- 1Centre for Technology in Water and Wastewater, School of Civil and Environmental Engineering, University of Technology Sydney, Sydney, NSW, Australia
- 2School of Engineering and Technology, Central Queensland University, Rockhampton, QLD, Australia
- 3School of Engineering, Swinburne University of Technology, Hawthorn, VIC, Australia
- 4School of Environmental and Life Sciences, University of Newcastle, Callaghan, NSW, Australia
- 5Mechanical Engineering Department, College of Engineering, King Khalid University, Abha, Saudi Arabia
This review paper delves into the intricate challenge of transforming microalgal biomass into biofuel through anaerobic digestion, elucidating its significance for sustainable energy production and waste management. Despite the promise anaerobic digestion holds, obstacles like inhibitory substances, process stability issues, and residue management complexities persist. Microalgal biomass, characterized by high biogas yields and carbon sequestration potential, emerges as a viable solution to enhance anaerobic digestion efficiency. Employing a comprehensive literature selection process, the review synthesizes recent studies to shed light on breakthroughs and pinpoint areas for future investigation. Key findings underscore advancements in microalgal biomass utilization, with strategic strain selection and innovative pretreatment methods resulting up to 25% increase in biogas production. Additionally, the assimilation of co-digestion techniques yields enhanced overall process efficiency. Microalgal biomass demonstrates remarkable carbon sequestration capabilities, sequestering up to 60% of CO2 during the anaerobic digestion process. Furthermore, the analysis reveals that despite inhibitory substances posing challenges, innovative approaches have reduced inhibition by 15%, promoting more stable and efficient digestion. Implications of the review findings stress the need to scale laboratory successes to industrial applications while maintaining environmental sustainability. Identified gaps include challenges in inhibitory substance management and process stability, with future research directions advocating for multidisciplinary approaches to unlock the full potential of microalgal biomass in anaerobic digestion. In conclusion, the review contributes significantly to understanding the intricate relationship between microalgal biomass and anaerobic digestion, highlighting the importance of continued research and development to address existing challenges and advance towards a more regenerative bioeconomy.
1 Introduction
Anaerobic digestion is recognized as a sustainable and environmentally friendly waste-to-energy technology, offering a compelling solution to address both waste management and renewable energy production. This biological process harnesses the power of microorganisms to break down organic matter in the absence of oxygen, converting it into biogas, which primarily consists of methane (CH4) and carbon dioxide (CO2) (Kirk and Gould, 2020). Anaerobic digestion has gained prominence in recent years due to its capacity to reduce organic waste volumes, mitigate greenhouse gas emissions, and generate renewable energy. Its versatility in treating a wide range of organic substrates, from agricultural residues to municipal solid waste, has made it a key player in the transition to a more sustainable and circular economy (Manyi-Loh et al., 2019). In tandem with the growing interest in anaerobic digestion, microalgal biomass has emerged as a promising feedstock with considerable potential.
Microalgal biomass, composed of microscopic algae, distinguishes itself from other biomass sources due to its diverse and versatile nature (Khoo et al., 2023). Unlike traditional lignocellulosic biomass, microalgae are unicellular organisms that encompass a wide range of species, each exhibiting distinct biochemical compositions. It is essential to recognize that microalgae serve as a valuable feedstock for biofuel production owing to their high growth rates, efficient photosynthetic capabilities, and the ability to thrive in various environmental conditions (Josephine et al., 2022). The constituents of microalgal biomass are integral to its suitability for anaerobic digestion and subsequent biofuel production. Microalgae typically contain lipids, proteins, carbohydrates, and various micronutrients. Lipids, in the form of triglycerides, are particularly significant as they can be converted into biodiesel through transesterification (Egesa and Plucinski, 2024). Proteins and carbohydrates contribute to the overall organic content, influencing the biogas yield during anaerobic digestion (Vargas-Estrada et al., 2022). Moreover, the diverse array of pigments, such as chlorophylls and carotenoids, not only facilitates photosynthesis but also influences the overall chemical composition of microalgal biomass (Maltsev et al., 2021). Microalgal biomass exhibits considerable variation in terms of fatty acid profiles, cell wall structures, and overall biochemical makeup. The lipid content, fatty acid composition, and structural components vary across different microalgal strains. Providing a nuanced understanding of these variations is crucial for comprehending the intricacies of anaerobic digestion and biofuel production from microalgal biomass.
The idea of harnessing biogas from microalgae, while not a new one and dating back over six decades, initially faced formidable challenges (Schenk et al., 2008). Early experiments in this domain yielded minimal biogas production, primarily attributed to the formidable barrier posed by the dense cell walls of microalgae, which restricted the access of anaerobic microorganisms to the organic material (Sharma et al., 2013). Furthermore, the presence of microorganisms characterized by low carbon-to-nitrogen (C/N) ratios fostered the accumulation of ammonia, hampering the growth of methanogenic bacteria (Nwokolo and Enebe, 2022). Nonetheless, the landscape in this field has undergone significant transformation thanks to technological advancements and persistent research efforts that have adeptly fine-tuned the variables influencing the anaerobic digestion process. This collective endeavour has borne fruit, capturing the attention of scholars and stakeholders. Biogas production from microalgae has demonstrated the potential to yield a substantial 1 kWh/kg of volatile solids (VS) in the form of electrical energy (Park et al., 2011; Xia and Murphy, 2016).
While anaerobic digestion offers significant advantages for harnessing energy from microalgae biomass, notably the elimination of the need to extract specific macromolecules like lipids, proteins, or carbohydrates, it is vital to acknowledge the substantial challenges in terms of operational and energy expenses. These challenges cast doubts on the feasibility of commercial applications. However, within this context, the integration of residual microalgae biomass (RMB) into the anaerobic digestion process presents a promising opportunity (Vargas-Estrada et al., 2022). The potential of combining RMB with microalgae culture, particularly in the context of wastewater treatment, enhances this prospect. Despite encountering various hurdles, such as low lipid content and the presence of other microorganisms in microalgae cultivated in wastewater, anaerobic digestion emerges as the most practical method for effectively harnessing energy from this resource. The anaerobic digestion of microalgae can be seamlessly executed using either the original, unaltered microalgae biomass or the RMB, especially after the extraction of lipids or high-value products. This approach aligns with sustainable practices and actively supports the establishment of a circular economy.
Recent research articles have provided valuable insights into biofuel production from microalgal biomass through anaerobic digestion. For example, Yu et al. (2015) focused on strategic strain selection, emphasizing the importance of choosing microalgal strains with enhanced digestibility and biogas production potential. This approach involves the identification and cultivation of microalgae species with traits that facilitate efficient breakdown during anaerobic digestion. Recent studies, including the work of Pugazhendi et al. (2022), have explored innovative pretreatment methods to enhance the accessibility of microalgal biomass to anaerobic microorganisms. Techniques such as disperser and biosurfactant were investigated to break down the formidable barrier posed by the dense cell walls of microalgae. Cabeza et al. (2023) delved into the efficacy of co-digestion techniques, where microalgal biomass is combined with bacterial biomass to enhance the overall anaerobic digestion process. This collaborative approach aims to optimize the nutrient composition and balance, promoting a more favourable environment for microbial activity and biogas production. Various studies, including contributions by Scarponi et al. (2024), Luna-Avelar et al. (2021) and Munisamy Sambasivam et al. (2023) have highlighted the transformative impact of technological advancements on the anaerobic digestion of microalgal biomass. These advancements include process optimization through the fine-tuning of variables influencing anaerobic digestion, leading to increased efficiency and biogas yields.
The amalgamation of recent studies into this review paper serves a pivotal role, fulfilling various essential objectives. Initially, it intricately weaves together a diverse array of findings and methodologies, crafting a comprehensive panorama of the latest advancements in the field. This synthesis is integral, providing researchers, policymakers, and industry experts with convenient access to a consolidated reservoir of knowledge. Additionally, the meticulous examination of multiple studies allows the identification of discernible patterns and trends in methodologies and outcomes. This not only guides future research directions but also enhances the efficacy of decision-making processes. Moreover, the review paper plays a crucial role in addressing existing knowledge gaps, illuminating areas requiring further exploration. Such insights are invaluable, shaping the trajectory of subsequent research endeavours. Lastly, by compiling recent studies, this review paper acts as a roadmap for upcoming research, offering nuanced insights into the most promising strategies and methodologies. This guidance is indispensable for researchers seeking to navigate the dynamic landscape of biofuel production from microalgal biomass through anaerobic digestion, fostering a more informed and strategic approach to overcoming existing challenges.
Several review papers have explored the integration of microalgal biomass into anaerobic digestion for biofuel production. Notable contributions in this domain include works by Gonzalez-Fernandez et al. (2015), Neves et al. (2016), Wirth et al. (2018), and Solé-Bundó et al. (2019). These prior reviews have extensively covered the historical landscape, challenges, and advancements in harnessing biogas from microalgae. While these reviews offer valuable insights, the current review distinguishes itself through its specific focus on the evolving symbiosis between microalgal biomass and anaerobic digestion, positioning it as a unique contribution to the existing literature. Unlike previous reviews that provide broad overviews, this review delves into the nuances of the anaerobic digestion process concerning microalgal biomass, narrowing its scope to this specific intersection. It critically assesses recent technological advancements, innovative pretreatment methods, and co-digestion techniques that have significantly enhanced biogas production. Furthermore, while acknowledging the historical challenges faced in this field, this review uniquely emphasizes how persistent research efforts and technological innovations have overcome barriers, making biogas production from microalgae a more viable prospect today.
Thus, the objective of this concise review is to scrutinize both the potential and the obstacles associated with the use of microalgal biomass in anaerobic digestion. The structure of this review paper is designed to comprehensively explore the symbiotic relationship between microalgal biomass and anaerobic digestion. Beginning with an examination of Microalgal Biomass as a Feedstock of Biofuel (Section 2), the review delves into the intricacies of the Anaerobic Digestion Process of Microalgal Biomass (Section 3). Section 4 scrutinizes Factors Affecting the Anaerobic Digestion of Microalgal Biomass, providing insights into the complexities and variables influencing this biological process. Opportunities in Anaerobic Digestion of Microalgal Biomass (Section 5) are then highlighted, emphasizing the potential benefits and applications in the transition to a sustainable bioeconomy. Conversely, Challenges in Anaerobic Digestion of Microalgal Biomass (Section 6) address the hurdles and limitations that demand attention. Recent Advances and Research Trends (Section 7) encapsulate the collective efforts and breakthroughs, offering a forward-looking perspective on the evolving landscape. Finally, Section 8 extrapolates the potential implications of the reviewed literature in a real-world context, providing a holistic view of the practical applications and implications of harnessing biofuel from microalgal biomass through anaerobic digestion. This structured exploration aims to provide readers with a nuanced understanding of the current state of research, laying the foundation for the subsequent discussions and insights presented in this review paper.
2 Microalgal biomass as a feedstock of biofuel
Microalgae, microscopic photosynthetic organisms, have garnered increasing attention as a potential feedstock for anaerobic digestion due to their unique characteristics, composition, and growth dynamics. These attributes make them a distinctive candidate for biogas production and sustainable waste management. Microalgae are characterized by their rapid growth rates, which can surpass that of traditional terrestrial crops. Their growth is fuelled by photosynthesis, making them highly efficient in converting solar energy into biomass (Hallenbeck et al., 2016). This feature is particularly advantageous in the context of anaerobic digestion, as it ensures a consistent and potentially year-round supply of feedstock (Colling Klein et al., 2018). The composition of microalgal biomass is another critical aspect. Microalgae are rich in organic matter, with protein content ranging from 10% to 70%, lipids ranging from 5% to 50%, and carbohydrates ranging from 5% to 40% (Niccolai et al., 2019). This composition makes them suitable for biogas production and nutrient recovery, as they provide a diverse mix of organic substrates. Furthermore, microalgae exhibit a broad species diversity, each with specific growth characteristics and nutrient requirements. This diversity allows for tailoring feedstock selection to optimize anaerobic digestion processes, enhancing biogas production and overall system efficiency (Siddiki et al., 2022).
