing and resources. ZY: software and writing–review and editing. QL: writing–review and editing and validation. ZR: writing–review and editing and formal analysis. TZ: data curation and writing–review and editing. YC: methodology, supervision, writing–review and editing, writing–original draft, and investigation.
Funding
The author(s) declare that financial support was received for the research, authorship, and/or publication of this article. This work was supported by the Science and Technology Project of the Guangxi Power Grid (Grant No. GXKJXM20222198).
Conflict of interest
The authors declare that the research was conducted in the absence of any commercial or financial relationships that could be construed as a potential conflict of interest.
Publisher’s note
All claims expressed in this article are solely those of the authors and do not necessarily represent those of their affiliated organizations, or those of the publisher, the editors, and the reviewers. Any product that may be evaluated in this article, or claim that may be made by its manufacturer, is not guaranteed or endorsed by the publisher.
References
Bawankar, S., Dwivedi, G., Nanda, I., Daniel Jiménez Macedo, V., Kesharvani, S., Meshram, K., et al. (2023). Environmental impact assessment of lithium ion battery employing cradle to grave. Sustain. Energy Technol. Assess. 60, 103530. doi:10.1016/j.seta.2023.103530
Chen, H., Cong, T. N., Yang, W., Tan, C., Li, Y., and Ding, Y. (2009). Progress in electrical energy storage system: a critical review. Prog. Nat. Sci. 19, 291–312. doi:10.1016/j.pnsc.2008.07.014
Crenna, E., Secchi, M., Benini, L., and Sala, S. (2019). Global environmental impacts: data sources and methodological choices for calculating normalization factors for LCA. Int. J. Life Cycle Assess. 24, 1851–1877. doi:10.1007/s11367-019-01604-y
Dehghani-Sanij, A. R., Tharumalingam, E., Dusseault, M. B., and Fraser, R. (2019). Study of energy storage systems and environmental challenges of batteries. Renew. Sustain. Energy Rev. 104, 192–208. doi:10.1016/j.rser.2019.01.023
Deimede, V., and Elmasides, C. (2015). Separators for lithium-ion batteries: a review on the production processes and recent developments. Energy Technol. 3, 453–468. doi:10.1002/ente.201402215
Han, X., Li, Y., Nie, L., Huang, X., Deng, Y., Yan, J., et al. (2023). Comparative life cycle greenhouse gas emissions assessment of battery energy storage technologies for grid applications. J. Clean. Prod. 392, 136251. doi:10.1016/j.jclepro.2023.136251
Hao, H., Mu, Z., Jiang, S., Liu, Z., and Zhao, F. (2017). GHG emissions from the production of lithium-ion batteries for electric vehicles in China. Sustainability 9, 504. doi:10.3390/su9040504
ICCSD (2021). Understanding carbon neutrality: China’s low-carbon development roadmap for 2020-2050. 1 ed. Beijing: China CITIC Press.
Kersey, J., Popovich, N. D., and Phadke, A. A. (2022). Rapid battery cost declines accelerate the prospects of all-electric interregional container shipping. Nat. Energy 7, 664–674. doi:10.1038/s41560-022-01065-y
Li, J., and Ma, Z.-F. (2019). Past and present of LiFePO4: from fundamental research to industrial applications. Chem 5, 3–6. doi:10.1016/j.chempr.2018.12.012
Liang, Y., Su, J., Xi, B., Yu, Y., Ji, D., Sun, Y., et al. (2017). Life cycle assessment of lithium-ion batteries for greenhouse gas emissions. Resour. Conserv. Recycl. 117, 285–293. doi:10.1016/j.resconrec.2016.08.028
Liu, S. (2022). Comparative study on life cycle assessment of Li-ion power battery and pure electric vehicle (Master dissertation). Xi’an, China: Chang’an University.
