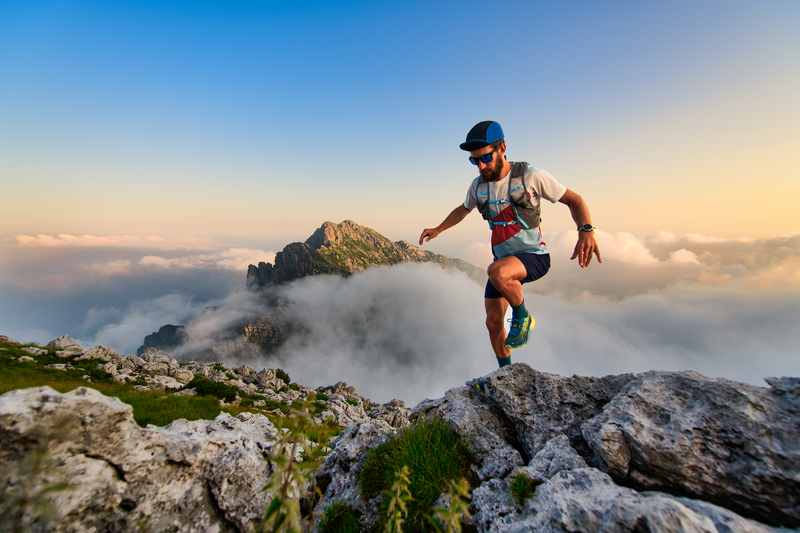
94% of researchers rate our articles as excellent or good
Learn more about the work of our research integrity team to safeguard the quality of each article we publish.
Find out more
REVIEW article
Front. Energy Res. , 08 February 2024
Sec. Sustainable Energy Systems
Volume 12 - 2024 | https://doi.org/10.3389/fenrg.2024.1358333
This article is part of the Research Topic Assessment of Renewable Energy Systems for Energy Conversion and Storage View all 4 articles
Concerns related to climate change have shifted global attention towards advanced, sustainable, and decarbonized energy systems. While renewable resources such as wind and solar energy offer environmentally friendly alternatives, their inherent variability and intermittency present significant challenges to grid stability and reliability. The integration of renewable energy sources requires innovative solutions to effectively balance supply and demand in the electricity grid. This review explores the critical role of electrolyzer systems in addressing these challenges by providing ancillary services to modern electricity grids. Electrolyzers traditionally used only for hydrogen production have now emerged as versatile tools capable of responding quickly to grid load variations. They can consume electricity during excess periods or when integrated with fuel cells generate electricity during peak demand, contributing to grid stability. Therefore, electrolyzer systems can fulfill the dual function of producing hydrogen for the end-user and offering grid balancing services, ensuring greater economic feasibility. This review paper aims to provide a comprehensive view of the electrolyzer systems’ role in the provision of ancillary services, including frequency control, voltage control, congestion management, and black start. The technical aspects, market, projects, challenges, and future prospects of using electrolyzers to provide ancillary services in modern energy systems are explored.
The global transition towards a sustainable and decarbonized energy landscape is leading to significant innovations in the grid energy systems sector. This transformation is primarily driven by the urgent need to mitigate climate change and reduce the environmental footprint of energy systems (International Energy Agency IEA, 2022). The integration of renewable energy sources, such as solar and wind, has gained considerable momentum due to their abundant availability and environmental benefits (International Energy Agency IEA, 2022). In 2022, global wind and solar installation continued dynamic growth, showing 75 GW of new wind capacity and 191 GW of new solar capacity, led by China, the US and the EU, contributing to a surge of global renewable energy production (Wind & Solar Share in Electricity Production Data | Enerdata, 2023). However, these renewable sources are characterized by their inherent variability and intermittency, presenting substantial challenges to grid stability and reliability (Worku, 2022).
To address the intermittency and variability of renewable energy sources, grid operators and energy planners are exploring innovative solutions that not only enhance grid stability but also facilitate the effective integration of renewables (Chakraborty et al., 2022). Electrolyzer systems, which have traditionally been used for hydrogen production (Cozzolino et al., 2021; Mendecka et al., 2021), have emerged as versatile tools capable of providing ancillary services to the grid (Dozein et al., 2021; 2023; Huang et al., 2023).
These services are essential to maintain the balance between electricity supply and demand, guarantee grid stability and optimize the use of renewable energy (Hossain et al., 2023a).
Ancillary services encompass a range of critical functions within an electrical grid, such as frequency control, voltage control, fast reactive current injections, inertia for local grid stability, short-circuit currents, and grid balancing (Rancilio et al., 2022). Traditionally, these services have been provided by conventional power plants, which are equipped with the capability to adjust their output rapidly in response to grid disturbances (Rancilio et al., 2022). However, the increasing penetration of renewable energy sources, with their variable generation patterns, has prompted a reevaluation of the grid’s reliance on conventional power plants for ancillary services (Banshwar et al., 2017).
This shift in the energy landscape has opened the door for alternative solutions, with electrolyzer-based systems at the forefront. Electrolyzers can function as flexible energy assets that respond rapidly to grid signals, either by consuming excess electricity during periods of oversupply or when integrated with fuel cells generating electricity during peak demand (Hossain et al., 2023b). Furthermore, electrolyzer systems can store surplus electricity by converting it into hydrogen through a process known as Power-to-Gas (PtG) (Fambri et al., 2022). This stored hydrogen can subsequently be converted back into electricity or used in various sectors, such as industry, transportation, and heating, offering a versatile approach to energy storage and grid support (Mirzaei et al., 2023).
The topic of ancillary services provided by electrolyzer systems is rather new and to date there is a gap in the literature as it is not possible to find complete reviews that explore this new research area. Therefore, this article aims to present a comprehensive review to explore the role of electrolyzer systems in providing ancillary services to modern power grids. Furthermore, by clarifying the multiple capabilities of electrolyzer systems in grid operations, this review aims to shed light on their potential to improve grid stability and facilitate the seamless integration of renewable energy sources into the modern energy landscape. The review covers various aspects, including electrolyzer technology, market, grid services, projects, challenges and future prospects. Each section analyzes specific aspects of electrolyzer-based systems, obviously providing insights into the potential of such systems in providing ancillary services, and the impact on grid operations.
Finally, this paper aims to fill the gap in the literature on the electrolyzer systems market by highlighting manufacturers and existing products, with a particular focus on large-scale systems suitable for applications such as grid-connected ancillary services.
The paper is organized as follows: in Section 2 the status and outlook for electrolyzers are presented with particular attention to technology, advances, and market; in Section 3 a detailed analysis of ancillary services provided by electrolyzer systems is carried out; in Section 4 some remarks on advantages and limitations are presented; in Section 5 a projects overview is taken into care; finally, in Section 6 some conclusions and future prospects are presented.
Hydrogen has emerged as a promising clean energy carrier, playing a pivotal role in the transition towards a more sustainable and low-carbon energy future. In this regard, hydrogen production methods are gaining considerable importance, with electrolysis standing out as a key technology for producing green hydrogen from renewable energy and for grid balancing and stabilizing. This section provides an overview of hydrogen electrolysis technologies, comparing their characteristics, advantages and disadvantages and current developments in which research is moving in order to optimize performance and costs, and increase lifetime. Furthermore, a perspective on the current international market scenario regarding manufacturers, commercially available large-scale products, and operational characteristics is proposed.
Hydrogen electrolysis is an electrochemical process that uses electrical energy to split water (H2O) into its constituent elements, hydrogen (H2) and oxygen (O2). This process is carried out in a device called an electrolyzer, which consists of two electrodes (anode and cathode) separated by an electrolyte. When an electrical current is passed through the water, hydrogen is generated at the cathode side, while oxygen is produced at the anode side.
Electrolysis technology encompasses three primary types of electrolyzers, Figure 1 - alkaline water electrolyzer (AWE), proton-exchange membrane electrolyzer (PEM), and solid oxide electrolyzer (SOE) - each with distinct characteristics, materials, and operating parameters offering unique advantages and applications Table 1.
FIGURE 1. Water electrolyzer type: (A) proton exchange membrane water electrolyzer (PEM), (B) alkaline water electrolyzer (AWE), (C) solid oxide water electrolyzer (SOE).
TABLE 1. Electrolyzer type specification (Shiva Kumar and Himabindu, 2019; López-Fernández et al., 2021; Nasser et al., 2022; Shiva Kumar and Lim, 2022).
PEM cell consists of an anode, and a cathode, aggregated with a solid polymer membrane electrolyte (such as Nafion). The water is fed to the anode side and through the anodic half-reaction is oxidized to O2, releasing H+ protons. The H+ protons then migrate across the membrane to the cathode side where are reduced to produce H2, Figure 1A PEM electrolyzers operate at temperatures between 50°C and 80°C and offer high current density (above 2 A cm−2), high efficiency, typically ranging from 50% to 83%, and a small footprint (Shiva Kumar and Himabindu, 2019). Their distinguishing feature is their rapid response time, on the order of milliseconds, which makes them ideal for applications requiring precise control and quick adjustments. PEM electrolyzers are commonly used in small to medium-scale applications and offer high hydrogen purity (Shiva Kumar and Himabindu, 2019). However, their durability is moderate (50,000 h), and the lifespan of the PEM membrane is a consideration (Nasser et al., 2022).
AWE cell has two compartments separated by a porous diaphragm (such as Zirfon) that allows OH− migration while preventing gas crossover. In this type of cell, H2O is reduced at the cathode to produce H2 and OH−, and the OH− ions migrate through the diaphragm to the anode where are then oxidized to O2, Figure 1B AWE electrolyzers commonly use KOH at very high concentrations (20%–40% KOH) as a liquid electrolyte (López-Fernández et al., 2021). They are known for their reliability and have a long history of industrial applications, especially for large-scale hydrogen production. Alkaline electrolyzers operate at temperatures between 70°C and 90°C and typically exhibit efficiency in the range of 50%–78% (Shiva Kumar and Lim, 2022). Their response time is moderate, measured in seconds, making them suitable for services that require relatively fast adjustments. They are highly scalable, capable of producing high-purity hydrogen, and with a long lifetime (60,000 h).
SOE cell is composed of two porous electrodes and a dense electrolyte layer generally made of zirconium (ZrO2) doped with yttrium (Y2O3), called YSZ (yttria-stabilized zirconia). Water (steam) fed to the cathode side is reduced to produce H2 and O2-, and the O2- ions migrate through the electrolyte (solid oxide) to the anode where are then oxidized to O2, Figure 1C SOE operate at much higher temperatures, typically between 700°C and 850°C (Hauch et al., 2020). They employ a solid oxide electrolyte and are recognized for their potential for high efficiency in the range of 89% (Shiva Kumar and Lim, 2022).While their response time is moderate, on the order of seconds, SOEC systems offer the unique advantage of co-producing syngas (a mixture of hydrogen and carbon monoxide) when operated in reverse mode. This capability can have additional economic benefits in applications such as power-to-gas and industrial processes. Solid oxide electrolyzers have a short lifetime (20,000 h) and find applications in large-scale industrial settings (Nasser et al., 2022).
In order to achieve significant technological advances, continuous research and development efforts have been made in the electrolyzer field area in recent years. These advancements are driven by the imperative to enhance the performance, efficiency, and cost-effectiveness of electrolyzer systems (Krishnan et al., 2023), making them increasingly competitive and viable for a wide range of applications.