Comparing microalgal biomass to other common feedstocks in anaerobic digestion, such as agricultural residues and organic municipal waste, reveals several key advantages. First, as mentioned earlier, microalgae’s rapid growth rates lead to higher biomass productivity, potentially exceeding traditional feedstocks (Abomohra et al., 2016). This accelerated growth offers the potential for year-round supply, reducing seasonality constraints that affect many other feedstocks. Additionally, the high nutrient content in microalgal biomass makes it an excellent candidate for nutrient recovery from anaerobic digestion effluents. Nutrient-rich digestate can be utilized as a valuable fertilizer in agriculture (Bauer et al., 2021). In contrast to lignocellulosic feedstocks, such as crop residues, microalgal biomass typically has a lower lignin content. This lower lignin content renders it more easily digestible by anaerobic microorganisms, leading to higher biogas yields and shorter retention times (Wargacki et al., 2012).
The advantages of utilizing microalgal biomass as a feedstock for anaerobic digestion extend beyond its growth and composition. Microalgae can capture and store carbon dioxide during growth, contributing to carbon sequestration and potentially reducing greenhouse gas emissions (Verma and Srivastava, 2018); Microalgae can be cultivated in a smaller land area compared to traditional energy crops, making them suitable for urban and industrial applications (Milano et al., 2016); Microalgal biomass has been reported to produce higher CH4 yields compared to lignocellulosic materials, making it an attractive feedstock for biogas production (Martínez-Gutiérrez, 2018); Beyond biogas production, microalgal biomass can also be used to extract value-added products such as biofuels, pigments, and high-value chemicals (Malik et al., 2022). In summary, microalgal biomass possesses unique characteristics, composition, and growth patterns that distinguish it as a promising feedstock for anaerobic digestion. These attributes, along with its advantages in terms of biogas production and sustainability, make microalgal biomass a compelling choice for integrated waste-to-energy systems.
2.1 Pretreatment methods for anaerobic digestion of microalgal biomass
The anaerobic digestion of various substrates often faces challenges due to low biodegradability. To overcome this, a range of pretreatment methods have been developed to enhance the hydrolysis step, which is essential for breaking down cell walls (Zhen et al., 2017) and making organic matter available to anaerobic microorganisms (Passos and Ferrer, 2014). These pretreatments can be broadly categorized into four main groups: thermal, mechanical (including techniques like ultrasound and microwave), chemical (employing agents such as acids, alkalis, solvents, and ozone), and thermo-chemical (a combination of acid or alkali reagents with high temperatures). Additionally, biological methods using enzymes and microorganisms are also employed. These pretreatments have been the subject of extensive research over the past decade, particularly for their role in improving biogas production from microalgae biomass. The diverse range of pretreatment methods for preparing microalgal biomass for anaerobic digestion and subsequent biogas production is illustrated in Figure 1.
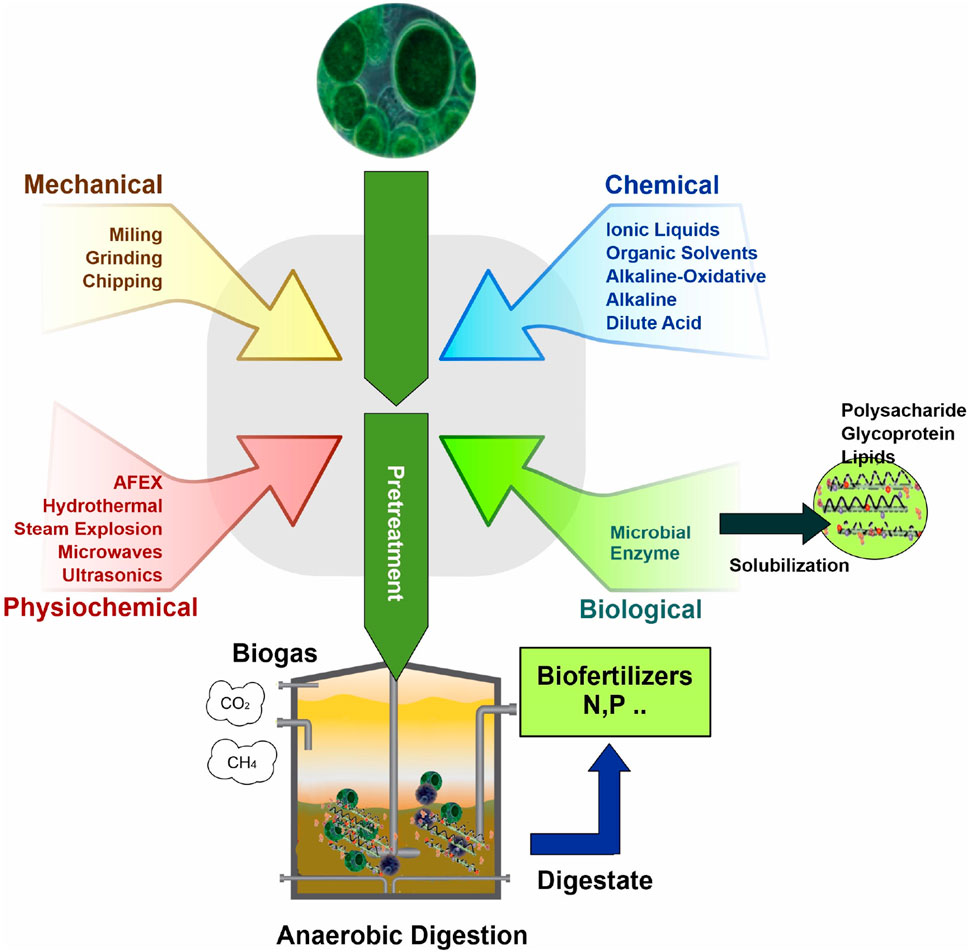
FIGURE 1. Different pretreatment methods of microalgal biomass to use in anaerobic digestion process for producing biogas.
Three pretreatments method including thermal, thermo-chemical, and mechanical used in the production of biogas require a significant amount of energy. These methods involve the application of heat (thermal), a combination of heat and chemical reactions (thermo-chemical), or physical forces (mechanical) to break down organic material, making it more accessible for biogas production. However, the energy required to generate the necessary temperatures, chemical reactions, or mechanical forces is substantial, leading to high energy consumption in these stages of biogas production. To assess their efficiency, it is crucial to consider the final energy balance of the pretreatment process. In biogas production facilities, thermal energy is often readily available, making thermal pretreatments a popular choice. These involve heating the biomass at temperatures ranging from 50°C to 270°C for varying durations, from minutes to hours. The effectiveness of thermal pretreatment depends on factors such as the specific microalgae strain and the temperature applied (Ruiz et al., 2017). For example (González-Fernández et al., 2012b), found that treating Scenedesmus at 80°C for just 15 min yielded comparable results, suggesting that temperature is a more critical factor than duration in thermal pretreatment. Further research by Wang et al. (2017) on Chlorella biomass showed that heating at 70°C and 90°C for 30 min enhanced CH4 yield by 37% and 48%, respectively, compared to untreated biomass. These findings indicate that the impact of thermal pretreatments is strain-specific, with different temperatures yielding varied results depending on the biomass used. Experiments with higher temperatures, such as 130°C for 15–30 min, also showed a 28% increase in CH4 yield with a raw biomass mixture of green algae and diatoms. However, moderate temperatures between 80°C and 120°C are more commonly tested due to the potential formation of Maillard compounds at higher temperatures, which can negatively impact the process (Passos et al., 2015).
The mode of operation during digestion, whether batch or semicontinuous, also affects the outcome of thermal pretreatments (Mendez et al., 2015). Reported that using 120°C for 40 min in a CSTR increased CH4 yield by 1.5 times compared to raw Chlorella biomass, though the results were 50% lower than those obtained in batch mode. This highlights the importance of testing each pretreatment in different operational modes. While thermal pretreatments generally improve CH4 yield, the results vary significantly based on the biomass type, temperature, duration of pretreatment, and mode of operation during digestion. However, these methods also have downsides, such as the formation of recalcitrant compounds, which can decrease process efficiency (Alzate et al., 2012; Mendez et al., 2014).
Mechanical pretreatments, particularly ultrasound, are pivotal in processing organic substrates. Studies show ultrasound’s effectiveness in breaking down microalgae cell walls, crucial for biofuel production (Rodriguez et al., 2015; Carrere et al., 2016). For instance, ultrasound significantly increased CH4 yield from Scenedesmus biomass, but the concurrent temperature rises during ultrasound also contributed to this effect, questioning its exclusive benefits over thermal methods (González-Fernández et al., 2012b; Passos and Ferrer, 2015). Moreover, while varying energy inputs on different microalgae biomass mixtures did enhance CH4 yield, higher energy inputs beyond a certain point did not yield significant improvements (Alzate et al., 2012). However, ultrasound’s high energy requirement remains a major limitation, especially compared to other methods like thermal, chemical, or biological treatments (Passos et al., 2014).
In the realm of pretreatment methods for microalgae biomass used in biogas production, chemical methods often go hand in hand with heat pretreatment, known as thermochemical pretreatments. However, these methods have seen less usage compared to thermal and mechanical pretreatments. This is primarily due to the potential toxicity these chemicals may pose to anaerobic microorganisms involved in the digestion process. Despite this, there’s evidence that cell wall disruption through alkali and acid pretreatments can be effective. Such methods have shown positive results in producing ethanol, butanol, and bioCH4 from microalgae biomass, as demonstrated in studies by Efremenko et al. (2012); Wang et al. (2016). Nevertheless, alkali and acid pretreatments have shown effectiveness in cell wall disruption and subsequent CH4 yield increase. For instance, thermo-alkaline methods using reagents like NaOH or CaO have yielded positive results in protein and carbohydrate solubilization and CH4 yield enhancement (Solé-Bundó et al., 2017a). Thermo-acid pretreatments also showed promise, particularly with sulfuric acid treatments enhancing carbohydrate solubilization (Mendez et al., 2013). However, post-pretreatment pH adjustments and chemical removal are necessary, adding complexity and potential costs (Pandey et al., 2014).
Biological pretreatments, involving enzymes or microorganisms, are notable for their lower energy requirements and specificity. This approach requires selecting effective enzymes based on cell wall composition, with carbohydrases being common due to structural similarities between higher plants and microalgae. Enzymes like cellulases, hemicellulases, amylases, and pectinases, along with other enzymatic cocktails, have been explored for hydrolysis, with protease-treated biomass showing substantial increases in CH4 yield (Carrillo-Reyes et al., 2016; Chng et al., 2017). These pretreatments can significantly enhance CH4 yield, though the extent varies depending on the specific microalgae biomass and digestion period. Overall, these pretreatment methods, each with their unique mechanisms and outcomes, play a vital role in optimizing biogas production from different microalgae strains. Table 1 lists various pretreatment methods employed by researchers to optimize biogas production using different microalgae strains.
