- 1Department of Chemical Engineering, Delft University of Technology, Delft, Netherlands
- 2Department of Engineering Systems and Services, Delft University of Technology, Delft, Netherlands
- 3Department of Process and Energy, Delft University of Technology, Delft, Netherlands
Despite the huge efforts devoted to the development of the electrochemical reduction of CO2 (ECO2R) in the past decade, still many challenges are present, hindering further approaches to industrial applications. This paper gives a perspective on these challenges from a Process Systems Engineering (PSE) standpoint, while at the same time highlighting the opportunities for advancements in the field in the European context. The challenges are connected with: the coupling of these processes with renewable electricity generation; the feedstock (in particular CO2); the processes itself; and the different products that can be obtained. PSE can determine the optimal interactions among the components of such systems, allowing educated decision making in designing the best process configurations under uncertainty and constrains. The opportunities, on the other hand, stem from a stronger collaboration between the PSE and the experimental communities, from the possibility of integrating ECO2R into existing industrial productions and from process-wide optimisation studies, encompassing the whole production cycle of the chemicals to exploit possible synergies.
1 Introduction
The electrochemical reduction of CO2 (ECO2R) has risen to prominence within the scientific community in the past decade, as this technology may play a role in improving the sustainability of the process industry within the broader fields of carbon capture and utilization (CCU) and electrification. Given the infancy of these technologies, the main research directions have been on the experimental improvement of the electrolytic cell performance via optimisation of catalytic materials, membranes and cell design (Chang et al., 2023; Farooqi et al., 2023). Parallel to this, techno-economic and life cycle assessments have become common (Somoza-Tornos et al., 2021), based on process designs and simulations taking into account the complete process centred around the electrolytic cell. These studies include upstream and downstream processes (USP and DSP, respectively), usually assuming that the cell performance observed in the laboratory is analogous to the electrolyser performance in an industrial size and context, which may not be the case in reality.
The European Union has the goal of positioning itself at the forefront of the green transition, for example, via the REPowerEU Plan (European Commission, 2022). Alongside ambitious plans for green hydrogen production, research on ECO2R is taking place in a multitude of organisations, both academic and industrial, across Europe (for example, groups at ETH Zurich, Imperial College London, TU Delft, and companies like Avantium, Sunfire and Topsoe). A strong expansion of renewable generation in Europe is envisioned, revolving around offshore wind power in the North Sea (European Commission, 2020).
Nevertheless, many challenges still have to be tackled to ensure industrial implementation and the tools of Process Systems Engineering (PSE) could be used to help shed light on still unanswered questions underlying these challenges (see Figure 1). Several conditions (for example, available and cheap low carbon electricity, mature and efficient carbon capture technologies, long term stable performance of electrolysers (De Luna et al., 2019)) must be present to unlock the potential of ECO2R to mitigate the effects of climate change by decreasing the emissions of the process industry and converting CO2 into products. The path forward will be shaped depending on how the existing challenges will be addressed and which opportunities will be taken in the future.
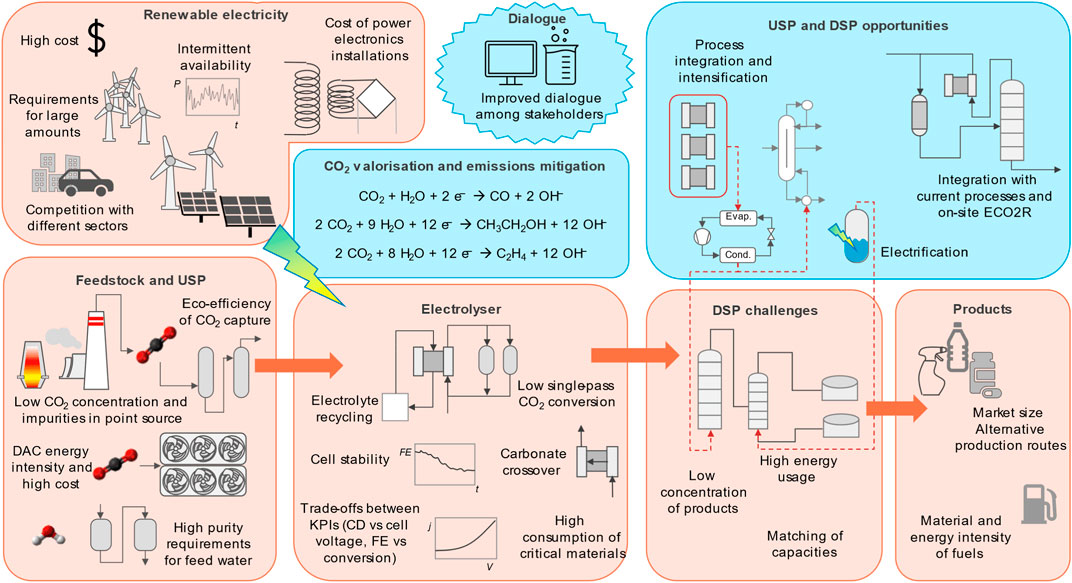
FIGURE 1. Overview of the challenges (with orange background) and opportunities (light blue background) for electrochemical reduction of CO2.