• Advanced Catalysts: One of the most critical areas of advancement in electrolyzer technology revolves around the development of advanced catalysts for both the hydrogen evolution reaction (HER) and the oxygen evolution reaction (OER). These catalysts play a pivotal role in facilitating the electrochemical reactions within the electrolyzer, and their effectiveness directly impacts the system’s overall efficiency. Researchers have made substantial progress in designing and synthesizing catalyst materials with improved properties. These advanced catalysts exhibit higher catalytic activity, improved durability, and reduced overpotential, resulting in enhanced reaction kinetics within the electrolyzer cell (Ayers, 2021). At the moment, platinum (Pt) and ruthenium (Ru) based compounds are the most effective catalysts for HER and OER reactions respectively, but these are very expensive and rare precious metals (Hughes et al., 2019) therefore replace them with low-cost (Wu et al., 2021) and earth-abundant non-precious catalysts (Sun et al., 2018) seems to be the best solution. A wide range of earth-abundant catalysts has been identified, including metal sulfides and metal phosphides (Peng et al., 2018), carbon-based materials (Stelmachowski et al., 2021; Patella et al., 2023), metal alloys (Aralekallu et al., 2024), and chalcogenides (Su et al., 2023). Numerous studies have made great processes in improving the activity and stability by using new materials such as transition metal oxides, namely, NbC, SnO2, Ta2O5, TiO2, WC and TiC, and highly active metal alloys but the performance still fails to meet the requirement of practical electrolyzers (Meyer et al., 2015; Anwar et al., 2021; Wang et al., 2022). These novel alternatives, if proven valid, could not only reduce production costs, but will also contribute to the sustainability of electrolyzer technology.
• Novel Materials: Innovative materials research is another focal point of electrolyzer technology development. Advances in materials science have led to the exploration of novel materials for electrolyte membranes and bipolar plate components.
The introduction of novel membrane materials, such as polyphenylene oxide (PPO) blends, demonstrated enhanced proton conductivity and chemical stability, addressing some of the limitations associated with traditional polymer electrolyte membranes (PEMs) (Wang et al., 2023). Furthermore, the coated membranes with catalysts, such as Iron (Fe) and Nickel (Ni), and porous titanium (Ji et al., 2021), decrease the level of corrosion and increase the efficiency and durability (Xie et al., 2021).
Finally, research into innovative bipolar plate materials like graphitic foams and metal-matrix composites showcased improved corrosion resistance and electrical conductivity, critical for the long-term performance of electrolyzers (Luo et al., 2023).
• Enhanced System Integration: Improved system integration techniques, such as stack design, thermal management (Rashidi et al., 2022), and control strategies (Shen et al., 2019), are being developed to optimize overall electrolyzer performance. These advances aim to reduce energy losses, improve system reliability and reduce cost.
In recent years, the global electrolyzer market has grown rapidly and will continue with a remarkable growth rate, in fact, it is estimated to be worth $2.8 billion in 2022 and is expected to exceed $45.48 billion by 2032 with a recorded compound annual growth rate (CAGR) of 32.2% from 2023 to 2032 (Electrolyzer Market Size, 2023).
If take into account the already announced projects and their expected operational dates, the global electrolysis capacity installed could reach almost 3 GW by the end of 2023, a substantial increase considering that in 2022 the global installed capacity was 0.7 GW. Additionally, if all currently planned projects are realized, global electrolysis capacity could reach 170–365 GW by 2030 (International Energy Agency IEA, 2023a).
Global electrolyzer manufacturing capacity reached almost 14 GW per year in 2023, an increase of more than 35% compared to the previous year (International Energy Agency IEA, 2023a). Based on expectations of future growth in demand, many manufacturers have already started to expand their production capacity, and are constantly continuing research and development activities to improve system performance.
In particular, several companies have decided to take on the challenge in the competition to build the largest electrolyzer giga-factory, including John Cockerill (John Cockerill, 2023), Longi (LONGi, 2023), PERIC (Peric, 2023), Cummins (Cummins, 2023), ITM (ITM Power, 2023), Nel (Nel Hydrogen, 2023), McPhy (McPhy, 2023), Sungrow (Sungrow, 2023), Bloomenergy (Bloom Energy, 2023), Plug Power (Plug Power, 2023), Ohmium (Ohmium, 2023), Sunfire (Sunfire, 2023), Siemens (Siemens, 2023), ThyssenKrupp (Thyssenkrupp, 2023). Figure 2 shows the worldwide major electrolyzer giga-factory sites, highlighting current and planned capacities. It can be seen that the Europe, Middle East, and Africa (EMEA) region and the Asia-Pacific (APAC) region account for the majority of global manufacturing capacity, with approximately 40% and 45% respectively.
Commercially available products from different manufacturers for large-scale applications (>1 MW), with their characteristics such as, hydrogen production capacity, operating pressure, energy consumption and ramp-up time, are presented in Table 2. Currently, AWE electrolyzers represent the dominant technology with 75% of global production capacity, PEMs cover approximately 25% of manufacturing production, while SOEs represent a very small amount of early-stage production (Scottish Government, 2023).
Regarding electrolyzer capital expenditure costs (CAPEX) proposed by manufacturers, these are difficult to compare as information about the system scope or key parameters is often lacking. However, to date, CAPEX cost for an installed electrolyzer system is in the range 500–1,400 $/kWe for AWE, 1,100-1,800 $/kWe for PEM, while estimates for SOE range from 2,800-5,600 $/kWe (International Energy Agency IEA, 2023a).
From Table 2, it can be noted that data relating to the dynamic characteristics of the electrolyzers, such as load flexibility and response time to rapid load changes (ramp up/down), are missing or present only in a few models. This is due to the caution of manufacturers in providing data regarding these operating parameters because electrolyzer technology has not yet been fully explored, especially large-scale systems.
Load flexibility is the ability of the electrolyzer to operate over a wide range of loads. The rated load at which the system is designed defines the upper operating load (maximum load). Overloads above 100% are generally not expected for MW sizes, as the CAPEX would increase significantly for power electronics and balance of plant (BOP) design capable of operating at higher loads (above 100%).
Differently, the lower operating load (minimum load) is defined by the operating conditions under which the system can be used in order to ensure safe conditions. This is more limited in AWE electrolyzers - equipped with a diaphragm permeable to gases dissolved in the electrolyte - to avoid mixing of dissolved gases capable of triggering explosions.
In any case, by analyzing the literature experimental data on small-scale systems, it is possible to assume that the operating power range is 5%–100% and 20%–100% respectively for PEM and AWE (Bertuccioli et al., 2014; Kiaee et al., 2015; Stansberry et al., 2017; Martinez Lopez et al., 2023).
However, it is worth noting that recent developments by manufacturers are leading to a further reduction of the lower operating load to below 10% (You et al., 2017).
Response time to rapid load changes (ramp up/down) is a key characteristic that electrolyzers must ensure to provide grid services. Depending on the electrolyzer state, in start-up (environmental conditions), in operation, or in stand-by (at operating temperature and pressure), the response time takes on different values.
Normally, the response time in operating or stand-by conditions depends solely on the ability of the power electronics to react to set points, therefore the response time for both technologies is in the range of seconds. In particular, PEM technology has a ramp-up rate in the range 10%/s—80%/s and a ramp-down of −40%/s (Bertuccioli et al., 2014; Stansberry and Brouwer, 2020; Tuinema et al., 2020). Differently, AWE technology has a ramp-up rate in the range 0.3%/s - 17%/s, and a ramp-down rate of −25%/s (Bertuccioli et al., 2014; Gorre et al., 2020). In general, electrolyzers react faster to down ramps than up ramps. Otherwise, the response time when starting the system from cold to minimum load (start-up time) depends on the technology and size of the unit. Normally PEMs require start-up times in the order of 5 min, while AWEs in the order of 20 min (You et al., 2017). It remains obvious that MW size electrolyzers require more start-up time than kW-size units for both technologies.
Given their fast reaction rates, electrolyzers are very attractive for grid frequency support and could play a fundamental role in future energy systems characterized by the absence of rotational inertia (Alshehri et al., 2019). For this reason, the two technologies, AWE and PEM, have received approval for their use as ultrafast ancillary services (Eichman et al., 2014).
Ancillary services play a crucial role in maintaining the stability, reliability, and efficiency of modern electricity grids, especially as the integration of renewable energy sources (RES) continues to grow. These services encompass a range of functions, Figure 3, including frequency control, voltage control, congestion management, black start, loss compensation, and oscillation damping which are essential for balancing electricity supply with demand. In the context of increasing RES penetration, ancillary services become even more critical, as RES generation is inherently variable and may not align perfectly with consumer demand (International Energy Agency IEA, 2023c). Recent advancements in ancillary service provision have seen innovative technologies like grid-connected energy storage systems (Zakeri and Syri, 2015) and demand response programs (Kirschen Goran Strbac, 2018) contribute significantly to grid stability. These developments underscore the dynamic nature of ancillary services, which are adapting to the evolving energy landscape to ensure that the lights stay on, and power quality remains high (U.S. Federal Energy Regulatory Commission, 2023). As already anticipated in Section 2, electrolyzer-based energy storage systems given their high reaction rate have emerged in recent studies as versatile resources capable of providing ancillary services (Alshehri et al., 2019; Hossain et al., 2023b).
Frequency control is a fundamental operation in modern electricity grids to ensure their stability and reliability, especially given the growing integration of intermittent renewable energy sources (RES) such as wind and solar energy. Ancillary services, aimed at frequency control, play a fundamental role in maintaining the grid frequency within an acceptable range (typically 50 or 60 Hz ± 0.05 Hz), with an active power positive injection every time the frequency falls below the nominal level and vice versa with an active power negative injection if the frequency exceeds the set value.
The main ancillary services provided for frequency control can be observed in Figure 4 and described below:
• Frequency Containment reserves (FCR)/Primary frequency control: FCR is an automatic response and consists of the injection of active power via reserve generators to contain frequency deviation. It has an activation time of less than 30 s;
• Automatic Frequency Restoration Reserves (aFRR)/Secondary Frequency Control: aFRR consists of recovery reserves and active power which are capable of returning the frequency to the nominal level when a disturbance occurs in the system. It is activated automatically and has an activation time between 30 s and 15 min;
• Manual Frequency Restoration Reserves (mFRR)/Tertiary Frequency Control: mFRR consists of restoring primary and secondary frequency control reserves, and restoring both frequency and interchanges to their specified target values. It can be activated semi-automatically or manually and has a maximum activation time of 15 min;
• Replacement Reserves (RR): consists of restoring or sustaining the FRR to be prepared for any further imbalances in the system. It is activated semi-automatically or manually and has a minimum activation time of 15 min.
FIGURE 4. Restoration process guided by three reserve products (TUT, RSE, BME, FIN, and IBEX, 2020).
Electrolyzer-based systems for frequency control have gained prominence due to their dynamic behavior, and recent research has focused on optimizing and expanding their application in this domain (Alshehri et al., 2019; Johnsen et al., 2022; Dozein et al., 2023; Maluenda et al., 2023; Zheng et al., 2023).
Their ability to dynamically adjust their electricity consumption makes them valuable tools in grid management. When grid frequency deviates from its nominal value, electrolyzer systems can rapidly respond by modulating their power consumption or production (integrated with fuel cell). This involves either increasing hydrogen production (electrolysis) or shifting to power consumption (hydrogen fuel cell operation) within milliseconds, thereby injecting or absorbing power to stabilize grid frequency (Alshehri et al., 2019).
Eichman et al., in a NERL report have highlighted the advantages of using electrolyzers for frequency control due to the ultra-fast response time, which is often in the sub-second range (Eichman et al., 2014). In particular, the researchers developed a laboratory microgrid composed of a 120 kW diesel generator, a load simulator (i.e., a resistive load bank) and a 40 kW electrolyzer (PEM or AWE). The microgrid was tested as frequency disturbances varied, in particular by increasing the resistive load an under-frequency disturbance was generated, while decreasing the resistive load an over-frequency disturbance was generated. Experimental results have shown that when the electrolyzer (both PEM and AWE) participates in the microgrid by providing power (in case of over-frequency disturbance) or reducing power (in case of under-frequency disturbance), a ±0.2 Hz frequency restoration is achieved faster than with the diesel generator alone.