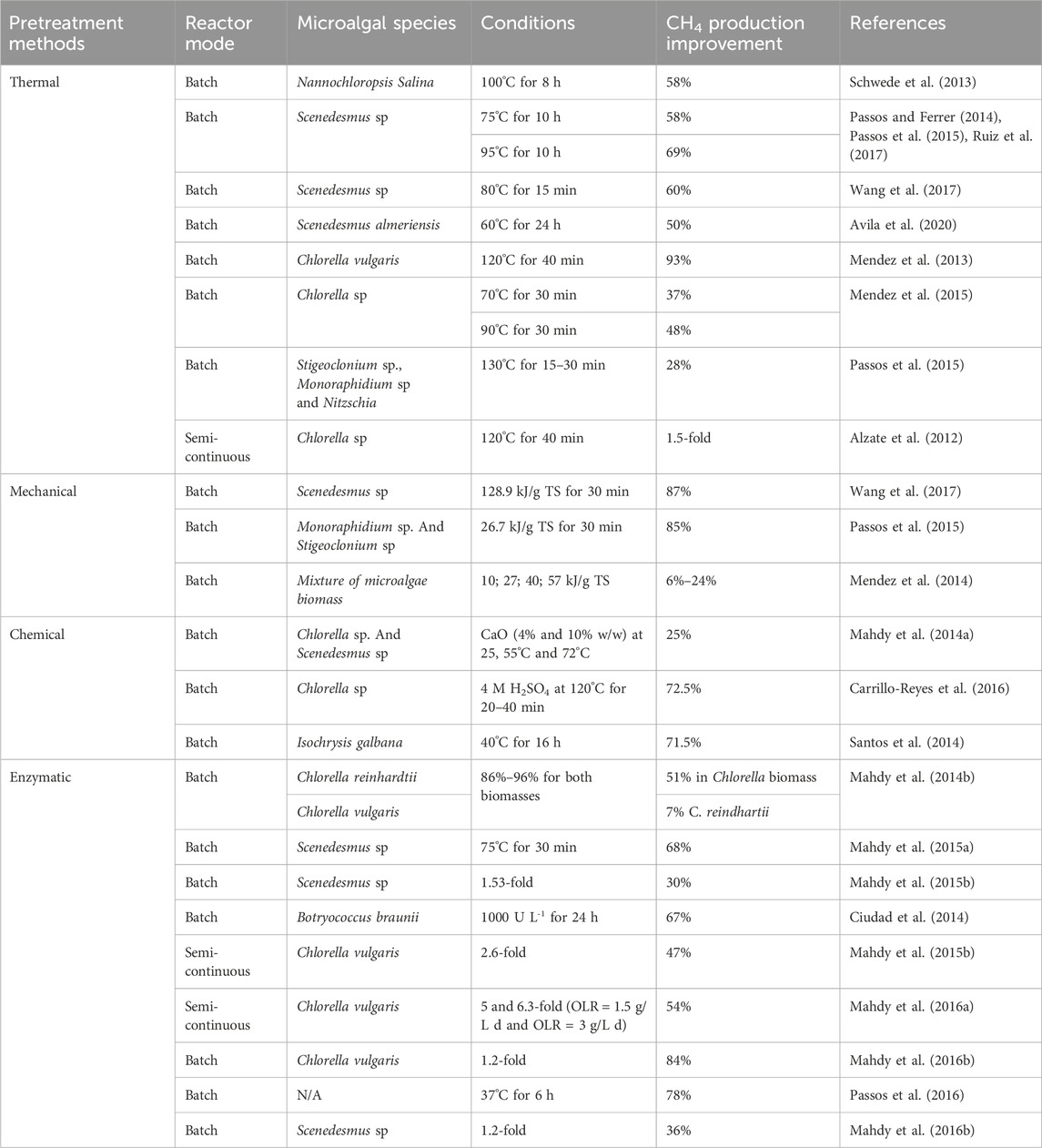
TABLE 1. List of different pretreatment methods employed by researchers to optimize biogas production using different microalgae strains.
In summary, the exploration of various pretreatment methods for microalgal biomass in the context of anaerobic digestion and biogas production reveals a nuanced landscape with distinct advantages and limitations. Thermal pretreatments, relying on the application of heat, demonstrate strain-specific effects, with varying temperatures and durations yielding diverse outcomes in terms of CH4 yield. While higher temperatures may enhance CH4 production, the risk of Maillard compound formation at extreme temperatures necessitates careful consideration. Mechanical pretreatments, particularly ultrasound, showcase effectiveness in breaking down microalgal cell walls but are hindered by their high energy requirements. Chemical pretreatments, especially thermo-alkaline and thermo-acid methods, exhibit promise in disrupting cell walls and enhancing CH4 yield but involve post-pretreatment adjustments and potential costs. Biological pretreatments, leveraging enzymes like cellulases and proteases, offer a lower energy alternative with specificity based on biomass composition. The selection of the optimal pretreatment method for optimized biogas production should consider the energy efficiency, strain-specific responses, and the overall economic feasibility. Given the energy-intensive nature of thermal, thermo-chemical, and mechanical methods, the potential benefits must be weighed against the associated energy costs. Conversely, biological pretreatments present a greener and potentially more sustainable approach. However, a comprehensive evaluation considering the specific microalgal strain, digestion conditions, and economic factors is essential for making informed decisions. Future research should focus on refining these pretreatment methods, considering their integration into large-scale biogas production facilities, and addressing the economic and environmental implications associated with each approach.
3 Anaerobic digestion process of microalgal biomass
Anaerobic digestion of microalgal biomass involves a series of biochemical reactions mediated by complex microbial communities, resulting in the conversion of organic matter into biogas, primarily composed of CH4 and CO2. This process can be divided into several key stages including hydrolysis, Acidogenesis, Acetogenesis, Methanogenesis. In the initial phase, hydrolytic bacteria play a crucial role. These microorganisms, such as Clostridium and Bacteroides species, secrete hydrolytic enzymes that break down complex organic molecules within microalgal biomass. As a result, lipids, proteins, and carbohydrates are converted into simpler, soluble compounds. This initial breakdown is essential because it makes the organic matter more accessible to subsequent microbial degradation (Menzel et al., 2020).
After the initial hydrolysis phase, where complex organic compounds are broken down into simpler soluble substances, acidogenic bacteria assume a pivotal role in the anaerobic digestion process. Notable among these bacteria are species such as Clostridium, Streptococcus, and Lactobacillus. These microorganisms facilitate further fermentation of the hydrolysed products, efficiently converting them into volatile fatty acids (VFAs), alcohols, and various intermediate compounds. During this acidogenic fermentation stage, the complex organic matter from the hydrolysis step is transformed into simpler organic acids, a process critical for the subsequent stages of digestion (Li et al., 2021).
Following the acidogenic phase, acetogenic bacteria, exemplified by species like Syntrophobacter and Syntrophomonas, commence their role. These bacteria undertake the intricate task of converting the previously formed VFAs into acetate, hydrogen, and CO2. This conversion is a delicate and essential intermediary step, setting the stage for the final act of biogas production: methanogenesis (Amin et al., 2021).
Methanogenesis is the ultimate and decisive phase in the anaerobic digestion process, driven by methanogenic archaea such as Methanosarcina and Methanobacterium species. These archaea are specialized in their function, taking the acetate, hydrogen, and CO2 produced by the acetogens and synthesizing CH4 and CO2. The biogas produced at this juncture, primarily composed of CH4, can be captured and utilized for energy generation among other uses (Holmes and Smith, 2016). Figure 2 presents standard procedures involved in anaerobic digestion process of microalgal biomass to produce biogas. However, the efficiency and effectiveness of anaerobic digestion rely heavily on maintaining a balanced microbial ecosystem. This balance is achieved through meticulous regulation of various operational parameters, including pH levels, temperature, and hydraulic retention time. By carefully modulating these factors, the growth and metabolic activity of the microbial consortium are optimized, ensuring a stable and productive anaerobic digestion process.
A diverse array of research endeavours has delved into the anaerobic digestion of microalgal biomass, yielding valuable insights into the impact of various factors. For example, Carrillo-Reyes et al. (2021) reported that the operational conditions, particularly temperatures of 35°C and 55°C) and hydraulic retention time (HRT) of 15 and 30 days, significantly influenced biogas yield. Higher temperatures (e.g., 55°C) can enhance microbial activity, leading to increased biogas production. Longer HRT allows more time for microorganisms to break down organic matter, potentially resulting in higher gas yields. González-Fernández et al. (2012c) investigated the CH4 production from microalgal species, including Scenedesmus sp., in batch anaerobic digesters, reporting low biogas yield of 134 mL/g VS. This could be attributed to the specific strain’s characteristics, such as low organic content or challenging cell wall composition, making it less suitable for efficient anaerobic digestion. Srinuanpan et al. (2017) studied the anaerobic digestion of five oleaginous microalgae strains, focusing on lipid content’s impact (ranging from 21% to 42%), which was found to influence biogas production.
Lipids are energy-rich molecules, and strains with higher lipid content offer a greater potential for biogas production since lipids can be converted to CH4 during anaerobic digestion. Mairet et al. (2012) examined the effects of different reaction conditions, reporting variations in biogas yield from microalgae strain Chlorella vulgaris. These variations could be attributed to factors such as the availability of nutrients, the specific microorganism consortium present, or variations in the microalgal biomass’s composition. In parallel, Ramos-Suárez and Carreras (2014) noted the importance of pH control in microalgal biomass digestion, with neutral pH (around 7) enhancing biogas production. A neutral pH is often preferred because it supports the activity of a wide range of anaerobic microorganisms involved in the digestion process.
Furthermore, Kinnunen et al. (2014) explored temperature’s effect on the anaerobic digestion of microalgae, observing the highest CH4 production at mesophilic conditions (e.g., 37°C). Mesophilic temperatures (25°C–40°C) are favourable for the activity of a broader spectrum of microorganisms and enzymes involved in anaerobic digestion, leading to increased CH4 yield. In the study by Aramrueang et al. (2016), the impact of hydraulic retention time (HRT) on Spirulina platensis digestion was investigated, with longer HRT (e.g., 25 days) resulting in increased CH4 yield. Longer HRT allows for more complete digestion of organic matter, which can lead to higher CH4 production. Meanwhile, Rincón-Pérez et al. (2021) studied the impact of pH levels on biogas production from Scenedesmus obtusiusculus, reporting pH 8.8 in a range of 7.5–9, as optimal. Different microorganisms have different pH optima, and pH control ensures the activity of a broader spectrum of microorganisms, which can enhance biogas production. Table 2 summarises various research findings of biogas production from microalgal biomass using different microalgae strains.
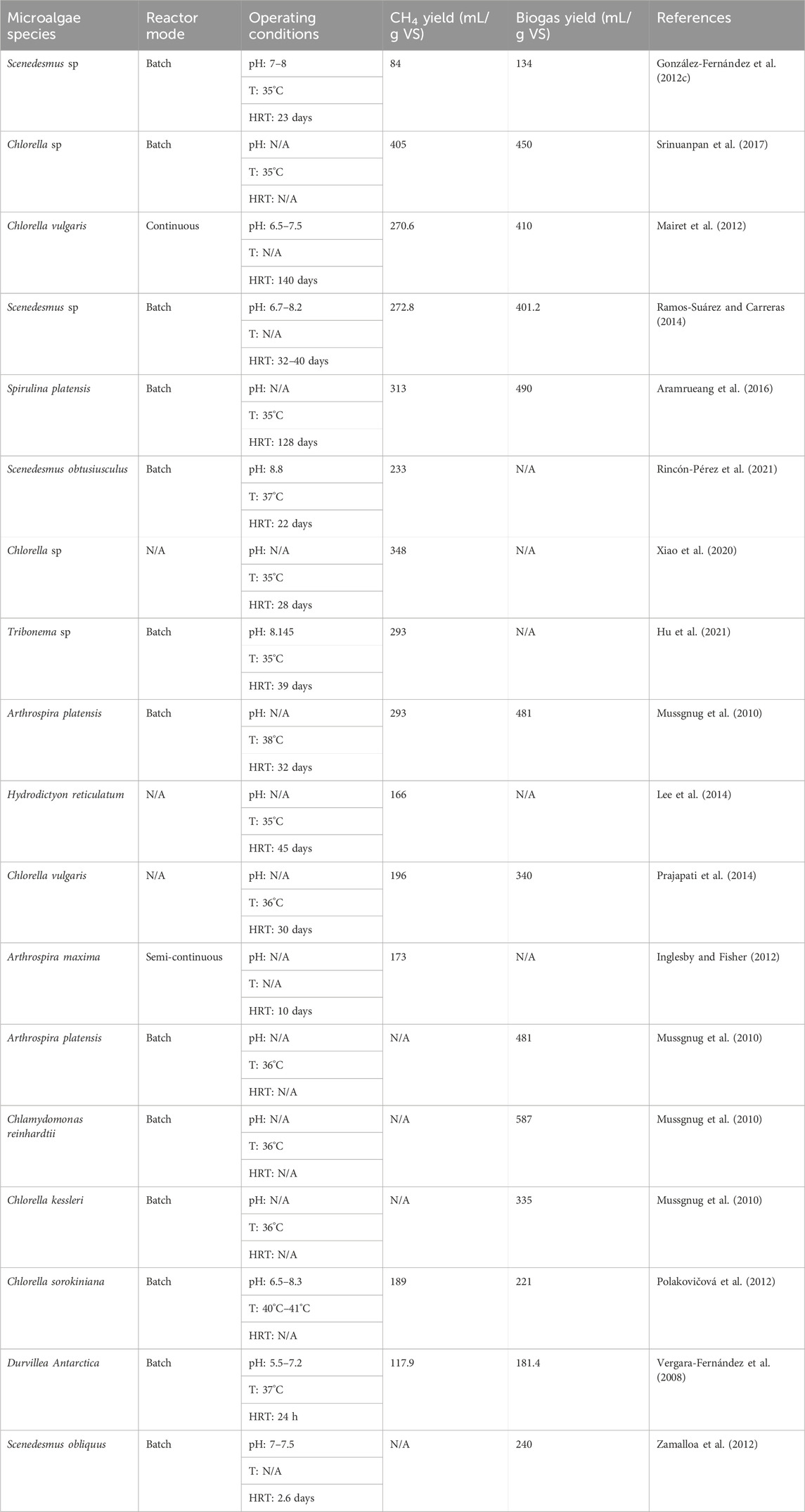
TABLE 2. Research findings of biogas production from microalgal biomass using different microalgae strains.