Comparing ECO2R to water electrolysis, it is possible to observe several additional difficulties posed by CO2, on top of inheriting the ones already present in green hydrogen production: for example, CO2 is a gaseous species, with limited solubility in water, and its reduction competes with the hydrogen evolution reaction itself, which has to be contained. It is worth noting that the scope of this perspective paper is limited to direct CO2 electroreduction and does not encompass the thermochemical conversion of CO2 with green hydrogen.
PSE contributions to ECO2R can be manifold, starting with the synthesis of the best up- and downstream process configurations to obtain the desired final products, once these are produced in the electrochemical cell. Complex separation systems are expected, for the liquid and gas mixtures leaving the cell will contain both desired products to be isolated and unreacted species to be recycled, chiefly CO2. How these separation and recycle systems will integrate with the modular nature of electrochemical reactors is another aspect in which PSE can contribute significantly. Furthermore, these processes can be optimised using different techniques, while considerations on the dynamics and control of units powered by intermittent energy sources can be drawn. The coupling of chemical processes with energy systems is a growing part of the PSE domain, as can be seen in the case of demand side management studies (Zhang and Grossmann, 2016). Further examples of applications are in the development of heat integration schemes. A more recent paper reviews the recent technological developments and process-level analysis of ECO2R technologies, focusing on early-stage evaluation of electrified conversion processes in terms of sustainability, and the optimal sizing of the electrified systems under the intermittency of renewable electricity (Chung et al., 2023).
Despite significant efforts devoted to the development of ECO2R, there are still many challenges left that hinder further approaches to industrial applications. In this context, this paper gives a perspective on these challenges from a PSE viewpoint, while at the same time highlighting the opportunities for advancements in the field, with a focus on the European arena.
2 Challenges
The technical and system challenges are plentiful. The most pressing ones are detailed as follows.
2.1 Renewable electricity generation
The integration between ECO2R and renewable electricity sources is of particular interest in the realm of electrochemical processes, since electricity consumption is one of the most relevant operating expenses (Shin, Hansen, and Jiao, 2021). In addition to this, ECO2R relies on the use of renewable electricity to allow for CO2 emissions mitigation (Jouny, Luc, and Jiao, 2018), making the two systems inextricably connected. Therefore, even though the challenges discussed hereafter are intrinsic to the power generation system, they translate into associated challenges for the ECO2R field.
• Price of renewable electricity. The economic viability of these technologies depends on the availability of abundant and cheap renewable electric energy (<20-30 $/MWh (Ramdin et al., 2021; Sisler et al., 2021)). Large scale installation for renewable energy production have high investment costs which need to be recovered and, even with power purchase agreements or similar options in place between chemical companies and energy operators, this seems difficult at such low prices. Consequently, if appropriately priced renewable electricity is not likely in the long term, then ECO2R will not be a competitive approach, regardless of technological advancements in cell and process design. Assuming a direct connection of the ECO2R plant to the power grid, this challenge is reflected in the degree of renewables in the grid itself (Ueckerdt et al., 2024).