Such rapid response times are crucial in addressing sudden fluctuations in grid frequency caused by the intermittent nature of RES. Electrolyzer-based systems for frequency control can enhance grid stability and reliability by quickly counteracting frequency deviations, preventing cascading failures, and minimizing the risk of blackouts (Alhelou et al., 2019).
Furthermore, the scalability of electrolyzer systems makes them adaptable to various grid sizes and configurations. Smaller-scale systems can be deployed at distribution levels, where RES integration is increasing, to provide localized frequency control. In this regard, Mohanpurkar et al. presented a real-time simulation to verify the frequency grid support with a 120 kW electrolyzer driven by a generic front-end controller (FEC). The results showed that the electrolyzer can provide sub-second response and the frequency deviation was reduced (Mohanpurkar et al., 2017).
Conversely, larger-scale electrolyzer installations can be strategically placed at transmission or substation levels to support broader grid stability and enhance overall frequency control capabilities (Suárez et al., 2018; Samani et al., 2020; Tuinema et al., 2020).
In a case study Alshehri et al. (Alshehri et al., 2019) evaluated the effectiveness of PEM electrolyzers and fuel cells for the provision of primary frequency reserves based on realistic projections of the Northern Netherlands grid for the year 2030. The results obtained showed that hydrogen PEM technologies can improve frequency response compared to synchronous generators with the same reserve value. In a subsequent analysis by Tuinema et al. (Tuinema et al., 2020) on the same case study, a model for use a large electrolyzer in real-time simulations on a digital simulator was developed. The model was validated with experimental measurements of a 1 MW plant installed in the northern part of the Netherlands, and was scaled up to simulate a large 300 MW plant. Several real-time simulations were conducted to study the frequency support from electrolyzers to the northern part of the Dutch transmission grid. The results highlighted an electrolyzer positive effect on frequency stability after the loss of generating or load capacity, because can respond faster to frequency deviations than conventional generators.
Samani et al. (2020) evaluated the provision of primary reserve (FCR) via a large 25 MW electrolyzer for the Belgian grid transmission. The revenues from the FCR reserve were analyzed according to a techno-economic model, and the optimal economic strategy resulting from the analysis was to run the electrolyzer at a base load of 55% while providing the remaining capacity as a power reserve. Furthermore, the study results showed that the fast response of the PEM electrolyzer provides a high degree of flexibility, and therefore offers the opportunity to participate in the ancillary market.
Recent case studies have demonstrated the feasibility and effectiveness of electrolyzer-based systems for frequency control in real-world grid scenarios. These studies often involve the deployment of projects or the integration of electrolyzer systems into existing grid infrastructure. Matute et al. (2019) developed a technical-economic model of a multi-MW electrolyzer to provide grid services and obtain a more accurate assessment of the feasibility of business case related to the hydrogen supply for different uses, such as fuel cell electric vehicle (FCEV) operators and hydrogen refueling stations (HRS) in Spanish territory. The results showed that grid services contribute to the profitability of hydrogen production for mobility. In particular, the contribution from the provision of secondary frequency control for a 5 MW electrolyzer is around 10% and the minimum demand to obtain non-negative business cases should be above 1000 FCEV.
The value that electrolyzer systems can guarantee from an economic point of view by providing frequency control services has been the subject of recent studies. Johnsen et al. (2022) conducted a value analysis of an electrolyzer in Denmark using a mixed-integer linear optimization model, which allows the electrolyzer to make informed bidding decisions in the FCR and FRR reserve markets. The results showed that an electrolyzer in the Danish bidding zone DK1 could have earned 57% more in 2021 by providing ancillary services.
Zheng et al. (2023) proposed a comprehensive operational model to enable an economic evaluation of a PtG project from the perspective of its net present value and break-even price to show the effectiveness of the provision of reserves in the Danish market in increasing profitability. The results revealed that providing FCR and FRR services increase efficiently the yearly contribution margin, raise the project net present value (NPV) and reduce the hydrogen break-even price.
Scolaro and Kittner (2022) investigated whether participation in the German ancillary services market is cost-competitive for 150 MW offshore wind based on 131 MW hydrogen production electrolyzer system. Leveraging empirical data on the European energy market and wind generation, the results showed that offshore wind-based hydrogen must participate in ancillary services markets to generate positive net revenues at current wind generation levels to become competitive in terms of costs in Germany.
Dadkhah et al. (2022) examined with a probabilistic mixed integer linear programming model the technical-economic characteristics of providing frequency grid services by hydrogen refueling stations (HRS). The results indicated that by participating in the ancillary service markets it is possible to significantly increase the economic profit, while ensuring the stable performance of the HRS.
Recent advancements in control strategies and grid integration have further improved the effectiveness of electrolyzer-based systems for frequency control. Advanced control algorithms, such as model predictive control (MPC) and adaptive control, are being developed to optimize the response of electrolyzer systems to grid frequency deviations (Lee and Kim, 2022; Lee et al., 2023). These algorithms enable precise adjustments of electrolyzer operation, ensuring that they provide the necessary support without compromising their primary function, such as hydrogen production for energy storage or industrial applications.
However, challenges remain in the widespread adoption of electrolyzer-based systems for frequency control. The capital costs associated with the installation and maintenance of electrolysis systems represent a significant barrier and therefore considerable efforts are being made to reduce costs in the short and long term (IRENA, 2020).
Furthermore, the development of regulatory frameworks that incentivize the participation of electrolyzer-based businesses in frequency control markets is essential (ENTSO-E, 2022; Ringsgwandl et al., 2022). These challenges require collaborative efforts by governments, grid operators and the private sector to create an enabling environment for electrolyzer-based systems for ancillary services.
Voltage control is a critical aspect of maintaining the stability and reliability of modern electrical grids, particularly in the context of increasing renewable energy integration (Ku et al., 2020).
Historically, this ancillary service was provided by conventional synchronous generators (Stanelyte and Radziukynas, 2019), however, in recent years, the importance of using electrolyzers to support voltage regulation is attracting much attention.
Electrolyzer systems, with their inherent ability to modulate reactive power, could play a vital role in voltage control (Torres et al., 2019). Reactive power is essential for regulating voltage levels within acceptable limits, especially in the presence of intermittent renewable resources like wind and solar power. Electrolyzers can dynamically absorb or inject (if coupled with a fuel cell) reactive power, thereby contributing to grid voltage stability (Torres et al., 2019). When grid voltage deviates from the desired range, electrolyzers respond swiftly to produce hydrogen through the electrolysis process thus actively adjusting the flow of reactive power to stabilize the voltage.
One noteworthy advantage of utilizing electrolyzers for voltage control is their rapid response time (Eichman et al., 2014). Electrolyzers can adjust their operation within milliseconds, making them an ideal choice for managing transient voltage deviations (Eichman et al., 2014). In contrast, conventional solutions like synchronous condensers or static var compensators may require several seconds to react to voltage changes, which could be insufficient to prevent voltage instability in fast-changing grid conditions (Teleke et al., 2008; Lepour et al., 2021).
Furthermore, the integration of electrolyzers into the grid for voltage control aligns with the broader goal of achieving a sustainable and resilient energy system (Cai et al., 2022). As renewable energy sources continue to grow, the demand for effective and environmentally friendly solutions for grid management becomes more pressing. Electrolyzers, particularly when powered by renewable energy, enable grid operators to balance voltage levels while reducing greenhouse gas emissions.
Although in literature there are not yet many studies on the subject, some recent studies have demonstrated the advantages of using systems based on electrolyzers for voltage control (Chiesa et al., 2011; Ghosh et al., 2020; Pijarski and Kacejko, 2021).
Chiesa et al. (2011) demonstrated the feasibility of using an electrolyzer for improving voltage quality. The analyzed system consists of an alkaline electrolyzer coupled with a wind system and both connected to the grid. In order to obtain realistic fluctuations in active and reactive power, a stochastic model was developed for the wind turbine, while for the electrolyzer a model was developed that takes efficiency and dynamic response into account. The results obtained showed that the implemented operational strategy is capable of dynamically controlling the energy consumption of the electrolyzer in such a way as to minimize grid voltage fluctuations.
In the study conducted by Ghosh et al. (2020), an operational model of a fleet of grid-integrated electrolyzers has been developed, to minimize the impact of the increasing penetration of solar PV into the distribution network. To quantify operational improvements, the study includes performance parameters related to distribution voltage profiles. The results highlighted that the electrolyzer fleet control application can help reduce overvoltage, voltage fluctuations and operation of control devices caused by intermittent solar generation.
Pijarski and Kacejko (2021) proposed an innovative voltage control system in the MV grid, in which it was hypothesized that the loads represented by electrolyzers were used to produce “green hydrogen” for power-to-gas purposes. Analyses conducted with hundreds of calculation cycles using Monte Carlo simulation have shown that the subordination of the hydrogen production process to the voltage control objectives in the MV grid clearly contributes to stabilizing the voltage value and guaranteeing its quality, while meeting hydrogen production requirements.
In order to maximize the benefits of using electrolyzers for voltage control and reactive power management, grid operators must integrate advanced control and monitoring systems. These systems can enable real-time monitoring of grid conditions and the rapid adjustment of electrolyzer operations to respond to voltage fluctuations. Predictive algorithms and intelligent control strategies can optimize the deployment of electrolyzers for both voltage control and energy storage, ensuring that these systems operate efficiently and effectively.
Jain et al. (2023) evaluated the use of electrolyzers in a PtG scenario for grid voltage support, using real-time power-hardware-in-the-loop (PHIL) testing with a 225 kW PEM electrolyzer stack. The authors modelled electrolyzers as controllable loads and the voltage enhancement with electrolyzer support is analysed for DER penetration levels of 0%, 25%, and 50%. The results showed that the electrolyzer controls met the performance requirements for voltage control by providing a reduction of up to 112 Load Tap Changers (LTC) operations on the distribution grid, and provided substantial improvements in network operating conditions.
An electrochemical model of a 500 kW alkaline electrolyzer considering nonlinearity and dynamics, integrated with a phase-shifted full-bridge active front-end converter, was presented by Zhao et al. (2023). In order to study the harmonic distortion and system response during voltage deviation in the grid distribution, the electrolyzer power system was tested with MATLAB®/Simulink® and PLECS under different grid conditions. The simulation results showed that the designed system complied with the harmonic requirements of the Danish grid code for electricity storage and responded well to both 10% and 20% voltage deviation dips.
In the study of Jakobsen et al. (2023), grid code considerations that must be applied when connecting large scale electrolyzer plant to the electrical grid are addressed. In particular, a model for an electrolyzer based on a grid-connected three-phase h-bridge converter with a low-voltage ride-through strategy was developed. The results highlight the capabilities of the electrolysis system to provide reactive power support to the grid during voltage drops.
Grid balancing, is a fundamental aspect to guarantee the stability, reliability and resilience of the electricity grid, especially because the penetration of intermittent renewable energy sources, such as wind and solar energy, continues to grow strongly (Mendecka et al., 2020; Zsiborács et al., 2021).
In this context, electrolyzers have emerged as versatile ancillary services that play a pivotal role in addressing the challenges associated with grid balancing and energy storage (Stamatakis et al., 2022).
Electrolyzer systems are well-suited for grid balancing due to their remarkable flexibility in adjusting electricity consumption or production (when integrated with fuel cell systems) in response to grid conditions (Buttler and Spliethoff, 2018). Grid balancing involves matching electricity supply with demand in real-time, a task made increasingly complex by the intermittent nature of RES. Electrolyzers-fuel cell integrated systems’ bidirectional capability enables to dynamically support grid balancing by absorbing or injecting power, alleviating grid congestion, and mitigating supply-demand imbalances.
Recent case studies have demonstrated the feasibility and effectiveness of electrolyzer-based grid balancing and energy storage in practical grid scenarios.