4 Factors affecting the anaerobic digestion of microalgal biomass
The efficiency of the AD process is influenced by several key factors, each playing a crucial role in the overall effectiveness of the system. Figure 3 illustrates various factors which significantly influence the anaerobic digestion process of microalgal biomass. Firstly, the organic loading rate determines the amount of organic matter available for microbial action. Secondly, the retention time, which is the duration for which the substrates remain in the system, is critical for ensuring complete digestion. Thirdly, the temperature of the process significantly affects microbial activity and gas production. The pH level is another essential factor, as it needs to be maintained within a specific range for optimal microbial growth and activity.
The quality of the substrates, including the characteristics of their cell walls and any pretreatments they have undergone, also greatly influences the AD process. Substrates that are more readily biodegradable or have undergone effective pretreatment can significantly enhance biogas production. Lastly, the presence of methanogenesis inhibitors can adversely affect the process, as these inhibitors can hinder the activity of methanogens, the microorganisms responsible for CH4 production in the AD process. Understanding and managing these factors is crucial for optimizing the AD process for efficient biogas production (Yadvika et al., 2004; Harun et al., 2010; González-Fernández et al., 2012a; Saharan et al., 2013). All of those are described below. In the study of anaerobic digestion (AD) for biogas production, several factors significantly influence the efficiency and yield of CH4. One of these factors is the rate of organic loading and retention times. Higher rates of organic compounds have been linked to increased CH4 yield, as have longer solid retention times. Conventional biogas plants typically operate with a hydraulic retention time (HRT) of 30–50 days, whereas for microalgae AD at a laboratory scale, an HRT of 15–30 days is suggested (Yadvika et al., 2004; Chynoweth, 2005; Ehimen et al., 2011; Ras et al., 2011; Diltz and Pullammanappallil, 2013).
Temperature is another critical factor in AD, with effective ranges including mesophilic (30°C–38°C) and thermophilic (50°C–55°C) conditions. Increasing the temperature can enhance CH4 production, likely due to reduced microalgae photosynthesis activity at higher temperatures (González-Fernández et al., 2012a). It is been found that the efficiency of microalgae biomass digestibility is similar at 20°C and ambient temperature, provided it does not drop below 16°C (Kinnunen et al., 2014). pH level is also crucial, with the optimum range for methanogenesis being between 6.5 and 8.5. Varying pH levels have been reported in different contexts, and adjustments are often made to maintain optimal conditions for methanogenesis (Angelidaki et al., 2002; Harun et al., 2010; Wang et al., 2013). Inhibitory factors such as ammonia concentration and the carbon/nitrogen (C/N) ratio significantly affect methanogenesis. High ammonia concentrations can inhibit methanogenesis, and to counter low algae C/N ratios, carbon-rich substrates may be added. The ideal C/N ratio for AD is between 26 and 31 (Yen and Brune, 2007; Demirbas, 2010; Iyovo et al., 2010; Wang et al., 2013). Finally, the inoculum to substrate ratio (ISR) is vital for CH4 yield. A decrease in ISR can lead to reduced CH4 yield, with the highest yield achieved at an ISR value of 1.0. An ISR of 1.0 is also effective for preventing pH drops and long-chain fatty acid inhibition (Zhao et al., 2014).
In addition to the previously mentioned factors, there are other elements that can impact CH4 production in the anaerobic digestion (AD) process. Among these, two operational parameters stand out: nitrogen deficiency and the methods used for harvesting and storing microalgal biomass. Nitrogen deficiency during microalgae cultivation has been linked to increased production of intracellular lipids, as noted by Chu et al. (2013). This deficiency leads to a starvation strategy, which, in turn, decreases microalgae productivity and affects digestibility in two primary ways. Firstly, there’s a change in cell morphology, characterized by the accumulation of lipids, proteins, and carbohydrates within the cell. This results in increased cell volume and wall thickness (Safi et al., 2014; Ellegaard and Ribeiro, 2018). Secondly, microalgae respond to starvation by secreting exudates, which can build up on the external part of the cell wall and protect it against enzymatic breakdown (González-Fernández et al., 2012a; Srivastava et al., 2021; Tong et al., 2023). The timing of microalgae harvesting is also crucial for AD, as it influences the distribution of intracellular macromolecules, which vary throughout the growth stages of microalgae. Harvesting at an optimal growth stage can ensure a higher concentration of desired macromolecules (González-Fernández and Ballesteros, 2013; McPherson and Cudney, 2014; Jankowska et al., 2017). It is hypothesized that cyst formation during maturation can reduce algae digestibility (Miao et al., 2010).
However, different harvesting methods, such as centrifugation, filtration, or flocculation, do not seem to affect this macromolecular distribution (Harith et al., 2010). Specific operating conditions have been found to increase the concentration of favourable macromolecules (González-Fernández et al., 2012a). Storage techniques significantly affect the biochemical composition of microalgae. Various studies have noted that storage temperature is a critical factor. For instance, freezing can reduce carbohydrate and protein content (Babarro et al., 2001), and temperatures between 40°C and 60°C can alter macromolecular distribution (Zepka et al., 2008). Additionally, decreases in organic compound content have been observed due to bacterial degradation, chemical oxidation, or the presence of protease enzymes (Ruggaber and Talley, 2006; Mahajan and Gupta, 2015).
5 Opportunities in anaerobic digestion of microalgal biomass
The anaerobic digestion of microalgal biomass presents a myriad of opportunities that extend beyond traditional biofuel production. Numerous researchers have explored the multifaceted benefits and promising avenues of the anaerobic digestion of microalgal biomass. For example, Doğan-Subaşı and Demirer (2016) reported a significant increase in biogas production from the anaerobic digestion of C. vulgaris microalgal biomass. The study demonstrated an enhanced biogas yield of 238 mL/g VS (volatile solids) added, highlighting the potential of microalgae as a high-yield feedstock. Microalgal biomass often yields biogas with a high CH4 content. Research by Mendez et al. (2014) reported a CH4 content of approximately 67.5% in the biogas produced from microalgal biomass, underlining the potential for high CH4 yield from microalgae.
The anaerobic digestion of microalgal biomass not only generates biogas but also provides opportunities for nutrient recovery and recycling. For instance, Kisielewska et al. (2022) emphasized the nutrient-rich nature of the digestate produced from microalgal biomass digestion. The digestate was found to contain valuable nutrients, including total nitrogen at 1.4 g/L and total phosphorus at 0.15 g/L. These values underscore the potential for recycling essential nutrients into agricultural systems. Sayedin et al. (2020) investigated the potential for phosphorus recovery from microalgal biomass through anaerobic digestion. The study demonstrated effective phosphorus recovery, with a concentration of 87.2 mg/L in the digestate, addressing concerns about phosphorus scarcity and environmental impact.
The anaerobic digestion of microalgal biomass contributes to the reduction of greenhouse gas emissions. Sepulveda et al. (2019) noted the carbon sequestration potential of microalgae. During growth, microalgae can capture and store CO2. The study reported a carbon sequestration rate of approximately 1.83 kg of CO2 per kg of microalgal biomass, highlighting its role in reducing net greenhouse gas emissions. The co-digestion of microalgal biomass with other organic substrates presents an opportunity to optimize anaerobic digestion processes. Zhang et al. (2020) demonstrated the synergistic effect of co-digesting microalgal biomass with food waste. The study reported an improved biogas yield of 0.43 L/g VSadded, showing the potential for enhanced process efficiency through co-digestion. Co-digestion also offers the opportunity to divert organic waste from landfills, reducing environmental burdens. Khalid et al. (2011) highlighted the potential to integrate various organic substrates into anaerobic digestion systems, further contributing to waste management and reducing the environmental impact of organic waste disposal.
These findings emphasize the significant opportunities presented by the anaerobic digestion of microalgal biomass, ranging from enhanced biogas production and CH4 yield to nutrient recovery, greenhouse gas reduction, and the potential for co-digestion with other organic substrates. Microalgal biomass stands as a promising and sustainable resource for waste-to-energy systems and environmental management.
6 Challenges in anaerobic digestion of microalgal biomass
The anaerobic digestion of microalgal biomass presents several challenges related to the presence of inhibitory substances. High lipid content in microalgal biomass can lead to the release of inhibitory compounds, such as long-chain fatty acids, which can hinder the anaerobic digestion process. For instance, Dasa et al. (2016) reported the inhibition of methanogenesis due to long-chain fatty acids in the anaerobic digestion of microalgal biomass. They found that inhibitory concentrations of long-chain fatty acids significantly impacted CH4 production. Some microalgal species produce toxins, such as microcystins, which are harmful to anaerobic microorganisms. This can lead to process inhibition, especially when microalgal biomass contains toxin-producing species (Yuan et al., 2011).
Anaerobic digestion of microalgal biomass faces challenges related to process instability and operational difficulties. Foaming is a common issue in anaerobic digesters treating microalgal biomass. Timira et al. (2022) reported that foaming is a challenge in microalgal biomass digestion due to the release of surfactants during cell disruption. Foaming can disrupt reactor operation and efficiency. Additionally, anaerobic digestion of microalgal biomass can result in high solids content in the digester, leading to challenges in maintaining adequate mixing and mass transfer. Research by Valigore et al. (2012) noted that the high solids content in microalgal biomass digestion affects process stability and can result in solids settling and poor mixing.
Seasonal variations in microalgal growth can pose challenges to the consistency of feedstock supply for anaerobic digestion. Microalgal biomass production is often influenced by seasonal variations in light intensity and temperature. This seasonal fluctuation can lead to inconsistent feedstock quality and quantity for anaerobic digestion, affecting process performance and biogas production (Grobbelaar, 2010). Challenges in post-digestion treatment and algae residue management need to be addressed. After anaerobic digestion, microalgal biomass residues may require dewatering and proper management. Research by Stiles et al. (2018) discussed the challenges associated with dewatering digestate from microalgal biomass digestion, which can be energy-intensive and require additional treatment. The management and disposal of algae residues can pose environmental challenges. The concentrated residues from microalgal biomass digestion may require appropriate disposal strategies to avoid environmental impacts (Rashid et al., 2013).