• Amount of renewable electricity. The scale of the electricity generation is also an issue, as the separation of diluted streams and the electrolysis especially are highly energy intensive processes. Looking at ethylene, one of the most discussed products of ECO2R, electrolysis alone, without downstream processing, would require a power input in the order of several GWs to match the production capacity of a modern day steam cracker (∼1,000 kt/y) (Alerte et al., 2021). About 17% of electricity generated in the Netherlands would be required to produce ethylene in the Netherlands alone (4,000 kt/y) (IEA, 2023). Large investments in energy generation will need to be made and this might consequently lead to increases in the price of the chemicals, already a critical aspect of the economic viability of these processes (Hydrohub Innovation Program, 2022). The decarbonisation potential of the chemical industry in Europe would require more low carbon energy than it is predicted by the International Energy Agency to be overall available (Bazzanella and Ausfelder, 2017).
• Competition with other sectors. Even though renewable power capacity is being added at a growing rate, the impact of these additions on the decarbonisation of electricity generation, except for a few cases, has not been so large, but rather it has contributed to the fulfilment of the globally growing demand (IEA, 2022a). All sectors display strong demands for renewable electrons, as can be seen with the transportation sector which consumes right now less than 2% of the world electricity, but is bound to expand its share with the increasing deployment of electric vehicles (IEA, 2019). The environmental returns per renewable electron are different across sectors, highlighting the crucial need for a prioritization in the allocation of renewable electricity. Connecting ECO2R technologies to a power system that is still largely fossil-based would not be advisable, as it would clearly offset the CO2 emission mitigation potential of these technologies. Even in a country with a large penetration of renewables in the grid, such as Germany, the carbon footprint of the grid remains significant at ∼400 gCO2/kWh (Icha and Lauf, 2023).
• Intermittency. The intermittency of the power supply from renewable sources could be compensated by a grid connection in which a base load is guaranteed by other (possibly fossil-based) power generation. However, this should be carefully considered to avoid offsetting the possible emissions reduction promised by ECO2R. Conversely, if the ECO2R is directly coupled with renewable power generation, a mitigation avenue could be energy storage, for example, by means of batteries (for example, vanadium redox flow batteries, which have been installed for energy storage with capacities up to 200 MW/800 MWh (Pal, 2023)) but this might increase further the cost of already hardly competitive technologies. Other possibilities lay in the development of flexible and agile processes, which are still in their infancy, as are studies devoted to the identification of optimal placement of buffer capacities, either within the plant or with respect to energy storage. The water electrolysis community is currently exploring operation under intermittency (Kojima et al., 2023), while this operation modality in ECO2R is currently limited to studies of Solid Oxide Cells (Tomberg et al., 2023).
• Power electronics installations. Even though normally not strictly within the battery limits for chemical engineering studies, Capital Expenditures (CAPEX) estimations for electrolysis processes should cover also the installation of power electronics equipment, which would significantly impact the investment and thus the economic viability of the processes considered. For instance, the advanced design and total CAPEX estimation for a 1 GW green hydrogen plan reported in Hydrohub Innovation Program, 2022 show that power supply and electronics installation on site would account for around 1/3 of the total direct costs: this share is likely to be lower for a more complex CO2-based plant, given the larger upstream and downstream requirements, but still very significant. Power electronics would also substantially impact the overall footprint of the factory, considering that power lines, transformers and rectifiers will need to be installed within the factory perimeter.
The optimal allocation of the available and forecasted renewable electricity among different sectors to obtain the largest environmental benefits will have to be decided through system-wide studies, in which PSE, and Life Cycle Assessments (LCAs) in particular, will play a major role. The evaluation of the dynamics, control and availability of the process (Luo, Moncada, and Ramirez, 2022) with electricity intermittency (Roh et al., 2022) or fluctuating energy prices will require robust efforts from the PSE community, especially for the processes which will include complex separation trains which cannot exploit the possible modularity of the electrochemical cells. Insights into the integration with renewable power and with electricity markets can be found in (Guerra et al., 2023) and these could be expanded for the European case considering the current European Union (EU) Emission Trading Scheme (ETS) (Bruninx, Moncada, and Ovaere, 2022).
2.2 Feedstock
Ensuring efficient and scalable CO2 capture and treatment processes is a major challenge for process design, considering that some technologies, while mature (for example, CO2 capture with amines), are expensive and energy intensive. Similar challenges are faced when dealing with the other inlet streams, water and electrolyte. The current experimental research is mostly using very pure feed streams of CO2 and water, which might not be available or might require complex separation steps beforehand.