Gusain et al. (2020) conducted a technical analysis to evaluate the ability of a large-scale PEM electrolyzer to provide grid balancing services. Three case studies were defined to evaluate the electrolyzer in different operating conditions and the impact on the grid then the electrolyzer was evaluated. The results showed how the electrolyzer is particularly suitable for providing grid balancing services and due to its efficient and safe operation at partial load, it can be used to correct forecast errors of the wind during the day. Finally, a drop in efficiency was demonstrated for the case in which the cell operated for a whole year, concluding that to avoid an incorrect flexibility assessment it is important to take into account the drop in efficiency in the long-term strategy of balancing service models.
Zenith et al. (2022) quantified the value of grid services for hydrogen production units in providing alternative income to wind-powered hydrogen-producing electrolyzers. Grid services from several countries such as France, Italy, Norway, and Spain, were simulated with each country’s energy price data and wind energy profiles from wind farms. The results showed that the economics of electrolyzers can be improved through network services that can monetize the unused capacity of hydrogen production facilities. In fact, for 3 countries such as Italy, Spain, and France, in conditions of “upregulation”, a curtailed value of hydrogen higher than 6 €/kg is obtained, more than 3 times higher than the EU price target for 2030.
To evaluate the effectiveness of using electrolyzers to provide grid-forming services, Tavakoli et al. (2023) conducted numerical analyses on a low-inertia test grid inspired by a portion of the transmission grid in southern Australia. In addition to the grid-forming mode, to ensure the safe operation of the electrolyzer in case of adverse interaction between the grid-forming operation and hydrogen production constraints, the authors developed two other additional operating modes, such as the DC voltage and constant power modes, respectively. The results highlighted the great potential of the electrolyzer to provide grid-forming services, furthermore with a nearby wind generation system, a grid-forming electrolyzer can form the voltage and frequency of an islanded grid only if the hydrogen production constraints are not violated, otherwise the operating mode of the electrolyzer switches to DC voltage mode or constant power mode.
Dadkhah et al. (2021) developed a model to evaluate the technical-economic feasibility of a hydrogen refueling station (HRS), whose electrolyzer is powered by energy purchased from the electricity market, and also participates in the auxiliary services market. The scenarios investigated, 2020 and 2030, highlighted how the electrolyzer participation in the reserve market increases revenues up to 16% and decreases the hydrogen break-even price up to 4.7% and 6.4%, in 2020 and 2030, respectively.
In the study conducted by Allidières et al. (2019), the objective was to characterize and qualify PEM electrolyzers for grid service balancing purposes. The authors first analyzed the management constraints of the European electricity grid, subsequently designed the specific load profiles for the management of the electricity grid, and finally used these protocols for the electrolyzers characterization. The results demonstrated that electrolyzers can be used for such applications and also can satisfy the most stringent grid constraints.
Raab et al. (2022) developed a model to economically evaluate hydrogen production plants with grid participation, to minimize the specific costs of each site for a constant hydrogen supply. The analysis highlighted that grid participation means that the hydrogen produced will not be 100% renewable, but it is more advantageous from a technical and economic point of view with production costs for the five locations analyzed ranging between 4 -6 €/kg, and even more ecological.
Wu et al. (2022) conducted a techno-economic evaluation for a hydrogen production and storage system considering different energy delivery pathways and various grid services. The developed model takes into account the operational capacity, flexibility, and constraints associated with the hydrogen system. The analysis highlights that for a hydrogen system to be financially sustainable and advantageous it must also include grid services, whose value streams resulting from the bundling of network services could account for up to 76% of the overall benefits.
As already mentioned, the increase in renewable energy production and its grid integration, aggregated with population growth and therefore the increase in energy consumption, represent the main factors contributing to the problem of grid congestion.
Grid congestion refers to the condition where the electricity demand exceeds the capacity of the power grid, leading to inefficiencies, potential outages, and increased stress on the system. This issue arises when the electricity generation and transmission infrastructure cannot keep up with the growing power demand. To address grid congestion, various strategies are being explored, including investments in grid expansion and modernization, the development of smart grid technologies (Romero-Ruiz et al., 2016), and the implementation of energy storage systems to enhance grid flexibility (Tarashandeh and Karimi, 2021). Policy initiatives, smart network tariffs (Hennig et al., 2023), and the integration of advanced analytics also play crucial roles in mitigating grid congestion and ensuring a reliable and resilient power supply.
In literature, several studies evaluated new methods aiming the managing congestion (Yusoff et al., 2017; Bouloumpasis et al., 2019; Gumpu et al., 2019; Hu et al., 2021), but still few studies have examined the role of electrolyzers in providing support to congestion management.
Dowling and Jansen of the TNO research organization in the study (Dowling and Jansen, 2023) examined congestion management using an electrolyzer system in 4 use-cases, such as, renewable generation, industrial end-user, energy hub, and grid operator, evaluating the legal and market aspects and the impact of congestion problems. The analysis showed that the presence of a local hydrogen user ensures the electrolyzer’s economic viability, and that the greatest expected impact on congestion occurs when the electrolyzer is owned and operated by the grid operator. Furthermore, it was found that the business case and the impact on congestion do not necessarily go hand in hand and the cost-effective operational strategy of electrolyzers can hurt the congestion problem.
Ghaemi et al. (2023) developed an economic model applied to a north Netherlands region to evaluate the feasibility of an electrolysis system dedicated to hydrogen production, to reduce congestion in medium voltage distribution grids integrated with a high rate of renewable sources and price-conscious consumers. An incentive-based single-leader and multiple-follower method to improve flexibility in such grids has been adopted. In particular, the method provides that the leader (grid operator) determines the dynamic congestion prices for each grid node every time, and in turn, the followers (producers and/or consumers) adapt their electricity production or consumption to maximize one’s objective function (i.e., minimize costs). The investigation showed that electrolyzers operate profitably when faced with dynamic congestion pricing and high renewable generation volumes. These results provide useful information on grid incentives potential (i.e., dynamic pricing) that can motivate companies to use electrolyzers to provide flexibility to grid operators to resolve congestion problems.
Lieberwirth and Hobbie (2023) evaluated the electrolyzers ability that support decarbonization in the German industrial sector, in contributing to the efficient management of electricity networks and network congestion. An electricity market and congestion management optimization model was developed and applied to different scenarios that differ in the electrolyzers operating mode, the industrial sector decarbonization strategy, and the electrolyzers penetration rate into the electricity grid.
The analysis highlighted the effectiveness of integrating electrolyzers into congestion management practices, especially if industries are decentralized and more distributed throughout Germany, such as the chemical, paper, and printing industries. Differently in scenarios with electrolyzers with flexible operation for congestion management, the total congestion management volume decreases proportionally to the electrolyzers market penetration regardless of the territorial distribution of electrolyzers.
The last focus of this section is the problem of grid black-start, which refers to the challenge of restoring an electricity grid after a total or partial blackout, often caused by events such as natural disasters or cyber-attacks, so that the affected areas can recover energy more quickly.
It involves starting isolated production units and gradually reconnecting them to form an interconnected system again. It is a critical resource for the safe, reliable and resilient operation of electric power systems and for effectively restoring electricity. Traditionally this process is based on conventional generators (Sun et al., 2011), which, despite being consolidated technologies, have the disadvantage of being heavily dependent on fossil energy sources. For this reason, battery energy storage systems (BESS) (Fohrmann, 2021; Pagnani et al., 2023) and renewable energy systems (Pagnam et al., 2020; Sam, 2021), have been explored as viable alternatives that could play an important role in the context of black-start operations. These innovative solutions not only improve grid reliability, but also align with broader goals of transitioning to cleaner and more sustainable energy systems.
Otherwise, although still little investigated, the integration of hydrogen energy systems (e.g., electrolyzers and fuel cells) in black-start procedures could represent a further step forward toward improving the overall resilience and environmental sustainability of electricity infrastructures (Jacobsen, 2022; Han et al., 2023; Mtu, 2023).
As highlighted in the previous section, the ancillary services provided by electrolyzer systems have become increasingly popular in recent years due to electrolyzers’ ability to respond quickly to load fluctuations and assist in grid balancing, thereby increasing the grid capacity to accommodate higher levels of renewable energy and reduce dependence on conventional fossil fuel.
The integration of electrolyzer systems into the grid to provide ancillary services offers several notable advantages:
• Versatility: Electrolyzer systems are versatile and adaptable, capable of providing a range of ancillary services such as frequency control, voltage control, congestion management, and black starts. This versatility makes them valuable assets in modern grid operations, where flexibility is of utmost importance;
• Scalability: Electrolyzer systems can be scaled to meet specific grid requirements. This scalability allows grid operators to deploy the appropriate size of electrolyzer systems based on the local grid’s needs, from small-scale deployments to large utility-scale installations;
• Emissions Reduction: Ancillary services provided by electrolysis systems promote greenhouse gas emissions reduction. By using excess renewable energy to produce hydrogen, they facilitate the decarbonization of the energy sector, thereby contributing to environmental sustainability.
While the potential benefits are promising, the deployment of ancillary services provided by electrolyzer-based systems faces several challenges and limitations:
• Capital Costs: The upfront capital costs associated with installing electrolyzers can be significant. Although these costs have decreased over time due to technological advances and economies of scale (IRENA, 2020), they remain a significant barrier to widespread adoption;
• Hydrogen Infrastructure: Building a reliable and comprehensive hydrogen infrastructure, including storage, transport and distribution, is crucial for the efficient operation of electrolyzer systems. Building this infrastructure is a complex and expensive undertaking (Kim et al., 2023);
• Grid Integration: Effectively integrating electrolyzer systems into the grid and developing robust control strategies is a technical challenge. Coordination with existing grid assets, such as power plants and transmission lines, is critical to ensure seamless operation;
• Round-trip efficiency: The hydrogen produced must be compressed, transported, and stored, and when energy is needed again, the hydrogen can be consumed by the fuel cell system for energy production. Each of these processes involves energy losses, and to date, the round-trip efficiency is quite low and at most reaches 40% (Escamilla et al., 2022).
Addressing these challenges is essential to realizing the full potential of electrolyzer-based ancillary services. Ongoing research and development efforts, supportive policies, and collaborations between stakeholders are crucial in overcoming these obstacles and enabling the widespread adoption of this technology.
According to IEA report (International Energy Agency IEA, 2023b), there are about 2,550 hydrogen production projects through electrolysis worldwide, some of which are completed and operational, some are in the development phase, and some are pure demonstration projects. However, only 0.6% of these focused on demonstrating the feasibility and advantages of integrating electrolysis systems into grid operations to ensure balancing and frequency control services.
In the last decade, greater emphasis has been placed on these aspects and private and community-funded projects and initiatives have been launched to provide valuable information for practical application and to test and demonstrate the effectiveness of on-site electrolyzer-based ancillary services. To the authors’ knowledge, there are 15 completed or ongoing projects worldwide, Table 3.
The electrolyzer technology used is divided equally between AWE and PEM with MW-scale sizes, with the exception of the DOE (Department of Energy) and QualyGridS projects (R&D), where the kW laboratory-scale is examined. In most projects the electrolyzers are powered by renewable energy, such as wind and solar, and the end-users considered are industrial, PtG, mobility, residential and power.
As shown in Figure 5, only one project is from the USA, while all others are located in Europe, where Denmark and Germany are leaders or are involved in most European projects. Furthermore, it is highlighted that in 63% of cases, grid balancing services are provided via electrolyzers, while in the remaining 37% of cases, frequency control services are provided.
Among the projects of greatest interest and already operational are highlighted:
• P2G-BioCat, Denmark 2014-2017, (P2G-BioCat, 2017; Rasmussen, 2021), - A project with a total cost of 6.7 million euros to demonstrate a power-to-gas plant based on a 1 MW alkaline electrolyzer powered by excess wind energy to produce renewable gas for storage in the Danish natural gas network. The BioCat consortium facility is located at the Avedøre wastewater treatment plant in Copenhagen and has been approved by Energinet to participate in the DK2 grid market of Eastern Denmark to provide frequency control (FCR, FRR) and power regulation services.