7 Recent advances and research trends
Recent studies have introduced innovative approaches to advance the anaerobic digestion of microalgal biomass. Researchers have been meticulously selecting algal strains to boost biogas production. For instance, Bohutskyi et al. (2014) observed a substantial 50% increase in biogas production by carefully choosing microalgal strains with elevated biomass and lipid content. This strategic selection resulted in an impressive biogas yield of 0.51 L/g VSadded, a significant improvement compared to the control group’s yield of 0.34 L/g VS. In addition to strain selection, pre-treatment methods have been explored to enhance the digestibility of microalgal biomass. Lee et al. (2014) delved into ultrasonication pre-treatment technique for microalgae Hydrodictyon reticulatum and found an increased CH4 production of 384 mL/g VS that was 2.3 times higher than the untreated microalgae. Moreover, thermo-alkaline pre-treatment utilised by Candia-Lomeli et al. (2022) delivered a remarkable 46% boost in CH4 production. These findings underscore the potential of pre-treatment approaches to maximize biogas production. Furthermore, researchers have been actively investigating co-digestion strategies to improve overall biogas production. Solé-Bundó et al. (2017b) successfully demonstrated the synergistic effects of co-digesting microalgal biomass with wheat straw, resulting in a substantial 77% increase in CH4 production. The CH4 yield from co-digestion reached 0.21 L/g VSadded, a noteworthy enhancement compared to the 0.12 L/g VS achieved through mono-digestion of microalgal biomass. Additionally, efforts have been directed towards valorising algal residues generated post-digestion. Bae et al. (2011) explored the conversion of these residues into biochar, reporting a biochar yield of approximately 33% of the initial microalgal biomass dry weight. This sustainable approach contributes not only to waste reduction but also to the creation of value-added products.
Emerging technologies and approaches have been devised to overcome the challenges associated with the anaerobic digestion of microalgal biomass. To mitigate inhibition caused by lipids, researchers have explored lipid extraction from microalgal biomass prior to digestion. Alzate et al. (2014) reported a 15% increase in CH4 yield after lipid extraction, raising it from 332 mL/g VS to 382 mL/g VS. Moreover, advanced process monitoring, and control systems have been developed to ensure the stable operation of anaerobic digesters. Feng et al. (2021) utilized an automated pH control system that maintained optimal pH levels, resulting in stable reactor performance and consistent biogas production. Additionally, the development of biorefinery concepts has been a significant trend. González-Balderas et al. (2020) presented an integrated biorefinery approach that enables the recovery of lipids, proteins, and carbohydrates from microalgal biomass. This integrated system enhances resource recovery, with lipid yields of up to 73%, protein yields of up to 97% and carbohydrate yields of up to 89%. Lastly, researchers have focused on managing algal toxins, developing techniques using activated carbon to reduce the concentration of harmful algal toxins like microcystins. Song et al. (2022) effectively mitigated the inhibitory effects of these toxins on the anaerobic digestion process by reducing their concentration from 1.2 μg/L to below detection limits.
To provide a roadmap for achieving higher biogas production from microalgal biomass, future research directions should prioritize a holistic approach. This involves a synergistic integration of genetic engineering, cultivation optimization, and process engineering. Efforts should be directed towards developing high-yielding microalgal strains with tailored biochemical profiles, exploring innovative cultivation methods, and refining anaerobic digestion processes through advanced monitoring and control strategies. Moreover, collaborative interdisciplinary research, encompassing microbiology, bioprocess engineering, and genetic sciences, is pivotal for unravelling the full potential of microalgal biomass in bioenergy applications. Establishing standardized protocols for strain characterization, cultivation, and anaerobic digestion can facilitate comparative studies and the development of best practices.
8 The potential implications in a real-world context
The potential implications of the findings presented in this review bear significant weight in translating laboratory successes to real-world applications, particularly in the context of microalgal biomass utilization in anaerobic digestion for biofuel production. The synthesis of diverse studies underscores the transformative potential of microalgal biomass as a feedstock for anaerobic digestion, offering a sustainable solution for waste management and renewable energy generation. Notably, recent research by Park et al. (2011) and Xia and Murphy (2016) have demonstrated the capacity of anaerobic digestion to yield an impressive 1 kWh/kg of VS in the form of electrical energy from microalgae, accentuating the real-world feasibility of harnessing energy from this resource.
The implications extend beyond energy production, with a focus on addressing challenges associated with traditional feedstocks (Kan et al., 2023). The inherent versatility of microalgal biomass in anaerobic digestion allows for the treatment of a wide range of organic substrates, from agricultural residues to municipal solid waste (Liebetrau et al., 2019; Zamri et al., 2021). This not only contributes to reducing organic waste volumes but also mitigates greenhouse gas emissions, aligning with the growing global imperative for sustainable waste management practices. The potential for microalgal biomass to serve as a key player in the transition to a circular economy is further emphasized by its nutrient-rich composition and year-round cultivability (Calicioglu and Demirer, 2022; Kaparaju et al., 2023).
Moreover, the integration of RMB into the anaerobic digestion process presents a promising opportunity, especially in the context of wastewater treatment. Despite hurdles like low lipid content and the presence of other microorganisms, anaerobic digestion emerges as the most practical method for effectively harnessing energy from microalgal biomass (Kusmayadi et al., 2022). This finding holds crucial implications for wastewater treatment facilities, offering a sustainable approach to both waste management and energy generation. The significance of this integration is underscored by the potential to enhance the overall anaerobic digestion process, as demonstrated by recent advancements in co-digestion techniques (Vargas-Estrada et al., 2022).
9 Conclusion and future outlook
Anaerobic digestion emerges as a leading sustainable method for waste-to-energy conversion, particularly through the utilization of microalgal biomass. This process is highlighted for its environmental benefits and the advancements in the field. Through the development of effective algal strain selection, innovative pre-treatment processes, synergistic co-digestion methods, and the utilization of residual algal matter, significant enhancements in biogas production efficiency have been achieved. These improvements underscore the potential of anaerobic digestion in contributing to sustainable energy solutions. Additionally, the introduction of advanced techniques such as lipid extraction prior to digestion, improved monitoring of the process, and the adoption of integrated biorefinery concepts address previous challenges, paving the way for more efficient and effective waste-to-energy conversion practices.
Author contributions
MH: Conceptualization, Writing–original draft. MM: Formal Analysis, Writing–original draft. MU: Visualization, Writing–review and editing. ZK: Writing–review and editing. IB: Writing–review and editing. TK: Writing–review and editing.
Funding
The author(s) declare financial support was received for the research, authorship, and/or publication of this article. The authors extend their appreciation to the Deanship of Scientific Research at King Khalid University, Saudi Arabia for funding this work through the large groups project under grant number RGP2/367/44.
Conflict of interest
The authors declare that the research was conducted in the absence of any commercial or financial relationships that could be construed as a potential conflict of interest.
The author(s) declared that they were an editorial board member of Frontiers, at the time of submission. This had no impact on the peer review process and the final decision.
Publisher’s note
All claims expressed in this article are solely those of the authors and do not necessarily represent those of their affiliated organizations, or those of the publisher, the editors and the reviewers. Any product that may be evaluated in this article, or claim that may be made by its manufacturer, is not guaranteed or endorsed by the publisher.
References
Abomohra, A.E.-F., Jin, W., Tu, R., Han, S.-F., Eid, M., and Eladel, H. (2016). Microalgal biomass production as a sustainable feedstock for biodiesel: current status and perspectives. Renew. Sustain. Energy Rev. 64, 596–606. doi:10.1016/j.rser.2016.06.056
Alzate, M. E., Muñoz, R., Rogalla, F., Fdz-Polanco, F., and Pérez-Elvira, S. I. (2012). Biochemical methane potential of microalgae: influence of substrate to inoculum ratio, biomass concentration and pretreatment. Bioresour. Technol. 123, 488–494. doi:10.1016/j.biortech.2012.06.113
Alzate, M. E., Muñoz, R., Rogalla, F., Fdz-Polanco, F., and Pérez-Elvira, S. I. (2014). Biochemical methane potential of microalgae biomass after lipid extraction. Chem. Eng. J. 243, 405–410. doi:10.1016/j.cej.2013.07.076
Amin, F. R., Khalid, H., El-Mashad, H. M., Chen, C., Liu, G., and Zhang, R. (2021). Functions of bacteria and archaea participating in the bioconversion of organic waste for methane production. Sci. Total Environ. 763, 143007. doi:10.1016/j.scitotenv.2020.143007
Angelidaki, I., Ellegaard, L., Sorensen, A., and Schmidt, J. (2002). Environmental biotechnology 12133. Environment and resources DTU. Lyngby, Denmark: Danmarks Tekniske Universitet.
Aramrueang, N., Rapport, J., and Zhang, R. (2016). Effects of hydraulic retention time and organic loading rate on performance and stability of anaerobic digestion of Spirulina platensis. Biosyst. Eng. 147, 174–182. doi:10.1016/j.biosystemseng.2016.04.006
Avila, R., Carrero, E., Crivillés, E., Mercader, M., Vicent, T., and Blánquez, P. (2020). Effects of low temperature thermal pretreatments in solubility and co-digestion of waste activated sludge and microalgae mixtures. Algal Res. 50, 101965. doi:10.1016/j.algal.2020.101965
Babarro, J. M., Reiriz, M. F., and Labarta, U. (2001). Influence of preservation techniques and freezing storage time on biochemical composition and spectrum of fatty acids of Isochrysis galbana clone T-ISO. Aquac. Res. 32, 565–572. doi:10.1046/j.1365-2109.2001.00579.x
Bae, Y. J., Ryu, C., Jeon, J.-K., Park, J., Suh, D. J., Suh, Y.-W., et al. (2011). The characteristics of bio-oil produced from the pyrolysis of three marine macroalgae. Bioresour. Technol. 102, 3512–3520. doi:10.1016/j.biortech.2010.11.023
Bauer, L., Ranglová, K., Masojídek, J., Drosg, B., and Meixner, K. (2021). Digestate as sustainable nutrient source for microalgae—challenges and prospects. Appl. Sci. 11, 1056. doi:10.3390/app11031056
Bohutskyi, P., Betenbaugh, M. J., and Bouwer, E. J. (2014). The effects of alternative pretreatment strategies on anaerobic digestion and methane production from different algal strains. Bioresour. Technol. 155, 366–372. doi:10.1016/j.biortech.2013.12.095
Cabeza, C., Van Lier, J. B., and Van Der Steen, P. (2023). Effects of thermal and enzymatic pre-treatments on the solubilisation of extracellular polymeric substances (EPS) and subsequent anaerobic digestion of microalgae-bacterial biomass. Algal Res. 72, 103130. doi:10.1016/j.algal.2023.103130
Calicioglu, O., and Demirer, G. N. (2022). “Chapter 1 - role of microalgae in circular economy,” in Integrated wastewater management and valorization using algal cultures. Editors GN Demirer, and S Uludag-Demirer (Amsterdam, Netherlands: Elsevier), 1–12.