• CO2 source. The source of CO2 brings several challenges, under the process, system and logistic perspectives (d’Amore, Romano, and Bezzo, 2021; d’Amore and Bezzo, 2020). The concentration of CO2 shows an inverse proportionality with the cost of its capture, leading to a preference for more concentrated streams, such as from bioethanol production (Bains, Psarras, and Wilcox, 2017), while on the other hand the presence of impurities can be detrimental to the performance (in terms of stability, durability and selectivity) of the electrolysers, if they are not removed in advance, generating additional costs (Luc et al., 2019; Harmon and Wang, 2022; Van Daele et al., 2024). Some impurities are also more readily reduced than CO2, as is the case of oxygen, thus decreasing the overall energy efficiency of the process. It is also important to consider possible carbon lock-in effects resulting from the pairing of natural gas and coal fired power plants with CO2-based chemicals productions (Seto et al., 2016), on top of representing a short-circuiting from the power generation point of view: electricity is the product of the coal power plant, but a renewable power plant would be used to supply the energy to convert the CO2 produced by the coal power plant. Instead, this should be shut down and the grid should be provided electricity by the renewable generators. Direct Air Capture (DAC) is a developing technology that might go alongside ECO2R (Almajed et al., 2023), but the projected costs are still very high, with significant impacts on the economic viability of the processes (Young et al., 2023). DAC, due to the high dilution of CO2 in the atmosphere (Darton and Yang, 2020) still does not seem a viable option for industrial applications in the foreseeable future, particularly when paired with already energy intensive and costly technologies (Smith et al., 2019).
• Water and electrolyte. Water quality for the electrochemical reaction could be critical, given the stability issues of the cells. For hydrogen production, demineralised water is required, generating additional requirements for the upstream processing. Moreover, shortages in the supply of water are a problem in many areas of the world (United Nations Educational Scientific and Cultural Organization, 2023). Some of the regions in the European continent that have the highest potential for renewables (in particular photovoltaic), such as the Mediterranean countries, will also face increasing pressure on their water supply (European Commission. Joint Research Centre, 2020). The availability of water might thus be limited, with more intense competition for it among different sectors (domestic, agricultural, industrial). Desalination could be used to mitigate this risk and provide fresh water for these technologies in places with water supply issues, but this would further increase the load on the energy inputs and possibly have environmental side effects (Cai et al., 2023). The electrolyte must also be taken into consideration, as it will not only be part of the bill of materials, but might introduce contaminants in the system (Wuttig and Surendranath, 2015).
2.3 Electrolyser
• Conversion. Low conversion might be desirable to keep current density high (>200 mA/cm2, ideally >1 A/cm2) (Moore et al., 2023), but might yield diluted product streams and result in large recycles of CO2 to be separated with energy intensive processes. Lower single-pass conversion (for example, 10%) would also limit the throughput of the reactor, requiring higher numbers of cells and stacks, with increased CAPEX.
• Carbonate crossover and overall atom economy. Carbonate crossover is also a relevant issue in many cell configurations for low temperature electrolysis, with dire consequences for the process: the CO2 at the cathode is not converted into the desired products, but it forms carbonate ions which migrate to the anode, where they are oxidised back to CO2 (Rabinowitz and Kanan, 2020). The usage of bipolar membranes would allow to avoid this problem, but this comes with significant ohmic losses (Xie et al., 2022). On the anode side, oxygen production takes place, unless a coproduction route is followed (Verma, Lu, and Kenis, 2019; Vass, Endrődi, and Janáky, 2021). Oxygen is considered a by-product and is typically vented (as it is in the water electrolysis field), decreasing further the atom economy of the system.
• Electrolyte recycle. The recycling of the electrolyte in alkaline flow cells appears to be an underdeveloped area in ECO2R, despite its impact on the scaling up possibilities of such systems when producing liquid products. Sisler et al., 2021 present a KOH recycling system in the case of production of ethylene, but when products like formic acid, ethanol and acetic acid are obtained, the electrolyte separation and recycle needs further explorations, as there are significant implications for the operating costs (material requirements, energy expenditure in the separations) and for the environmental performance (increased waste).