• Demo4Grid, Austria 2017-2023, (Demo4Grid, 2017a; Demo4Grid, 2017b), - A project with a total cost of 7.7 million euros with the aim of demonstrating the feasibility of providing grid balancing services with a 3.2 MW pressurized alkaline electrolyzer, while producing green hydrogen for both the MPREIS food production site in Austria, and for the refueling of fuel cell trucks. The installation on the MPREIS site took place in 2021, and the subsequent demonstration period highlighted that to achieve greater economic value and revenues from the management strategy it is necessary to combine multiple business cases, such as the simultaneous provision of hydrogen for industry and mobility, and the participation in grid balancing services.
• HyBalance, Denmark 2015-2020, (HyBalance, 2016), - A project with a total cost of 15.8 million euros based on a 1.2 MW PEM electrolyzer powered by renewable energy. The facility has been approved by Energinet to participate in the Western Danish DK1 network market and, thanks to dynamic operation, is capable of providing frequency regulation services (FCR, FRR). The green hydrogen produced is used for PtG and mobility purposes. The project managed to demonstrate the feasibility and flexibility of PEM electrolyzers, with the plant capable of producing 99.998% pure hydrogen, while participating in the Danish electricity market with frequency control services with a shorter response time of 10 s.
• H2FUTURE, Austria 2017-2021, (H2FUTURE/Technology, 2017), - A project coordinated by the energy supplier VERBUND with a total cost of 17.8 million euros with the aim of producing green hydrogen for the VOESTALPINE steelworks in Linz using a 6 MW PEM electrolysis system from Siemens. In addition, with the support of the transmission system operator (TSO) Austrian Power Grid (APG), the system was qualified to provide grid balancing services as a primary, secondary or tertiary reserve. In the first 2 years of demonstration testing, the H2FUTURE electrolyzer produced more than 500 tons of green hydrogen, equivalent to 8,800 tons of “green steel.” The project manager sees expansion on a large industrial scale as a future challenge in order to increase hydrogen production in the longer term to the 340,000 tons of hydrogen that the steelworks requires at full capacity.
• REFHYNE, Germany 2018-2024, (REFHYNE, 2018; REFHYNE/Cordis, 2018), - A project with a total cost of 19.7 million euros to install a 10 MW electrolyzer from ITM Power at a large refinery in Rhineland, Germany, operated by Shell Deutschland Oils. The electrolyzer delivers large quantities of hydrogen to refinery processes to replace existing methane reformers. Through its dynamic operation, it helps balance the refinery’s internal electricity network and also sells the primary control power to the German transmission system operator (TSO). The 2-year technical-economic analysis showed that the combination of hydrogen supply to the refinery and grid balancing payments creates an economically advantageous business case that justifies the initial investment. The project results were sufficiently promising and led to the new REFHYNE 2 project.
• REFHYNE 2 Germany 2021-2026, (REFHYNE II, 2021; REFHYNE II/Cordis, 2021), - A project with a total cost of 149 million euros, which aims to extend the initial REFYHNE project by building a 100 MW PEM electrolysis site powered by a mix of dedicated renewable and grid electricity, again with the same purpose of supplying hydrogen to the Rhineland refinery in Germany.
These projects demonstrate the diverse applications of electrolyzers providing ancillary services in different regions and grid scenarios. They highlight the ability of electrolyzers systems to improve grid stability, store excess renewable energy and contribute to decarbonization efforts. As these projects continue to evolve, they provide valuable insights for future implementations and highlight the growing importance of electrolyzer technology in modern grid operations.
In the pursuit of a robust and sustainable energy future, the use of innovative energy systems to provide ancillary services in modern power grids represents an important and evolving paradigm. Due to their dynamic behavior, electrolyzers are adaptive systems that can bridge the gap between intermittent renewable energy sources and grid stability. Their dual ability to produce green hydrogen while providing grid services puts them at the center of today’s evolving energy landscape.
In this review article, the main features of the ancillary services provided by electrolyzer systems such as technology, market, grid services, benefits and projects have been highlighted.
From the literature analyzed, excellent results could be observed in various numerical studies carried out to assess the electrolyzer capabilities in providing services for frequency control (FCR, FRR), voltage control or to manage grid congestion or black starts. On the other hand, few experimental studies and projects have been started in this research area.
The projects already in operation are all European and lie between Austria, Denmark and Germany. In these projects electrolyzers are used to produce hydrogen for industrial or mobility end-users and at the same time provide frequency control (FCR, FRR) or grid balancing, which demonstrates better economic feasibility when merging the two case businesses.
Several future development key areas show the great potential that electrolyzer systems can have to improve and positively influence grid operations and facilitate the integration of renewable energy sources:
The prospects of electrolyzer technology are expected to be significantly influenced by its continuous improvement. The performance of electrolysis systems will be further enhanced by advances in membrane technologies, catalytic materials, and system integration, which will increase their attractiveness for grid ap-plications. Longer system lifespan, lower capital costs, and higher efficiency are expected results of the research efforts.
Market mechanisms and funding policies will be crucial for the future development of electrolyzer-based ancillary services. Governments and regulators are increasingly recognizing the importance of grid flexibility and de-carbonization. Feed-in tariffs, CO2 pricing mechanisms and incentive programs can promote investments in electrolyzers and facilitate their integration into grid operations.
The growth of the hydrogen economy will be closely linked to the expansion of ancillary services based on electrolyzers. As with some projects, hydrogen has become an increasingly important energy source for various sectors, including transport, industry, and heating, and electrolyzers have demonstrated a dual role in supporting grid stability and contributing to hydrogen production. This dual function will certainly increase the economic viability of using electrolyzers.
With the increasing decentralization of energy production through distributed renewable sources, the role of electrolyzer systems in local grid stabilization and microgrid applications will increase. Electrolyzers can serve as flexible energy resources in microgrids, contributing to local resilience and reducing dependence on core grid infrastructure.
In conclusion, it is possible to state that while the world is transitioning towards a more sustainable and resilient energy system, systems based on electrolyzers are increasingly cutting edge, offering solutions capable of guaranteeing decarbonization objectives. Their continued growth and integration into the power grid mark a significant step towards achieving a cleaner, more reliable and environmentally sustainable energy future.
RC: Conceptualization, Data curation, Methodology, Resources, Writing–original draft, Writing–review and editing. GB: Methodology, Supervision, Visualization, Writing–review and editing.
The author(s) declare that no financial support was received for the research, authorship, and/or publication of this article.
The authors declare that the research was conducted in the absence of any commercial or financial relationships that could be construed as a potential conflict of interest.
All claims expressed in this article are solely those of the authors and do not necessarily represent those of their affiliated organizations, or those of the publisher, the editors and the reviewers. Any product that may be evaluated in this article, or claim that may be made by its manufacturer, is not guaranteed or endorsed by the publisher.
Alhelou, H. H., Hamedani-Golshan, M. E., Njenda, T. C., and Siano, P. (2019). A survey on power system blackout and cascading events: research motivations and challenges. Energies 12, 1–28. doi:10.3390/en12040682
Allidières, L., Brisse, A., Millet, P., Valentin, S., and Zeller, M. (2019). On the ability of pem water electrolysers to provide power grid services. Int. J. Hydrogen Energy 44, 9690–9700. doi:10.1016/J.IJHYDENE.2018.11.186
Alshehri, F., Suárez, V. G., Rueda Torres, J. L., Perilla, A., and van der Meijden, M. A. M. M. (2019). Modelling and evaluation of PEM hydrogen technologies for frequency ancillary services in future multi-energy sustainable power systems. Heliyon 5, e01396. doi:10.1016/J.HELIYON.2019.E01396
Angstrom Advanced (2023). Angstrom advanced. Available at: https://www.angstrom-advanced.com/pro6.html (Accessed October 6, 2023).
Anwar, S., Khan, F., Zhang, Y., and Djire, A. (2021). Recent development in electrocatalysts for hydrogen production through water electrolysis. Int. J. Hydrogen Energy 46, 32284–32317. doi:10.1016/J.IJHYDENE.2021.06.191
Aralekallu, S., Sannegowda Lokesh, K., and Singh, V. (2024). Advanced bifunctional catalysts for energy production by electrolysis of earth-abundant water. Fuel 357, 129753. doi:10.1016/J.FUEL.2023.129753
AUYAN (2023). AUYAN. Available at: https://www.auyangloble.com/hydrogen-production/Hydrogen-Production-Electrolyzer.html#TECHNICALDATA (Accessed November 10, 2023).
Ayers, K. (2021). High efficiency PEM water electrolysis: enabled by advanced catalysts, membranes, and processes. Curr. Opin. Chem. Eng. 33, 100719. doi:10.1016/J.COCHE.2021.100719
Banshwar, A., Sharma, N. K., Sood, Y. R., and Shrivastava, R. (2017). Renewable energy sources as a new participant in ancillary service markets. Energy Strateg. Rev. 18, 106–120. doi:10.1016/j.esr.2017.09.009
Bertuccioli, L., Chan, A., Hart, D., Lehner, F., Madden, B., and Standen, E. (2014). Development of water electrolysis in the European union.
Bloom Energy (2023). Bloom energy. Available at: https://www.bloomenergy.com/resource/electrolyzer-spec-sheet/ (Accessed November 13, 2023).
Bouloumpasis, I., Steen, D., and Tuan, L. A. (2019). “Congestion management using local flexibility markets: recent development and challenges,” in Proc. 2019 IEEE PES Innov. Smart Grid Technol. Eur. ISGT-Europe, Bucharest, Romania, September 29 - October 2, 2019. doi:10.1109/ISGTEUROPE.2019.8905489
Buttler, A., and Spliethoff, H. (2018). Current status of water electrolysis for energy storage, grid balancing and sector coupling via power-to-gas and power-to-liquids: a review. Renew. Sustain. Energy Rev. 82, 2440–2454. doi:10.1016/J.RSER.2017.09.003
Cai, S., Xie, Y., Wu, Q., Zhang, M., Jin, X., and Xiang, Z. (2022). Active and reactive power coordinated two-stage MG scheduling for resilient distribution systems under uncertainties. IEEE Trans. Smart Grid 13, 2986–2998. doi:10.1109/TSG.2022.3149816
Chakraborty, M. R., Dawn, S., Saha, P. K., Basu, J. B., and Ustun, T. S. (2022). A comparative review on energy storage systems and their application in deregulated systems. Batteries 8, 124. doi:10.3390/batteries8090124
Chiesa, N., Korpås, M., Kongstein, O. E., and Ødegård, A. (2011). Dynamic control of an electrolyser for voltage quality enhancement. Int. Conf. Power Syst. Transients.
Cozzolino, R., Chiappini, D., and Tribioli, L. (2021). Off-grid PV/URFC power plant fueled with biogas from food waste: an energetic and economic analysis. Energy 219, 119537. doi:10.1016/j.energy.2020.119537
Cummins (2023). Cummins. Available at: https://www.cummins.com (Accessed October 6, 2023).
Dadkhah, A., Bozalakov, D., De Kooning, J. D. M., and Vandevelde, L. (2021). On the optimal planning of a hydrogen refuelling station participating in the electricity and balancing markets. Int. J. Hydrogen Energy 46, 1488–1500. doi:10.1016/J.IJHYDENE.2020.10.130
Dadkhah, A., Bozalakov, D., De Kooning, J. D. M., and Vandevelde, L. (2022). Techno-economic analysis and optimal operation of a hydrogen refueling station providing frequency ancillary services. IEEE Trans. Ind. Appl. 58, 5171–5183. doi:10.1109/TIA.2022.3167377
Demo4Grid (2017a). Demo4Grid. Available at: https://cordis.europa.eu/project/id/736351 (Accessed November 21, 2023).
Demo4Grid (2017b). Demo4Grid. Available at: https://www.demo4grid.eu/ (Accessed November 21, 2023).