Candia-Lomeli, M., Tapia-Rodríguez, A., Morales-Ibarría, M., Razo-Flores, E., and Celis, L. B. (2022). Anaerobic digestion under alkaline conditions from thermochemical pretreated microalgal biomass. BioEnergy Res. 15, 346–356. doi:10.1007/s12155-021-10325-w
Carrere, H., Antonopoulou, G., Affes, R., Passos, F., Battimelli, A., Lyberatos, G., et al. (2016). Review of feedstock pretreatment strategies for improved anaerobic digestion: from lab-scale research to full-scale application. Bioresour. Technol. 199, 386–397. doi:10.1016/j.biortech.2015.09.007
Carrillo-Reyes, J., Barragán-Trinidad, M., and Buitrón, G. (2016). Biological pretreatments of microalgal biomass for gaseous biofuel production and the potential use of rumen microorganisms: a review. Algal Res. 18, 341–351. doi:10.1016/j.algal.2016.07.004
Carrillo-Reyes, J., Buitrón, G., Arcila, J. S., and López-Gómez, M. O. (2021). Thermophilic biogas production from microalgae-bacteria aggregates: biogas yield, community variation and energy balance. Chemosphere 275, 129898. doi:10.1016/j.chemosphere.2021.129898
Chng, L. M., Lee, K. T., and Chan, D. J. C. (2017). Synergistic effect of pretreatment and fermentation process on carbohydrate-rich Scenedesmus dimorphus for bioethanol production. Energy Convers. Manag. 141, 410–419. doi:10.1016/j.enconman.2016.10.026
Chu, F.-F., Chu, P.-N., Cai, P.-J., Li, W.-W., Lam, P. K. S., and Zeng, R. J. (2013). Phosphorus plays an important role in enhancing biodiesel productivity of Chlorella vulgaris under nitrogen deficiency. Bioresour. Technol. 134, 341–346. doi:10.1016/j.biortech.2013.01.131
Chynoweth, D. P. (2005). Renewable biomethane from land and ocean energy crops and organic wastes. HortScience HortSci 40, 283–286. doi:10.21273/hortsci.40.2.283
Ciudad, G., Rubilar, O., Azócar, L., Toro, C., Cea, M., Torres, Á., et al. (2014). Performance of an enzymatic extract in Botrycoccus braunii cell wall disruption. J. Biosci. Bioeng. 117, 75–80. doi:10.1016/j.jbiosc.2013.06.012
Colling Klein, B., Bonomi, A., and Maciel Filho, R. (2018). Integration of microalgae production with industrial biofuel facilities: a critical review. Renew. Sustain. Energy Rev. 82, 1376–1392. doi:10.1016/j.rser.2017.04.063
Dasa, K. T., Westman, S. Y., Millati, R., Cahyanto, M. N., Taherzadeh, M. J., and Niklasson, C. (2016). Inhibitory effect of long-chain fatty acids on biogas production and the protective effect of membrane bioreactor. BioMed Res. Int. 2016, 1–9. doi:10.1155/2016/7263974
Demirbas, A. (2010). Use of algae as biofuel sources. Energy Convers. Manag. 51, 2738–2749. doi:10.1016/j.enconman.2010.06.010
Diltz, R., and Pullammanappallil, P. (2013). Biofuels from algae liquid. Gaseous Solid Biofuels Convers. Tech. 14, 432–449.
Doğan-Subaşı, E., and Demirer, G. N. (2016). Anaerobic digestion of microalgal (Chlorella vulgaris) biomass as a source of biogas and biofertilizer. Environmental Progress & Sustainable Energy 35, 936–941.
Efremenko, E. N., Nikolskaya, A. B., Lyagin, I. V., Senko, O. V., Makhlis, T. A., Stepanov, N. A., et al. (2012). Production of biofuels from pretreated microalgae biomass by anaerobic fermentation with immobilized Clostridium acetobutylicum cells. Bioresour. Technol. 114, 342–348. doi:10.1016/j.biortech.2012.03.049
Egesa, D., and Plucinski, P. (2024). Efficient extraction of lipids from magnetically separated microalgae using ionic liquids and their transesterification to biodiesel. Biomass Convers. Biorefinery 14, 419–434. doi:10.1007/s13399-022-02377-5
Ehimen, E. A., Sun, Z. F., Carrington, C. G., Birch, E. J., and Eaton-Rye, J. J. (2011). Anaerobic digestion of microalgae residues resulting from the biodiesel production process. Appl. Energy 88, 3454–3463. doi:10.1016/j.apenergy.2010.10.020
Ellegaard, M., and Ribeiro, S. (2018). The long-term persistence of phytoplankton resting stages in aquatic ‘seed banks. Biol. Rev. 93, 166–183. doi:10.1111/brv.12338
Feng, K., Wang, Q., Li, H., Du, X., and Zhang, Y. (2021). Microbial mechanism of enhancing methane production from anaerobic digestion of food waste via phase separation and pH control. J. Environ. Manag. 288, 112460. doi:10.1016/j.jenvman.2021.112460
González-Balderas, R. M., Velásquez-Orta, S. B., Valdez-Vazquez, I., and Orta Ledesma, M. T. (2020). Intensified recovery of lipids, proteins, and carbohydrates from wastewater-grown microalgae Desmodesmus sp. by using ultrasound or ozone. Ultrason. Sonochemistry 62, 104852. doi:10.1016/j.ultsonch.2019.104852
González-Fernández, C., and Ballesteros, M. (2013). Microalgae autoflocculation: an alternative to high-energy consuming harvesting methods. J. Appl. Phycol. 25, 991–999. doi:10.1007/s10811-012-9957-3
González-Fernández, C., Sialve, B., Bernet, N., and Steyer, J.-P. (2012a). Impact of microalgae characteristics on their conversion to biofuel. Part II: focus on biomethane production. Biofuels, Bioprod. Biorefining 6, 205–218. doi:10.1002/bbb.337
González-Fernández, C., Sialve, B., Bernet, N., and Steyer, J. P. (2012b). Comparison of ultrasound and thermal pretreatment of Scenedesmus biomass on methane production. Bioresour. Technol. 110, 610–616. doi:10.1016/j.biortech.2012.01.043
González-Fernández, C., Sialve, B., Bernet, N., and Steyer, J. P. (2012c). Thermal pretreatment to improve methane production of Scenedesmus biomass. Biomass Bioenergy 40, 105–111. doi:10.1016/j.biombioe.2012.02.008
Gonzalez-Fernandez, C., Sialve, B., and Molinuevo-Salces, B. (2015). Anaerobic digestion of microalgal biomass: challenges, opportunities and research needs. Bioresour. Technol. 198, 896–906. doi:10.1016/j.biortech.2015.09.095
Grobbelaar, J. U. (2010). Microalgal biomass production: challenges and realities. Photosynth. Res. 106, 135–144. doi:10.1007/s11120-010-9573-5
Hallenbeck, P. C., Grogger, M., Mraz, M., and Veverka, D. (2016). Solar biofuels production with microalgae. Appl. Energy 179, 136–145. doi:10.1016/j.apenergy.2016.06.024
Harith, Z., Yusoff, F., Shariff, M., and Ariff, A. (2010). Effect of different separation techniques and storage temperatures on the viability of marine microalgae, Chaetoceros calcitrans, during storage. Biotechnology 9, 387–391. doi:10.3923/biotech.2010.387.391
Harun, R., Singh, M., Forde, G. M., and Danquah, M. K. (2010). Bioprocess engineering of microalgae to produce a variety of consumer products. Renew. Sustain. Energy Rev. 14, 1037–1047. doi:10.1016/j.rser.2009.11.004
Holmes, D. E., and Smith, J. A. (2016). “Chapter one - biologically produced methane as a renewable energy source,” in Advances in applied microbiology. Editors S Sariaslani, and G Michael Gadd (United States: Academic Press), 1–61.
Hu, Y., Kumar, M., Wang, Z., Zhan, X., and Stengel, D. B. (2021). Filamentous microalgae as an advantageous co-substrate for enhanced methane production and digestate dewaterability in anaerobic co-digestion of pig manure. Waste Manag. 119, 399–407. doi:10.1016/j.wasman.2020.10.041
Inglesby, A. E., and Fisher, A. C. (2012). Enhanced methane yields from anaerobic digestion of Arthrospira maxima biomass in an advanced flow-through reactor with an integrated recirculation loop microbial fuel cell. Energy and Environ. Sci. 5, 7996–8006. doi:10.1039/c2ee21659k
Iyovo, G. D., Du, G., and Chen, J. (2010). Sustainable bioenergy bioprocessing: biomethane production, digestate as biofertilizer and as supplemental feed in algae cultivation to promote algae biofuel commercialization. J. Microb. Biochem. Technol. 2, 100–106. doi:10.4172/1948-5948.1000032
Jankowska, E., Sahu, A. K., and Oleskowicz-Popiel, P. (2017). Biogas from microalgae: review on microalgae's cultivation, harvesting and pretreatment for anaerobic digestion. Renew. Sustain. Energy Rev. 75, 692–709. doi:10.1016/j.rser.2016.11.045
Josephine, A., Kumar, T. S., Surendran, B., Rajakumar, S., Kirubagaran, R., and Dharani, G. (2022). Evaluating the effect of various environmental factors on the growth of the marine microalgae, Chlorella vulgaris. Front. Mar. Sci. 9. doi:10.3389/fmars.2022.954622
Kan, Y., Li, J., Zhang, S., and Gao, Z. (2023). Novel bridge assistance strategy for tailoring crosslinking networks within soybean-meal-based biocomposites to balance mechanical and biodegradation properties. Chem. Eng. J. 472, 144858. doi:10.1016/j.cej.2023.144858
Kaparaju, P., Sarker, N. K., Mukherjee, T., and Herat, S. (2023). “Circular-BioEconomy through anaerobic digestion,” in Circular economy adoption: catalysing decarbonisation through policy instruments. Editors SK Ghosh, and SK Ghosh (Singapore: Springer Nature Singapore), 449–468.
Khalid, A., Arshad, M., Anjum, M., Mahmood, T., and Dawson, L. (2011). The anaerobic digestion of solid organic waste. Waste Manag. 31, 1737–1744. doi:10.1016/j.wasman.2011.03.021
Khoo, K. S., Ahmad, I., Chew, K. W., Iwamoto, K., Bhatnagar, A., and Show, P. L. (2023). Enhanced microalgal lipid production for biofuel using different strategies including genetic modification of microalgae: a review. Prog. Energy Combust. Sci. 96, 101071. doi:10.1016/j.pecs.2023.101071
Kinnunen, V., Craggs, R., and Rintala, J. (2014). Influence of temperature and pretreatments on the anaerobic digestion of wastewater grown microalgae in a laboratory-scale accumulating-volume reactor. Water Res. 57, 247–257. doi:10.1016/j.watres.2014.03.043
Kirk, D. M., and Gould, M. C. (2020). “Chapter 17 - bioenergy and anaerobic digestion,” in Bioenergy Editor A Dahiya. Second Edition (United States: Academic Press), 335–360.
Kisielewska, M., Dębowski, M., Zieliński, M., Kazimierowicz, J., Quattrocelli, P., and Bordiean, A. (2022). Effects of liquid digestate treatment on sustainable microalgae biomass production. BioEnergy Res. 15, 357–370. doi:10.1007/s12155-021-10251-x
Kusmayadi, A., Lu, P.-H., Huang, C.-Y., Leong, Y. K., Yen, H.-W., and Chang, J.-S. (2022). Integrating anaerobic digestion and microalgae cultivation for dairy wastewater treatment and potential biochemicals production from the harvested microalgal biomass. Chemosphere 291, 133057. doi:10.1016/j.chemosphere.2021.133057
Lee, K., Chantrasakdakul, P., Kim, D., Kong, M., and Park, K. Y. (2014). Ultrasound pretreatment of filamentous algal biomass for enhanced biogas production. Waste Manag. 34, 1035–1040. doi:10.1016/j.wasman.2013.10.012
Li, Y., Xu, H., Yi, X., Zhao, Y., Jin, F., Chen, L., et al. (2021). Study of two-phase anaerobic digestion of corn stover: focusing on the conversion of volatile fatty acids and microbial characteristics in UASB reactor. Industrial Crops Prod. 160, 113097. doi:10.1016/j.indcrop.2020.113097
Liebetrau, J., Sträuber, H., Kretzschmar, J., Denysenko, V., and Nelles, M. (2019). “Anaerobic digestion,” in Biorefineries. Editors K. Wagemann, and N. Tippkötter (Cham: Springer International Publishing), 281–299.