Mahamud, R., and Park, C. (2022). Theory and practices of Li-ion battery thermal management for electric and hybrid electric vehicles. Energies 15, 3930. doi:10.3390/en15113930
Miller, I., Gençer, E., and O’Sullivan, F. (2018). A general model for estimating emissions from integrated power generation and energy storage. Case study: integration of solar photovoltaic power and wind power with batteries. Processes 6, 267. doi:10.3390/pr6120267
Mutel, C. (2017). Brightway: an open source framework for life cycle assessment. J. Open Source Softw. 2, 236. doi:10.21105/joss.00236
Nordelöf, A., Poulikidou, S., Chordia, M., Bitencourt De Oliveira, F., Tivander, J., and Arvidsson, R. (2019). Methodological approaches to end-of-life modelling in life cycle assessments of lithium-ion batteries. Batteries 5, 51. doi:10.3390/batteries5030051
Olabi, A. G., Onumaegbu, C., Wilberforce, T., Ramadan, M., Abdelkareem, M. A., and Al – Alami, A. H. (2021). Critical review of energy storage systems. Energy 214, 118987. doi:10.1016/j.energy.2020.118987
Oliveira, L., Messagie, M., Rangaraju, S., Sanfelix, J., Hernandez Rivas, M., and Van Mierlo, J. (2015). Key issues of lithium-ion batteries – from resource depletion to environmental performance indicators. J. Clean. Prod. 108, 354–362. doi:10.1016/j.jclepro.2015.06.021
Ouyang, M., Chu, Z., Lu, L., Li, J., Han, X., Feng, X., et al. (2015). Low temperature aging mechanism identification and lithium deposition in a large format lithium iron phosphate battery for different charge profiles. J. Power Sources 286, 309–320. doi:10.1016/j.jpowsour.2015.03.178
Rahman, M. M., Gemechu, E., Oni, A. O., and Kumar, A. (2021). The greenhouse gas emissions’ footprint and net energy ratio of utility-scale electro-chemical energy storage systems. Energy Convers. Manag. 244, 114497. doi:10.1016/j.enconman.2021.114497
Sadhukhan, J., and Christensen, M. (2021). An in-depth life cycle assessment (LCA) of lithium-ion battery for climate impact mitigation strategies. Energies 14, 5555. doi:10.3390/en14175555
Shen, S., Sadoughi, M., Chen, X., Hong, M., and Hu, C. (2019). A deep learning method for online capacity estimation of lithium-ion batteries. J. Energy Storage 25, 100817. doi:10.1016/j.est.2019.100817
Shu, X., Guo, Y., Yang, W., Wei, K., and Zhu, G. (2021). Life-cycle assessment of the environmental impact of the batteries used in pure electric passenger cars. Energy Rep. 7, 2302–2315. doi:10.1016/j.egyr.2021.04.038
Sullivan, J. L., and Gaines, L. (2010). A review of battery life-cycle analysis: state of knowledge and critical needs. (No. ANL/ESD/10-7). Argonne, IL (United States): Argonne National Lab. ANL. doi:10.2172/1000659
Tan, Y. (2017). Life cycle greenhouse gas impact analysis of battery energy storage systems in power system applications (Master dissertation). Nanjing, China: Nanjing University.
Wang, C., Chen, B., Yu, Y., Wang, Y., and Zhang, W. (2017). Carbon footprint analysis of lithium ion secondary battery industry: two case studies from China. J. Clean. Prod. 163, 241–251. doi:10.1016/j.jclepro.2016.02.057
Wang, M., Liu, K., Dutta, S., Alessi, D. S., Rinklebe, J., Ok, Y. S., et al. (2022). Recycling of lithium iron phosphate batteries: status, technologies, challenges, and prospects. Renew. Sustain. Energy Rev. 163, 112515. doi:10.1016/j.rser.2022.112515
Wernet, G., Bauer, C., Steubing, B., Reinhard, J., Moreno-Ruiz, E., and Weidema, B. (2016). The ecoinvent database version 3 (part I): overview and methodology. Int. J. Life Cycle Assess. 21, 1218–1230. doi:10.1007/s11367-016-1087-8
Yin, R., Hu, S., and Yang, Y. (2019). Life cycle inventories of the commonly used materials for lithium-ion batteries in China. J. Clean. Prod. 227, 960–971. doi:10.1016/j.jclepro.2019.04.186
Keywords: lithium iron phosphate, battery, energy storage, environmental impacts, emission reductions
Citation: Lin X, Meng W, Yu M, Yang Z, Luo Q, Rao Z, Zhang T and Cao Y (2024) Environmental impact analysis of lithium iron phosphate batteries for energy storage in China. Front. Energy Res. 12:1361720. doi: 10.3389/fenrg.2024.1361720
Received: 26 December 2023; Accepted: 07 February 2024;
Published: 28 February 2024.
Edited by:
Zhijie Wang, Hong Kong Polytechnic University, Hong Kong SAR, ChinaReviewed by:
Rudrodip Majumdar, National Institute of Advanced Studies, IndiaChenxu Wang, Washington State University Tri-Cities, United States
Copyright © 2024 Lin, Meng, Yu, Yang, Luo, Rao, Zhang and Cao. This is an open-access article distributed under the terms of the Creative Commons Attribution License (CC BY). The use, distribution or reproduction in other forums is permitted, provided the original author(s) and the copyright owner(s) are credited and that the original publication in this journal is cited, in accordance with accepted academic practice. No use, distribution or reproduction is permitted which does not comply with these terms.
*Correspondence: Wenchuan Meng, bWVuZ3djQGNzZy5jbg==; Yuwei Cao, eXdjYW9AaHVzdC5lZHUuY24=