Dowling, R., and Jansen, N. (2023). Potential cases for electrolysis as solution for grid congestion TNO 2023 R10556.
Dozein, M. G., De Corato, A. M., and Mancarella, P. (2023). Virtual inertia response and frequency control ancillary services from hydrogen electrolyzers. IEEE Trans. Power Syst. 38, 2447–2459. doi:10.1109/TPWRS.2022.3181853
Dozein, M. G., Jalali, A., and Mancarella, P. (2021). Fast frequency response from utility-scale hydrogen electrolyzers. IEEE Trans. Sustain. Energy 12, 1707–1717. doi:10.1109/TSTE.2021.3063245
Eichman, J., Harrison, K., and Peters, M. (2014). Novel electrolyzer applications: providing more than just hydrogen novel electrolyzer applications: providing more than just hydrogen. Available at: http://www.nrel.gov/docs/fy14osti/61758.pdf.
Electrolyzer Market Size (2023). Electrolyzer market size. Available at: https://www.precedenceresearch.com/electrolyzer-market (Accessed October 6, 2023).
Elogen (2023). Our-products/usine-delectrolyse-en. Available at: https://elogenh2.com/en/our-products/usine-delectrolyse-en/ (Accessed October 6, 2023).
ELYGRID (2014). ELYGRID. Available at: https://cordis.europa.eu/project/id/278824/reporting (Accessed November 21, 2023).
ELYNTEGRATION (2019). ELYNTEGRATION. Available at: https://elyntegration.eu/ (Accessed November 20, 2023).
ELYntegration/Cordis (2019). ELYntegration/Cordis. Available at: https://cordis.europa.eu/project/id/671458 (Accessed November 21, 2023).
ENTSO-E (2022). New ENTSO-E paper on the role of hydrogen - facts about system integration. Available at: https://www.entsoe.eu/2021/11/29/entso-e-publishes-new-paper-the-role-of-hydrogen-facts-about-system-integration/.
Escamilla, A., Sánchez, D., and García-Rodríguez, L. (2022). Assessment of power-to-power renewable energy storage based on the smart integration of hydrogen and micro gas turbine technologies. Int. J. Hydrogen Energy 47, 17505–17525. doi:10.1016/J.IJHYDENE.2022.03.238
Fambri, G., Diaz-Londono, C., Mazza, A., Badami, M., Sihvonen, T., and Weiss, R. (2022). Techno-economic analysis of Power-to-Gas plants in a gas and electricity distribution network system with high renewable energy penetration. Appl. Energy 312, 118743. doi:10.1016/J.APENERGY.2022.118743
Fohrmann, T. (2021). Battery energy storage systems for black start capability | Energy Central. Available at: https://energycentral.com/o/siemens-energy/battery-energy-storage-systems-black-start-capability (Accessed November 20, 2023).
Ghaemi, S., Li, X., and Mulder, M. (2023). Economic feasibility of green hydrogen in providing flexibility to medium-voltage distribution grids in the presence of local-heat systems. Appl. Energy 331, 120408. doi:10.1016/J.APENERGY.2022.120408
Ghosh, S., Jain, R., Veda, S., and Terlip, D. (2020). “Improving distribution system operations using fleet control of electrolyzers,” in Proc. IEEE Power Eng. Soc. Transm. Distrib. Conf, Washington DC, October 2020. doi:10.1109/TD39804.2020.9300018
Gorre, J., Ruoss, F., Karjunen, H., Schaffert, J., and Tynjälä, T. (2020). Cost benefits of optimizing hydrogen storage and methanation capacities for Power-to-Gas plants in dynamic operation. Appl. Energy 257, 113967. doi:10.1016/J.APENERGY.2019.113967
Green Hydrogen (2023). Green hydrogen. Available at: https://www.greenhydrogensystems.com/#electrolysers (Accessed October 6, 2023).
Greenhyscale (2021). Greenhyscale. Available at: https://greenhyscale.eu/the-project-info/ (Accessed November 21, 2023).
GreenHyScale/Cordis (2021). GreenHyScale/cordis. Available at: https://cordis.europa.eu/project/id/101036935 (Accessed November 21, 2023).
Gumpu, S., Pamulaparthy, B., and Sharma, A. (2019). Review of congestion management methods from conventional to smart grid scenario. Int. J. Emerg. Electr. Power Syst. 20. doi:10.1515/ijeeps-2018-0265
Gusain, D., Cvetkovic, M., Bentvelsen, R., and Palensky, P. (2020). “Technical assessment of large scale PEM electrolyzers as flexibility service providers,” in IEEE Int. Symp. Ind. Electron, Delft, The Netherlands, June 2020, 1074–1078. doi:10.1109/ISIE45063.2020.9152462
H2FUTURE (2017). H2FUTURE. Available at: https://www.h2future-project.eu/ (Accessed November 21, 2023).
H2FUTURE/Technology (2017). H2FUTURE/Technology. Available at: https://www.h2future-project.eu/technology (Accessed November 20, 2023).
Haeolus (2018). Haeolus. Available at: https://www.haeolus.eu/?p=1143 (Accessed November 20, 2023).
Haeolus/ Cordis (2018). Haeolus/Cordis. Available at: https://cordis.europa.eu/project/id/779469/it (Accessed November 21, 2023).
Han, J., Wang, J., He, Z., An, Q., Song, Y., Mujeeb, A., et al. (2023). Hydrogen-powered smart grid resilience. Energy Convers. Econ. 4, 89–104. doi:10.1049/ENC2.12083
Hauch, A., Küngas, R., Blennow, P., Hansen, A. B., Hansen, J. B., Mathiesen, B. V., et al. (2020). Recent advances in solid oxide cell technology for electrolysis. Science 80, eaba6118. doi:10.1126/science.aba6118
Hennig, R. J., de Vries, L. J., and Tindemans, S. H. (2023). Congestion management in electricity distribution networks: smart tariffs, local markets and direct control. Util. Policy 85, 101660. doi:10.1016/J.JUP.2023.101660
Hossain, M. A., Islam, M. R., Hossain, M. A., and Hossain, M. J. (2023a). Control strategy review for hydrogen-renewable energy power system. J. Energy Storage 72, 108170. doi:10.1016/j.est.2023.108170
Hossain, M. B., Islam, M. R., Muttaqi, K. M., Sutanto, D., and Agalgaonkar, A. P. (2023b). Advancement of fuel cells and electrolyzers technologies and their applications to renewable-rich power grids. J. Energy Storage 62, 106842. doi:10.1016/J.EST.2023.106842
Hovsapian, R. (2017). Role of electrolyzers in grid services. DOE Hydrog. Progr. 2021 AMR Proc., 34. Available at: https://www.hydrogen.energy.gov/pdfs/htac_may17_08_hovsapian.pdf.
HPEM2GAS (2016). HPEM2GAS. Available at: https://hpem2gas.eu/ (Accessed November 20, 2023).
HPEM2GAS/ Cordis (2016). HPEM2GAS/Cordis. Available at: https://cordis.europa.eu/project/id/700008 (Accessed November 21, 2023).
H-TEC (2023). H-Tec.Com/En/Products. Available at: https://www.h-tec.com/en/products/# (Accessed October 6, 2023).
Hu, J., Liu, X., Shahidehpour, M., and Xia, S. (2021). Optimal operation of energy hubs with large-scale distributed energy resources for distribution network congestion management. IEEE Trans. Sustain. Energy 12, 1755–1765. doi:10.1109/TSTE.2021.3064375
Huang, C., Zong, Y., You, S., and Traholt, C. (2023). Analytical modeling and control of grid-scale alkaline electrolyzer plant for frequency support in wind-dominated electricity-hydrogen systems. IEEE Trans. Sustain. Energy 14, 217–232. doi:10.1109/TSTE.2022.3208361
Hughes, J. P., Blanco, F. D., Banks, C. E., and Rowley-Neale, S. J. (2019). Mass-producible 2D-WS2 bulk modified screen printed electrodes towards the hydrogen evolution reaction. RSC Adv. 9, 25003–25011. doi:10.1039/C9RA05342E
HyBalance (2016). HyBalance. Available at: https://stateofgreen.com/en/solutions/power-to-x-the-hybalance-project/ (Accessed November 20, 2023).
HyBalance/ Cordis (2016). HyBalance/Cordis. Available at: https://cordis.europa.eu/project/id/671384 (Accessed November 23, 2023).
HydrogenPro (2023). HydrogenPro. Available at: https://hydrogen-pro.com/ (Accessed November 10, 2023).
HyScaling (2021). HyScaling. Available at: https://ispt.eu/projects/hyscaling-establishing-a-dutch-electrolyzer-industry/ (Accessed November 20, 2023).
Hystar (2023). Hystar. Available at: https://hystar.com/products/ (Accessed November 13, 2023).
International Energy Agency (IEA) (2022). Renewables 2022, analysis forecast to 2027. Available at: https://www.iea.org/reports/renewables-2022;License:CCBY4.0.
International Energy Agency (IEA) (2023a). Electrolysers - energy system. Available at: https://www.iea.org/energy-system/low-emission-fuels/electrolysers (Accessed October 5, 2023).
International Energy Agency (IEA) (2023b). Hydrogen production and infrastructure projects database - data product - IEA. Available at: https://www.iea.org/data-and-statistics/data-product/hydrogen-production-and-infrastructure-projects-database#hydrogen-production-projects (Accessed November 22, 2023).
International Energy Agency (IEA) (2023c). Renewable energy market update: outlook for 2023 and 2024, 87. Available at: www.iea.org/t&c/.
ITM Power (2023). ITM power. Available at: https://itm-power.com/products/ (Accessed October 6, 2023).
Jacobsen, B. (2022). Green flexibility Market - platform development for system services using fuel cells. Procedia CIRP 105, 158–163. doi:10.1016/j.procir.2022.02.027
Jain, R., Nagasawa, K., Veda, S., and Sprik, S. (2023). Grid ancillary services using electrolyzer-Based power-to-Gas systems with increasing renewable penetration. e-Prime - Adv. Electr. Eng. Electron. Energy 6, 100308. doi:10.1016/J.PRIME.2023.100308
Jakobsen, R., Huang, C., Rasmussen, T. W., and You, S. (2023). Large-scale electrolyzer plant integration to the electrical grid: preliminary investigation of VSC-based solutions. Energy Rep. 9, 478–483. doi:10.1016/J.EGYR.2023.09.061
Ji, W., Wang, S., Sun, Y., Lv, H., Shen, X., and Zhang, C. (2021). Research on the influence of collector microstructure on the performance of PEM electrolyzer. World Electr. Veh. J. 12, 165. Page 165 12. doi:10.3390/WEVJ12040165
John, C. (2023). Hydrogen johncockerill. Available at: https://hydrogen.johncockerill.com/en/products/ (Accessed October 6, 2023).
Johnsen, A. G., Mitidatri, L., Zarrilli, D., and Kazempour, J. (2022). The value of ancillary services for electrolyzers.
Kasei, A. (2023). Asahi Kasei starts construction of alkaline water electrolysis pilot test plant for hydrogen production. Available at: https://www.asahi-kasei.com/news/2022/e221107.html (Accessed October 6, 2023).
Kiaee, M., Cruden, A., Chladek, P., and Infield, D. (2015). Demonstration of the operation and performance of a pressurised alkaline electrolyser operating in the hydrogen fuelling station in Porsgrunn, Norway. Energy Convers. Manag. 94, 40–50. doi:10.1016/J.ENCONMAN.2015.01.070
Kim, C., Cho, S. H., Cho, S. M., Na, Y., Kim, S., and Kim, D. K. (2023). Review of hydrogen infrastructure: the current status and roll-out strategy. Int. J. Hydrogen Energy 48, 1701–1716. doi:10.1016/J.IJHYDENE.2022.10.053
Kirschen Goran Strbac, D. S. (2018). Fundamentals of power system economics. 2. Available at: https://www.wiley.com/en-cn/Fundamentals+of+Power+System+Economics%2C+2nd+Edition-p-9781119213253 (Accessed October 10, 2023).