Luna-Avelar, K. D., Barrena, R., Font, X., Sánchez, A., Santos-Ballardo, D. U., Germán-Báez, L. J., et al. (2021). A preliminary assessment of anaerobic co-digestion potential of mango and microalgal residue biomass using a design of experiments approach: effect of thermal, physical and biological pretreatments. Food Bioprod. Process. 128, 143–152. doi:10.1016/j.fbp.2021.04.015
Mahajan, N., and Gupta, P. (2015). New insights into the microbial degradation of polyurethanes. RSC Adv. 5, 41839–41854. doi:10.1039/c5ra04589d
Mahdy, A., Ballesteros, M., and González-Fernández, C. (2016a). Enzymatic pretreatment of Chlorella vulgaris for biogas production: influence of urban wastewater as a sole nutrient source on macromolecular profile and biocatalyst efficiency. Bioresour. Technol. 199, 319–325. doi:10.1016/j.biortech.2015.08.080
Mahdy, A., Mendez, L., Ballesteros, M., and González-Fernández, C. (2014a). Autohydrolysis and alkaline pretreatment effect on Chlorella vulgaris and Scenedesmus sp. methane production. Energy 78, 48–52. doi:10.1016/j.energy.2014.05.052
Mahdy, A., Mendez, L., Ballesteros, M., and González-Fernández, C. (2014b). Enhanced methane production of Chlorella vulgaris and Chlamydomonas reinhardtii by hydrolytic enzymes addition. Energy Convers. Manag. 85, 551–557. doi:10.1016/j.enconman.2014.04.097
Mahdy, A., Mendez, L., Ballesteros, M., and González-Fernández, C. (2015a). Algaculture integration in conventional wastewater treatment plants: anaerobic digestion comparison of primary and secondary sludge with microalgae biomass. Bioresour. Technol. 184, 236–244. doi:10.1016/j.biortech.2014.09.145
Mahdy, A., Mendez, L., Ballesteros, M., and González-Fernández, C. (2015b). Protease pretreated Chlorella vulgaris biomass bioconversion to methane via semi-continuous anaerobic digestion. Fuel 158, 35–41. doi:10.1016/j.fuel.2015.04.052
Mahdy, A., Mendez, L., Tomás-Pejó, E., Del Mar Morales, M., Ballesteros, M., and González-Fernández, C. (2016b). Influence of enzymatic hydrolysis on the biochemical methane potential of Chlorella vulgaris and Scenedesmus sp. J. Chem. Technol. Biotechnol. 91, 1299–1305. doi:10.1002/jctb.4722
Mairet, F., Bernard, O., Cameron, E., Ras, M., Lardon, L., Steyer, J.-P., et al. (2012). Three-reaction model for the anaerobic digestion of microalgae. Biotechnol. Bioeng. 109, 415–425. doi:10.1002/bit.23350
Malik, S., Shahid, A., Betenbaugh, M. J., Liu, C.-G., and Mehmood, M. A. (2022). A novel wastewater-derived cascading algal biorefinery route for complete valorization of the biomass to biodiesel and value-added bioproducts. Energy Convers. Manag. 256, 115360. doi:10.1016/j.enconman.2022.115360
Maltsev, Y., Maltseva, K., Kulikovskiy, M., and Maltseva, S. (2021). Influence of light conditions on microalgae growth and content of lipids, carotenoids, and fatty acid composition. Biology 10, 1060. doi:10.3390/biology10101060
Manyi-Loh, C. E., Mamphweli, S. N., Meyer, E. L., and Okoh, A. I. (2019). Microbial anaerobic digestion: process dynamics and implications from the renewable energy, environmental and agronomy perspectives. Int. J. Environ. Sci. Technol. 16, 3913–3934. doi:10.1007/s13762-019-02380-w
Martínez-Gutiérrez, E. (2018). Biogas production from different lignocellulosic biomass sources: advances and perspectives. 3 Biotech. 8, 233. doi:10.1007/s13205-018-1257-4
Mcpherson, A., and Cudney, B. (2014). Optimization of crystallization conditions for biological macromolecules. Acta Crystallogr. Sect. F. Struct. Biol. Commun. 70, 1445–1467. doi:10.1107/s2053230x14019670
Mendez, L., Mahdy, A., Ballesteros, M., and González-Fernández, C. (2015). Biomethane production using fresh and thermally pretreated Chlorella vulgaris biomass: a comparison of batch and semi-continuous feeding mode. Ecol. Eng. 84, 273–277. doi:10.1016/j.ecoleng.2015.09.056
Mendez, L., Mahdy, A., Demuez, M., Ballesteros, M., and González-Fernández, C. (2014). Effect of high pressure thermal pretreatment on Chlorella vulgaris biomass: organic matter solubilisation and biochemical methane potential. Fuel 117, 674–679. doi:10.1016/j.fuel.2013.09.032
Mendez, L., Mahdy, A., Timmers, R. A., Ballesteros, M., and González-Fernández, C. (2013). Enhancing methane production of Chlorella vulgaris via thermochemical pretreatments. Bioresour. Technol. 149, 136–141. doi:10.1016/j.biortech.2013.08.136
Menzel, T., Neubauer, P., and Junne, S. (2020). Role of microbial hydrolysis in anaerobic digestion. Energies 13, 5555. doi:10.3390/en13215555
Miao, G., Chuanming, Z., Leiming, Y., Xunlai, Y., and Chunzhao, W. (2010). Cell wall ultrastructures of the proterozoicacritarchleiosphaeridiaasperata and their implications for biological affinity. Sci. China Earth Sci. 53, 1750–1755. doi:10.1007/s11430-010-4083-z
Milano, J., Ong, H. C., Masjuki, H. H., Chong, W. T., Lam, M. K., Loh, P. K., et al. (2016). Microalgae biofuels as an alternative to fossil fuel for power generation. Renew. Sustain. Energy Rev. 58, 180–197. doi:10.1016/j.rser.2015.12.150
Munisamy Sambasivam, K., Kuppan, P., Shashirekha, V., Tamilarasan, K., and Abinandan, S. (2023). Cascading utilization of residual microalgal biomass: sustainable strategies for energy, environmental and value-added product applications. Bioresour. Technol. Rep. 23, 101588. doi:10.1016/j.biteb.2023.101588
Mussgnug, J. H., Klassen, V., Schlüter, A., and Kruse, O. (2010). Microalgae as substrates for fermentative biogas production in a combined biorefinery concept. J. Biotechnol. 150, 51–56. doi:10.1016/j.jbiotec.2010.07.030
Neves, V. T. D. C., Sales, E. A., and Perelo, L. W. (2016). Influence of lipid extraction methods as pre-treatment of microalgal biomass for biogas production. Renew. Sustain. Energy Rev. 59, 160–165. doi:10.1016/j.rser.2015.12.303
Niccolai, A., Chini Zittelli, G., Rodolfi, L., Biondi, N., and Tredici, M. R. (2019). Microalgae of interest as food source: biochemical composition and digestibility. Algal Res. 42, 101617. doi:10.1016/j.algal.2019.101617
Nwokolo, N. L., and Enebe, M. C. (2022). An insight on the contributions of microbial communities and process parameters in enhancing biogas production. Biomass Convers. Biorefinery 14, 1549–1565. doi:10.1007/s13399-022-02580-4
Pandey, A., Negi, S., Binod, P., and Larroche, C. (2014). Pretreatment of biomass: processes and technologies. United States: Academic Press.
Park, J. B. K., Craggs, R. J., and Shilton, A. N. (2011). Wastewater treatment high rate algal ponds for biofuel production. Bioresour. Technol. 102, 35–42. doi:10.1016/j.biortech.2010.06.158
Passos, F., Carretero, J., and Ferrer, I. (2015). Comparing pretreatment methods for improving microalgae anaerobic digestion: thermal, hydrothermal, microwave and ultrasound. Chem. Eng. J. 279, 667–672. doi:10.1016/j.cej.2015.05.065
Passos, F., and Ferrer, I. (2014). Microalgae conversion to biogas: thermal pretreatment contribution on net energy production. Environ. Sci. Technol. 48, 7171–7178. doi:10.1021/es500982v
Passos, F., and Ferrer, I. (2015). Influence of hydrothermal pretreatment on microalgal biomass anaerobic digestion and bioenergy production. Water Res. 68, 364–373. doi:10.1016/j.watres.2014.10.015
Passos, F., Hom-Diaz, A., Blanquez, P., Vicent, T., and Ferrer, I. (2016). Improving biogas production from microalgae by enzymatic pretreatment. Bioresour. Technol. 199, 347–351. doi:10.1016/j.biortech.2015.08.084
Passos, F., Uggetti, E., Carrère, H., and Ferrer, I. (2014). Pretreatment of microalgae to improve biogas production: a review. Bioresour. Technol. 172, 403–412. doi:10.1016/j.biortech.2014.08.114
Polakovičová, G., Kušnír, P., Nagyová, S., and Mikulec, J. (2012). “Process integration of algae production and anaerobic digestion,” in 15th international conference on process integration, modelling and: Citeseer, Clermont-Ferrand, France, August 30-September 2, 2011.
Prajapati, S. K., Malik, A., and Vijay, V. K. (2014). Comparative evaluation of biomass production and bioenergy generation potential of Chlorella spp. through anaerobic digestion. Appl. Energy 114, 790–797. doi:10.1016/j.apenergy.2013.08.021
Pugazhendi, A., Jamal, M. T., Al-Mur, B. A., Jeyakumar, R. B., and Kumar, G. (2022). Macroalgae (Ulva reticulata) derived biohydrogen recovery through mild surfactant induced energy and cost efficient dispersion pretreatment technology. Chemosphere 288, 132463. doi:10.1016/j.chemosphere.2021.132463
Ramos-Suárez, J. L., and Carreras, N. (2014). Use of microalgae residues for biogas production. Chem. Eng. J. 242, 86–95. doi:10.1016/j.cej.2013.12.053
Ras, M., Lardon, L., Bruno, S., Bernet, N., and Steyer, J.-P. (2011). Experimental study on a coupled process of production and anaerobic digestion of Chlorella vulgaris. Bioresour. Technol. 102, 200–206. doi:10.1016/j.biortech.2010.06.146
Rashid, N., Rehman, M. S. U., and Han, J.-I. (2013). Recycling and reuse of spent microalgal biomass for sustainable biofuels. Biochem. Eng. J. 75, 101–107. doi:10.1016/j.bej.2013.04.001
Rincón-Pérez, J., Celis, L. B., Morales, M., Alatriste-Mondragón, F., Tapia-Rodríguez, A., and Razo-Flores, E. (2021). Improvement of methane production at alkaline and neutral pH from anaerobic co-digestion of microalgal biomass and cheese whey. Biochem. Eng. J. 169, 107972. doi:10.1016/j.bej.2021.107972
Rodriguez, C., Alaswad, A., Mooney, J., Prescott, T., and Olabi, A. G. (2015). Pre-treatment techniques used for anaerobic digestion of algae. Fuel Process. Technol. 138, 765–779. doi:10.1016/j.fuproc.2015.06.027
Ruggaber, T. P., and Talley, J. W. (2006). Enhancing bioremediation with enzymatic processes: a review. Pract. Periodical Hazard. Toxic, Radioact. Waste Manag. 10, 73–85. doi:10.1061/(asce)1090-025x(2006)10:2(73)
Ruiz, H. A., Thomsen, M. H., and Trajano, H. L. (2017). Hydrothermal processing in biorefineries. Production of bioethanol and high added-value compounds of second and third generation biomass. Berlin, Germany: Springer.
Safi, C., Zebib, B., Merah, O., Pontalier, P.-Y., and Vaca-Garcia, C. (2014). Morphology, composition, production, processing and applications of Chlorella vulgaris: a review. Renew. Sustain. Energy Rev. 35, 265–278. doi:10.1016/j.rser.2014.04.007
Saharan, B. S., Sharma, D., Sahu, R., Sahin, O., and Warren, A. (2013). Towards algal biofuel production: a concept of green bio energy development. Innov. Romanian Food Biotechnol. 12, 1.