Krishnan, S., Koning, V., Theodorus de Groot, M., de Groot, A., Mendoza, P. G., Junginger, M., et al. (2023). Present and future cost of alkaline and PEM electrolyser stacks. Int. J. Hydrogen Energy 48, 32313–32330. doi:10.1016/J.IJHYDENE.2023.05.031
Ku, T. T., Lin, C. H., Hsu, C. T., Chen, C. S., Liao, Z. Y., Wang, S.De, et al. (2020). Enhancement of power system operation by renewable ancillary service. IEEE Trans. Ind. Appl. 56, 6150–6157. doi:10.1109/TIA.2020.3020782
Lee, G. H., and Kim, Y. J. (2022). Frequency regulation of an islanded microgrid using hydrogen energy storage systems: a data-driven control approach. Energies 15, 8887. doi:10.3390/EN15238887
Lee, G. H., Park, J. Y., Ban, J., Kim, Y. J., and Catalao, J. P. S. (2023). Data-driven modeling and optimal control of hydrogen energy storage for frequency regulation. IEEE Trans. Energy Convers. 38, 1231–1245. doi:10.1109/TEC.2022.3221165
Lepour, D., Paolone, M., Denis, G., Cardozo, C., Prevost, T., and Guiu, E. (2021). “Performance assessment of synchronous condensers vs voltage source converters providing grid-forming functions,” in 2021 IEEE Madrid PowerTech, PowerTech 2021 - Conf. Proc., Piscataway NJ USA, July 2021, 1–6. doi:10.1109/PowerTech46648.2021.9494883
Lieberwirth, M., and Hobbie, H. (2023). Decarbonizing the industry sector and its effect on electricity transmission grid operation—implications from a model based analysis for Germany. J. Clean. Prod. 402, 136757. doi:10.1016/J.JCLEPRO.2023.136757
LONGi (2023). Products/Hydrogen/Lhy-A1000. Available at: https://www.longi.com/en/products/hydrogen/lhy-a1000/ (Accessed October 6, 2023).
López-Fernández, E., Sacedón, C. G., Gil-Rostra, J., Yubero, F., González-Elipe, A. R., and de Lucas-Consuegra, A. (2021). Recent advances in alkaline exchange membrane water electrolysis and electrode manufacturing. Mol 26, 6326. doi:10.3390/MOLECULES26216326
Luo, X., Ren, C., Song, J., Luo, H., Xiao, K., Zhang, D., et al. (2023). Design and fabrication of bipolar plates for PEM water electrolyser. J. Mater. Sci. Technol. 146, 19–41. doi:10.1016/J.JMST.2022.10.039
Maluenda, M., Córdova, S., Lorca, Á., and Negrete-Pincetic, M. (2023). Optimal operation scheduling of a PV-BESS-Electrolyzer system for hydrogen production and frequency regulation. Appl. Energy 344, 121243. doi:10.1016/j.apenergy.2023.121243
Martinez Lopez, V. A., Ziar, H., Haverkort, J. W., Zeman, M., and Isabella, O. (2023). Dynamic operation of water electrolyzers: a review for applications in photovoltaic systems integration. Renew. Sustain. Energy Rev. 182, 113407. doi:10.1016/J.RSER.2023.113407
Matute, G., Yusta, J. M., and Correas, L. C. (2019). Techno-economic modelling of water electrolysers in the range of several MW to provide grid services while generating hydrogen for different applications: a case study in Spain applied to mobility with FCEVs. Int. J. Hydrogen Energy 44, 17431–17442. doi:10.1016/J.IJHYDENE.2019.05.092
McPhy (2023). Apparecchiature-E-servizi/elettrolizzatori/large. Available at: https://mcphy.com/it/apparecchiature-e-servizi/elettrolizzatori/large/ (Accessed October 6, 2023).
Mendecka, B., Chiappini, D., Tribioli, L., and Cozzolino, R. (2021). A biogas-solar based hybrid off-grid power plant with multiple storages for United States commercial buildings. Renew. Energy 179, 705–722. doi:10.1016/J.RENENE.2021.07.078
Mendecka, B., Tribioli, L., and Cozzolino, R. (2020). Life Cycle Assessment of a stand-alone solar-based polygeneration power plant for a commercial building in different climate zones. Renew. Energy 154, 1132–1143. doi:10.1016/j.renene.2020.03.063
Meyer, S., Nikiforov, A. V., Petrushina, I. M., Köhler, K., Christensen, E., Jensen, J. O., et al. (2015). Transition metal carbides (WC, Mo2C, TaC, NbC) as potential electrocatalysts for the hydrogen evolution reaction (HER) at medium temperatures. Int. J. Hydrogen Energy 40, 2905–2911. doi:10.1016/j.ijhydene.2014.12.076
Mirzaei, M. A., Habibi, M., Vahidinasab, V., and Mohammadi-Ivatloo, B. (2023). Integration of power-to-gas (P2G) technologies in Operation of integrated gas-electricity networks. Power-To-gas Bridg. Electr. Gas. Netw., 77–95. doi:10.1016/B978-0-323-90544-2.00012-9
Mohanpurkar, M., Luo, Y., Terlip, D., Dias, F., Harrison, K., Eichman, J., et al. (2017). Electrolyzers enhancing flexibility in electric grids. Energies 10, 1836. doi:10.3390/EN10111836
Mtu (2023). Pressreleases/2023/Rolls-Royce-Successfully-Tests-Mtu-Fuel-Cell-System-For-Blackout. Available at: https://www.mtu-solutions.com/eu/en/pressreleases/2023/rolls-royce-successfully-tests-mtu-fuel-cell-system-for-blackout.html (Accessed December 19, 2023).
Nasser, M., Megahed, T. F., Ookawara, S., and Hassan, H. (2022). A review of water electrolysis–based systems for hydrogen production using hybrid/solar/wind energy systems. Environ. Sci. Pollut. Res. Int. 29, 86994–87018. doi:10.1007/S11356-022-23323-Y
Nel Hydrogen (2023). Nel hydrogen. Available at: https://nelhydrogen.com/water-electrolysers-hydrogen-generators/ (Accessed October 6, 2023).
NEXT Hydrogen (2023). NEXT hydrogen. Available at: https://nexthydrogen.com/solutions/products/ (Accessed October 6, 2023).
Ohmium (2023). Ohmium. Available at: https://www.ohmium.com/our-product (Accessed October 6, 2023).
P2G-BioCat (2017). P2G-BioCat. Available at: https://energiforskning.dk/en/node/15072 (Accessed November 21, 2023).
Pagnam, D., Kocewiak, L. H., Hjerrild, J., Blaabjerg, F., and Bak, C. L. (2020). “Overview of black start provision by offshore wind farms,” in IECON Proc. Industrial Electron. Conf, Singapore, October 2020, 1892–1898. doi:10.1109/IECON43393.2020.9254743
Pagnani, D., Kocewiak, L., Hjerrild, J., Blaabjerg, F., Bak, C. L., Blasco-Gimenez, R., et al. (2023). Power system restoration services by grid-forming offshore wind farms with integrated energy storage. IEEE Power Energy Soc. Gen. Meet. doi:10.1109/PESGM52003.2023.10253374
Patella, B., Zanca, C., Ganci, F., Carbone, S., Bonafede, F., Aiello, G., et al. (2023). Pd–Co-Based electrodes for hydrogen production by water splitting in acidic media. Mater 16, 474. doi:10.3390/MA16020474
Peng, L., Shah, S. S. A., and Wei, Z. (2018). Recent developments in metal phosphide and sulfide electrocatalysts for oxygen evolution reaction. Chin. J. Catal. 39, 1575–1593. doi:10.1016/S1872-2067(18)63130-4
Peric (2023). Alkaline-type-hydrogen-G. Available at: http://www.peric718.com/Alkaline-Type-Hydrogen-G/r-85.html (Accessed November 10, 2023).
Pijarski, P., and Kacejko, P. (2021). Voltage optimization in MV network with distributed generation using power consumption control in electrolysis installations. Energies 14, 993. doi:10.3390/EN14040993
Plug Power (2023). Plug power. Available at: https://www.plugpower.com/hydrogen/electrolyzer-hydrogen/electrolyzer-products/ (Accessed October 6, 2023).
Power-2-Electrolysis (2016). Power-2-Electrolysis. Available at: https://eudp.dk/en/node/15053 (Accessed November 20, 2023).
Proton OnSite (2023). Proton OnSite. Available at: https://www.protononsite.com/sites/default/files/2016-10/pd-0600-0115_rev_a%281%29.pdf (Accessed October 6, 2023).
QualyGridS (2017). Proyectos/Qualygrids_En. Available at: https://hidrogenoaragon.org/en/proyectos/qualygrids_en/ (Accessed November 20, 2023).
QualyGridS/ Cordis (2017). QualyGridS/Cordis. Available at: https://cordis.europa.eu/project/id/735485 (Accessed November 21, 2023).
Raab, M., Körner, R., and Dietrich, R. U. (2022). Techno-economic assessment of renewable hydrogen production and the influence of grid participation. Int. J. Hydrogen Energy 47, 26798–26811. doi:10.1016/J.IJHYDENE.2022.06.038
Rancilio, G., Rossi, A., Falabretti, D., Galliani, A., and Merlo, M. (2022). Ancillary services markets in europe: evolution and regulatory trade-offs. Renew. Sustain. Energy Rev. 154, 111850. doi:10.1016/J.RSER.2021.111850
Rashidi, S., Karimi, N., Sunden, B., Kim, K. C., Olabi, A. G., and Mahian, O. (2022). Progress and challenges on the thermal management of electrochemical energy conversion and storage technologies: fuel cells, electrolysers, and supercapacitors. Prog. Energy Combust. Sci. 88, 100966. doi:10.1016/J.PECS.2021.100966
Rasmussen, L. H. (2021). Centrica ’ s activities on hydrogen Examples from Denmark PtX flexibility for the electricity markets.
REFHYNE (2018). REFHYNE. Available at: https://www.refhyne.eu/ (Accessed November 21, 2023).
REFHYNE/ Cordis (2018). REFHYNE/Cordis. Available at: https://cordis.europa.eu/project/id/779579 (Accessed November 21, 2023).
REFHYNE II (2021). REFHYNE II. Available at: https://www.refhyne.eu/refhyne-2/ (Accessed November 21, 2023).
REFHYNE II/ Cordis (2021). REFHYNE II/Cordis. Available at: https://cordis.europa.eu/project/id/101036970 (Accessed November 21, 2023).
Ringsgwandl, L. M., Schaffert, J., Brücken, N., Albus, R., and Görner, K. (2022). Current legislative framework for green hydrogen production by electrolysis plants in Germany. Energies 15, 1786. doi:10.3390/EN15051786
Romero-Ruiz, J., Pérez-Ruiz, J., Martin, S., Aguado, J. A., and De La Torre, S. (2016). Probabilistic congestion management using EVs in a smart grid with intermittent renewable generation. Electr. Power Syst. Res. 137, 155–162. doi:10.1016/J.EPSR.2016.03.015
Sam, S. (2021). Black-start using renewable energy resources. IEEE smart grid. Available at: https://smartgrid.ieee.org/bulletins/april-2021/black-start-using-renewable-energy-resources (Accessed November 20, 2023).
Samani, A. E., D’Amicis, A., de Kooning, J. D. M., Bozalakov, D., Silva, P., and Vandevelde, L. (2020). Grid balancing with a large-scale electrolyser providing primary reserve. IET Renew. Power Gener. 14, 3070–3078. doi:10.1049/IET-RPG.2020.0453
Scolaro, M., and Kittner, N. (2022). Optimizing hybrid offshore wind farms for cost-competitive hydrogen production in Germany. Int. J. Hydrogen Energy 47, 6478–6493. doi:10.1016/J.IJHYDENE.2021.12.062
Scottish Government (2023). Existing supply chains, capabilities and growth. Dir. Communities 1, 1–51. Available at: http://www.gov.scot/publications/assessment-electrolysers-report/pages/4/ (Accessed January 11, 2024).
Shen, X., Zhang, X., Lv, H., Li, G., and Lie, T. T. (2019). Structure design and control strategy of a new alkaline water electrolyzer based on heat exchange. Int. J. Energy Res. 43, 4729–4742. doi:10.1002/ER.4612
Shiva Kumar, S., and Himabindu, V. (2019). Hydrogen production by PEM water electrolysis – a review. Mater. Sci. Energy Technol. 2, 442–454. doi:10.1016/J.MSET.2019.03.002
Shiva Kumar, S., and Lim, H. (2022). An overview of water electrolysis technologies for green hydrogen production. Energy Rep. 8, 13793–13813. doi:10.1016/J.EGYR.2022.10.127
Siemens (2023). Products-services/energy/renewable-energy/hydrogen-solutions/silyzer. Available at: https://new.siemens.com/content/dam/internet/siemens-com/global/products-services/energy/renewable-energy/hydrogen-solutions/silyzer/ (Accessed October 6, 2023).
Stamatakis, E., Perwög, E., Garyfallos, E., Millán, M. S., Zoulias, E., and Chalkiadakis, N. (2022). Hydrogen in grid balancing: the European market potential for pressurized alkaline electrolyzers. Energies 15, 637. doi:10.3390/EN15020637
Stanelyte, D., and Radziukynas, V. (2019). Review of voltage and reactive power control algorithms in electrical distribution networks. Energies 13, 58. doi:10.3390/EN13010058
Stansberry, J., Hormaza Mejia, A., Zhao, L., and Brouwer, J. (2017). Experimental analysis of photovoltaic integration with a proton exchange membrane electrolysis system for power-to-gas. Int. J. Hydrogen Energy 42, 30569–30583. doi:10.1016/J.IJHYDENE.2017.10.170
Stansberry, J. M., and Brouwer, J. (2020). Experimental dynamic dispatch of a 60 kW proton exchange membrane electrolyzer in power-to-gas application. Int. J. Hydrogen Energy 45, 9305–9316. doi:10.1016/J.IJHYDENE.2020.01.228
Stelmachowski, P., Duch, J., Sebastián, D., Lázaro, M. J., and Kotarba, A. (2021). Carbon-based composites as electrocatalysts for oxygen evolution reaction in alkaline media. Mater 14, 4984. doi:10.3390/MA14174984
Su, H., Jiang, J., Song, S., An, B., Li, N., Gao, Y., et al. (2023). Recent progress on design and applications of transition metal chalcogenide-associated electrocatalysts for the overall water splitting. Chin. J. Catal. 44, 7–49. doi:10.1016/S1872-2067(22)64149-4
Suárez, V. G., Rueda, J. L., Tuinema, B., Guerra, A. P., and Meijden, M. van der (2018). Integration of power-to-gas conversion into Dutch electrical ancillary services markets. 1–7. ENERGY Econ. Technol., Available at: https://research.tudelft.nl/en/publications/integration-of-power-to-gas-conversion-into-dutch-electrical-anci (Accessed October 20, 2023).
Sun, W., Liu, C. C., and Liu, S. (2011). Black start capability assessment in power system restoration. IEEE Power Energy Soc. Gen. Meet. doi:10.1109/PES.2011.6039752
Sun, X., Xu, K., Fleischer, C., Liu, X., Grandcolas, M., Strandbakke, R., et al. (2018). Earth-abundant electrocatalysts in proton exchange membrane electrolyzers. Catal 8, 657. doi:10.3390/CATAL8120657
Sunfire (2023). Sunfire hydrogen. Available at: https://www.sunfire.de/en/hydrogen (Accessed October 6, 2023).
Sungrow (2023). Sungrow pem-water-electrolysis-equipment-pem-water-electrolysis-equipment. Available at: https://en.sungrowpower.com/productDetail/3482/pem-water-electrolysis-equipment-pem-water-electrolysis-equipment# (Accessed November 10, 2023).
Tarashandeh, N., and Karimi, A. (2021). Utilization of energy storage systems in congestion management of transmission networks with incentive-based approach for investors. J. Energy Storage 33, 102034. doi:10.1016/J.EST.2020.102034
Tavakoli, S. D., Dozein, M. G., Lacerda, V. A., Mane, M. C., Prieto-Araujo, E., Mancarella, P., et al. (2023). Grid-forming services from hydrogen electrolyzers. IEEE Trans. Sustain. Energy 14, 2205–2219. doi:10.1109/TSTE.2023.3270295
Teleke, S., Abdulahovic, T., Thiringer, T., and Svensson, J. (2008). Dynamic performance comparison of synchronous condenser and SVC. IEEE Trans. Power Deliv. 23, 1606–1612. doi:10.1109/TPWRD.2007.916109
Thyssenkrupp (2023). UCPthyssenkruppBAISUhdeChlorineEngineers. Available at: https://d2zo35mdb530wx.cloudfront.net/_legacy/UCPthyssenkruppBAISUhdeChlorineEngineers/assets.files/products/water_electrolysis/tk_19_0820_hydrogen_broschuere_2019_03.pdf (Accessed October 6, 2023).
Topsoe (2023). Knowledge/our-products/Equipment/soec. Available at: https://www.topsoe.com/our-resources/knowledge/our-products/equipment/soec (Accessed November 13, 2023).
Torres, J. R., Tuinema, B. W., Adabi, M. E., Ahmad, Z., Suárez, V. G., Ayivor, P. K., et al. (2019). TSO2020 activity 2: stability analysis of an international electricity system connected to regional and local sustainable gas systems final report.
Tuinema, B. W., Adabi, E., Ayivor, P. K. S., Suárez, V. G., Liu, L., Perilla, A., et al. (2020). Modelling of large-sized electrolysers for real-time simulation and study of the possibility of frequency support by electrolysers. IET Gener. Transm. Distrib. 14, 1985–1992. doi:10.1049/IET-GTD.2019.1364
U.S. Federal Energy Regulatory Commission (2023). Essential reliability services and the evolving bulk-power system-primary frequency response. Available at: https://www.federalregister.gov/documents/2018/03/06/2018-03707/essential-reliability-services-and-the-evolving-bulk-power-system-primary-frequency-response (Accessed October 10, 2023).
Wang, T., Cao, X., and Jiao, L. (2022). PEM water electrolysis for hydrogen production: fundamentals, advances, and prospects. Carbon Neutrality 11 (1), 21–19. doi:10.1007/S43979-022-00022-8
Wang, X., Mardle, P., Adamski, M., Chen, B., and Holdcroft, S. (2023). Proton exchange membrane water electrolysis incorporating sulfo-phenylated polyphenylene catalyst coated membranes. J. Electrochem. Soc. 170, 024502. doi:10.1149/1945-7111/ACB643
Wind & Solar Share in Electricity Production Data (2023). Wind & solar share in electricity production data | Enerdata. Available at: https://yearbook.enerdata.net/renewables/wind-solar-share-electricity-production.html (Accessed October 4, 2023).
Worku, M. Y. (2022). Recent advances in energy storage systems for renewable source grid integration: a comprehensive review. Sustain 14, 5985. doi:10.3390/su14105985
Wu, D., Wang, D., Ramachandran, T., and Holladay, J. (2022). A techno-economic assessment framework for hydrogen energy storage toward multiple energy delivery pathways and grid services. Energy 249, 123638. doi:10.1016/J.ENERGY.2022.123638
Wu, H., Feng, C., Zhang, L., Zhang, J., and Wilkinson, D. P. (2021). Non-noble metal electrocatalysts for the hydrogen evolution reaction in water electrolysis. Electrochem. Energy Rev. 43, 473–507. doi:10.1007/S41918-020-00086-Z
Xie, Z., Yu, S., Yang, G., Li, K., Ding, L., Wang, W., et al. (2021). Optimization of catalyst-coated membranes for enhancing performance in proton exchange membrane electrolyzer cells. Int. J. Hydrogen Energy 46, 1155–1162. doi:10.1016/J.IJHYDENE.2020.09.239
You, S., Træholt, P., Reissner, R., and Zong, Y. (2017). Report. Available at: https://www.qualygrids.eu/app/uploads/sites/5/2017/02/Deliverable-1.1-Electrical-Grid-Service-Catalogue-for-Water-Electrolysers-27-11-2017.pdf.
Yusoff, N. I., Zin, A. A. M., and Khairuddin, A. B. (2017). “Congestion management in power system: a review,” in 3rd Int. Conf. Power Gener. Syst. Renew. Energy Technol. PGSRET 2017, Johor Bahru, Malaysia, 2018-January, 22–27. doi:10.1109/PGSRET.2017.8251795
Zakeri, B., and Syri, S. (2015). Electrical energy storage systems: a comparative life cycle cost analysis. Renew. Sustain. Energy Rev. 42, 569–596. doi:10.1016/J.RSER.2014.10.011
Zenith, F., Flote, M. N., Santos-Mugica, M., Duncan, C. S., Mariani, V., and Marcantonini, C. (2022). Value of green hydrogen when curtailed to provide grid balancing services. Int. J. Hydrogen Energy 47, 35541–35552. doi:10.1016/J.IJHYDENE.2022.08.152
Zhao, W., Nielsen, M. R., Kjær, M., Iov, F., and Nielsen, S. M. (2023). Grid integration of a 500 kW alkaline electrolyzer system for harmonic analysis and robust control. e-Prime - Adv. Electr. Eng. Electron. Energy 5, 100217. doi:10.1016/J.PRIME.2023.100217
Zheng, Y., Huang, C., You, S., and Zong, Y. (2023). Economic evaluation of a power-to-hydrogen system providing frequency regulation reserves: a case study of Denmark. Int. J. Hydrogen Energy 48, 26046–26057. doi:10.1016/J.IJHYDENE.2023.03.253
Zsiborács, H., Pintér, G., Vincze, A., Birkner, Z., and Baranyai, N. H. (2021). Grid balancing challenges illustrated by two European examples: interactions of electric grids, photovoltaic power generation, energy storage and power generation forecasting. Energy Rep. 7, 3805–3818. doi:10.1016/J.EGYR.2021.06.007
Keywords: electrolyzer, ancillary services, grid stability, frequency control, voltage control, grid balancing, hydrogen storage
Citation: Cozzolino R and Bella G (2024) A review of electrolyzer-based systems providing grid ancillary services: current status, market, challenges and future directions. Front. Energy Res. 12:1358333. doi: 10.3389/fenrg.2024.1358333
Received: 19 December 2023; Accepted: 22 January 2024;
Published: 08 February 2024.
Edited by:
Tabish Alam, Central Building Research Institute (CSIR), IndiaReviewed by:
Giovanni Di Ilio, University of Naples Parthenope, ItalyCopyright © 2024 Cozzolino and Bella. This is an open-access article distributed under the terms of the Creative Commons Attribution License (CC BY). The use, distribution or reproduction in other forums is permitted, provided the original author(s) and the copyright owner(s) are credited and that the original publication in this journal is cited, in accordance with accepted academic practice. No use, distribution or reproduction is permitted which does not comply with these terms.
*Correspondence: Raffaello Cozzolino, cmFmZmFlbGxvLmNvenpvbGlub0B1bmljdXNhbm8uaXQ=
Disclaimer: All claims expressed in this article are solely those of the authors and do not necessarily represent those of their affiliated organizations, or those of the publisher, the editors and the reviewers. Any product that may be evaluated in this article or claim that may be made by its manufacturer is not guaranteed or endorsed by the publisher.
Research integrity at Frontiers
Learn more about the work of our research integrity team to safeguard the quality of each article we publish.