Santos, N. O., Oliveira, S. M., Alves, L. C., and Cammarota, M. C. (2014). Methane production from marine microalgae Isochrysis galbana. Bioresour. Technol. 157, 60–67. doi:10.1016/j.biortech.2014.01.091
Sayedin, F., Kermanshahi-Pour, A., He, Q. S., Tibbetts, S. M., Lalonde, C. G. E., and Brar, S. K. (2020). Microalgae cultivation in thin stillage anaerobic digestate for nutrient recovery and bioproduct production. Algal Res. 47, 101867. doi:10.1016/j.algal.2020.101867
Scarponi, P., Caminiti, V., Bravi, M., Izzo, F. C., and Cavinato, C. (2024). Coupling anaerobic co-digestion of winery waste and waste activated sludge with a microalgae process: optimization of a semi-continuous system. Waste Manag. 174, 300–309. doi:10.1016/j.wasman.2023.12.004
Schenk, P. M., Thomas-Hall, S. R., Stephens, E., Marx, U. C., Mussgnug, J. H., Posten, C., et al. (2008). Second generation biofuels: high-efficiency microalgae for biodiesel production. BioEnergy Res. 1, 20–43. doi:10.1007/s12155-008-9008-8
Schwede, S., Rehman, Z.-U., Gerber, M., Theiss, C., and Span, R. (2013). Effects of thermal pretreatment on anaerobic digestion of Nannochloropsis salina biomass. Bioresour. Technol. 143, 505–511. doi:10.1016/j.biortech.2013.06.043
Sepulveda, C., Gómez, C., El Bahraoui, N., and Acién, G. (2019). Comparative evaluation of microalgae strains for CO2 capture purposes. J. CO2 Util. 30, 158–167. doi:10.1016/j.jcou.2019.02.004
Sharma, S., Reena Kumar, A., and Mittal, P. (2013). “Recent progress on microbial metabolic engineering for the conversion of lignocellulose waste for biofuel production,” in Biofuels production, 119–145.
Siddiki, S. Y. A., Mofijur, M., Kumar, P. S., Ahmed, S. F., Inayat, A., Kusumo, F., et al. (2022). Microalgae biomass as a sustainable source for biofuel, biochemical and biobased value-added products: an integrated biorefinery concept. Fuel 307, 121782. doi:10.1016/j.fuel.2021.121782
Solé-Bundó, M., Carrère, H., Garfí, M., and Ferrer, I. (2017a). Enhancement of microalgae anaerobic digestion by thermo-alkaline pretreatment with lime (CaO). Algal Res. 24, 199–206. doi:10.1016/j.algal.2017.03.025
Solé-Bundó, M., Eskicioglu, C., Garfí, M., Carrère, H., and Ferrer, I. (2017b). Anaerobic co-digestion of microalgal biomass and wheat straw with and without thermo-alkaline pretreatment. Bioresour. Technol. 237, 89–98. doi:10.1016/j.biortech.2017.03.151
Solé-Bundó, M., Passos, F., Romero-Güiza, M. S., Ferrer, I., and Astals, S. (2019). Co-digestion strategies to enhance microalgae anaerobic digestion: a review. Renew. Sustain. Energy Rev. 112, 471–482. doi:10.1016/j.rser.2019.05.036
Song, K., Li, Z., Li, L., Zhao, X., Deng, M., Zhou, X., et al. (2022). Methane production from peroxymonosulfate pretreated algae biomass: insights into microbial mechanisms, microcystin detoxification and heavy metal partitioning behavior. Sci. Total Environ. 834, 155500. doi:10.1016/j.scitotenv.2022.155500
Srinuanpan, S., Cheirsilp, B., Kitcha, W., and Prasertsan, P. (2017). Strategies to improve methane content in biogas by cultivation of oleaginous microalgae and the evaluation of fuel properties of the microalgal lipids. Renew. Energy 113, 1229–1241. doi:10.1016/j.renene.2017.06.108
Srivastava, R., Basu, S., and Kumar, R. (2021). Phosphorus starvation response dynamics and management in plants for sustainable agriculture. J. Plant Biochem. Biotechnol. 30, 829–847. doi:10.1007/s13562-021-00715-8
Stiles, W. a.V., Styles, D., Chapman, S. P., Esteves, S., Bywater, A., Melville, L., et al. (2018). Using microalgae in the circular economy to valorise anaerobic digestate: challenges and opportunities. Bioresour. Technol. 267, 732–742. doi:10.1016/j.biortech.2018.07.100
Timira, V., Meki, K., Li, Z., Lin, H., Xu, M., and Pramod, S. N. (2022). A comprehensive review on the application of novel disruption techniques for proteins release from microalgae. Crit. Rev. Food Sci. Nutr. 62, 4309–4325. doi:10.1080/10408398.2021.1873734
Tong, C. Y., Honda, K., and Derek, C. J. C. (2023). A review on microalgal-bacterial co-culture: the multifaceted role of beneficial bacteria towards enhancement of microalgal metabolite production. Environ. Res. 228, 115872. doi:10.1016/j.envres.2023.115872
Valigore, J. M., Gostomski, P. A., Wareham, D. G., and O’sullivan, A. D. (2012). Effects of hydraulic and solids retention times on productivity and settleability of microbial (microalgal-bacterial) biomass grown on primary treated wastewater as a biofuel feedstock. Water Res. 46, 2957–2964. doi:10.1016/j.watres.2012.03.023
Vargas-Estrada, L., Longoria, A., Arenas, E., Moreira, J., Okoye, P. U., Bustos-Terrones, Y., et al. (2022). A review on current trends in biogas production from microalgae biomass and microalgae waste by anaerobic digestion and Co-digestion. BioEnergy Res. 15, 77–92. doi:10.1007/s12155-021-10276-2
Vergara-Fernández, A., Vargas, G., Alarcón, N., and Velasco, A. (2008). Evaluation of marine algae as a source of biogas in a two-stage anaerobic reactor system. Biomass Bioenergy 32, 338–344. doi:10.1016/j.biombioe.2007.10.005
Verma, R., and Srivastava, A. (2018). Carbon dioxide sequestration and its enhanced utilization by photoautotroph microalgae. Environ. Dev. 27, 95–106. doi:10.1016/j.envdev.2018.07.004
Wang, M., Lee, E., Dilbeck, M. P., Liebelt, M., Zhang, Q., and Ergas, S. J. (2017). Thermal pretreatment of microalgae for biomethane production: experimental studies, kinetics and energy analysis. J. Chem. Technol. Biotechnol. 92, 399–407. doi:10.1002/jctb.5018
Wang, M., Sahu, A. K., Rusten, B., and Park, C. (2013). Anaerobic co-digestion of microalgae Chlorella sp. and waste activated sludge. Bioresour. Technol. 142, 585–590. doi:10.1016/j.biortech.2013.05.096
Wang, Y., Guo, W., Cheng, C.-L., Ho, S.-H., Chang, J.-S., and Ren, N. (2016). Enhancing bio-butanol production from biomass of Chlorella vulgaris JSC-6 with sequential alkali pretreatment and acid hydrolysis. Bioresour. Technol. 200, 557–564. doi:10.1016/j.biortech.2015.10.056
Wargacki, A. J., Leonard, E., Win, M. N., Regitsky, D. D., Santos, C. N. S., Kim, P. B., et al. (2012). An engineered microbial platform for direct biofuel production from Brown macroalgae. Science 335, 308–313. doi:10.1126/science.1214547
Wirth, R., Lakatos, G., Böjti, T., Maróti, G., Bagi, Z., Rákhely, G., et al. (2018). Anaerobic gaseous biofuel production using microalgal biomass – a review. Anaerobe 52, 1–8. doi:10.1016/j.anaerobe.2018.05.008
Xia, A., and Murphy, J. D. (2016). Microalgal cultivation in treating liquid digestate from biogas systems. Trends Biotechnol. 34, 264–275. doi:10.1016/j.tibtech.2015.12.010
Xiao, C., Fu, Q., Liao, Q., Huang, Y., Xia, A., Chen, H., et al. (2020). Life cycle and economic assessments of biogas production from microalgae biomass with hydrothermal pretreatment via anaerobic digestion. Renew. Energy 151, 70–78. doi:10.1016/j.renene.2019.10.145
Yadvika, S., Sreekrishnan, T. R., Kohli, S., and Rana, V. (2004). Enhancement of biogas production from solid substrates using different techniques––a review. Bioresour. Technol. 95, 1–10. doi:10.1016/j.biortech.2004.02.010
Yen, H.-W., and Brune, D. E. (2007). Anaerobic co-digestion of algal sludge and waste paper to produce methane. Bioresour. Technol. 98, 130–134. doi:10.1016/j.biortech.2005.11.010
Yu, N., Dieu, L. T. J., Harvey, S., and Lee, D.-Y. (2015). Optimization of process configuration and strain selection for microalgae-based biodiesel production. Bioresour. Technol. 193, 25–34. doi:10.1016/j.biortech.2015.05.101
Yuan, X., Shi, X., Zhang, D., Qiu, Y., Guo, R., and Wang, L. (2011). Biogas production and microcystin biodegradation in anaerobic digestion of blue algae. Energy and Environ. Sci. 4, 1511–1515. doi:10.1039/c0ee00452a
Zamalloa, C., De Vrieze, J., Boon, N., and Verstraete, W. (2012). Anaerobic digestibility of marine microalgae Phaeodactylum tricornutum in a lab-scale anaerobic membrane bioreactor. Appl. Microbiol. Biotechnol. 93, 859–869. doi:10.1007/s00253-011-3624-5
Zamri, M. F. M. A., Hasmady, S., Akhiar, A., Ideris, F., Shamsuddin, A. H., Mofijur, M., et al. (2021). A comprehensive review on anaerobic digestion of organic fraction of municipal solid waste. Renew. Sustain. Energy Rev. 137, 110637. doi:10.1016/j.rser.2020.110637
Zepka, L. Q., Jacob-Lopes, E., Goldbeck, R., and Queiroz, M. I. (2008). Production and biochemical profile of the microalgae Aphanothece microscopica Nägeli submitted to different drying conditions. Chem. Eng. Process. Process Intensif. 47, 1305–1310. doi:10.1016/j.cep.2007.04.013
Zhang, L., Li, F., Kuroki, A., Loh, K.-C., Wang, C.-H., Dai, Y., et al. (2020). Methane yield enhancement of mesophilic and thermophilic anaerobic co-digestion of algal biomass and food waste using algal biochar: semi-continuous operation and microbial community analysis. Bioresour. Technol. 302, 122892. doi:10.1016/j.biortech.2020.122892
Zhao, B., Ma, J., Zhao, Q., Laurens, L., Jarvis, E., Chen, S., et al. (2014). Efficient anaerobic digestion of whole microalgae and lipid-extracted microalgae residues for methane energy production. Bioresour. Technol. 161, 423–430. doi:10.1016/j.biortech.2014.03.079
Zhen, G., Lu, X., Kato, H., Zhao, Y., and Li, Y.-Y. (2017). Overview of pretreatment strategies for enhancing sewage sludge disintegration and subsequent anaerobic digestion: current advances, full-scale application and future perspectives. Renew. Sustain. Energy Rev. 69, 559–577. doi:10.1016/j.rser.2016.11.187
Keywords: biogas production, sustainable energy, algal strain selection, co-digestion, algal toxins, resource recovery
Citation: Hasan MM, Mofijur M, Uddin MN, Kabir Z, Badruddin IA and Khan TMY (2024) Insights into anaerobic digestion of microalgal biomass for enhanced energy recovery. Front. Energy Res. 12:1355686. doi: 10.3389/fenrg.2024.1355686
Received: 14 December 2023; Accepted: 07 February 2024;
Published: 22 February 2024.
Edited by:
Abdul-Sattar Nizami, Government College University, Lahore, PakistanReviewed by:
Muhammad Farooq, University of Engineering and Technology, Lahore, PakistanAnees Ahmad, Government College University, Pakistan
Ghulam Murshid, Sultan Qaboos University, Oman
Copyright © 2024 Hasan, Mofijur, Uddin, Kabir, Badruddin and Khan. This is an open-access article distributed under the terms of the Creative Commons Attribution License (CC BY). The use, distribution or reproduction in other forums is permitted, provided the original author(s) and the copyright owner(s) are credited and that the original publication in this journal is cited, in accordance with accepted academic practice. No use, distribution or reproduction is permitted which does not comply with these terms.
*Correspondence: M. Mofijur, TWRNb2ZpanVyLlJhaG1hbkB1dHMuZWR1LmF1