• Cell stability. The stability of the cell might be the most pressing problem in the field, in general lacking long term experiments (i.e., above 1,000 h) demonstrating good performance for industrially relevant time scales (Krause et al., 2020 show stable Faradaic efficiency (FE) toward CO for 1,500 h, using a silver catalyst). This is particularly true for copper-based catalysts, which display several degradation mechanisms (Popović et al., 2020).
• Materials consumption. As is the case with many other areas within the energy transition, critical materials consumption plays a role in ECO2R as well. Cathodic electro-catalysts are often relatively abundant and well established materials, such as silver and copper, but issues might arise with anodic catalysts for some cells configurations, such as Proton Exchange Membrane/Polymer Electrolyte Membrane (PEM) cells using iridium (even though nickel could be used instead in some cell configurations (Ádám Vass et al., 2022)). The scarcity of such metal might prove a bottleneck in the scaling up of electrochemical technologies, including water electrolysis (Minke et al., 2021; IEA, 2022b).
• Trade-offs between electrolytic cell Key Performance Indicators. Electrochemical cells are evaluated on the basis of several Key Performance Indicators (KPIs), among which trade-offs exist, such as current density vs. voltage or FE vs. conversion. On this basis, striving for the simultaneous maximisation of all these parameters does not appear possible and the performance of the cell should be optimised from a holistic point of view.
• Scale up to industrial systems. Care should be taken when extrapolating the current performance metrics, like FE and current density, at bench scale to industrial scale. Electrochemical cells typically number up, rather than scale up, which reduces the uncertainty of the extrapolation to some extent, but does not allow exploiting economies of scale. ECO2R technologies are currently at Technology Readiness Level 2-4: this is not considered to be a viable option for commercial implementation in the timeframe of 2050 (Bazzanella and Ausfelder, 2017). As an example, (Grim et al., 2023), analyse a realistic timeline for implementation of production of CO (a relatively low hanging fruit compared to other ECO2R products) by 2050, and at the scale of 100 MW, which is significant but not nearly large enough to bring substantial industry-wide changes.
PSE tools could be used to investigate the possibility of integrating the production of oxygen in existing supply chains, considering its use for in the petrochemical industry and in the metal industry (Berenschot and TNO, 2019), thus adding a revenue stream and boosting the atomic efficiency of the production, while similar analyses could be performed for the co-production case, not neglecting the additional complexities that the co-product separations would entail and the costs associated to additional feedstocks. Techno-economic assessments (TEAs) address the problems of stability, material consumptions and the trade-offs between cell KPIs and they could be integrated with Monte Carlo simulations with defined covariances between the different variables to find feasible solutions while taking these trade-offs into account. PSE tools can be fruitfully used to identify the biggest sources of uncertainty in this respect, to be tackled by targeted experiments, and to guide the development of appropriate pilot/demonstration scale units. Given the scale up characteristics of electrolysis processes, potential benefits might reside in smaller, decentralised productions, instead of the large installations that have characterised the chemical industry since its beginnings. This would be a momentous, industry-wide change in the way some chemical commodities are produced and it should not be proposed without analyses of the new supply chains.
2.4 Process
Overall process development has been based on purely theoretical designs, albeit typically based on established technologies. However, given the novelty and specificity of electrochemical technologies (possibly powered by intermittent electricity inputs) for the manufacture of the chemical commodities discussed in the ECO2R field, many questions are still unanswered with respect to the possible designs and operations of the complete plant.
• Low concentration of liquid products. When the cell produces liquid products, which might have a market of interest, such as ethanol, acetic acid, 1-propanol, they are typically obtained as part of very diluted liquid streams (Xia et al., 2019). Recovering these products can be very expensive and energy intensive (thus potentially proving uneconomical), and it might be further complicated by the presence of the electrolyte, as mentioned previously. The discharge of these trace components to waste water treatment plants should be closely monitored.
• USP and DSP energy usage. Large scale productions centred around CO2 electrolysis would require vast amounts of energy for the separations as well, both upstream (for example, CO2 capture and pre-treatments) and downstream (product recovery and purification). Life cycle assessments should consider the emissions connected with the energy used for these processes, which might offset the CO2 fixed in the products.
• USP and DSP capacity. While the balance of plant design for green hydrogen is relatively straightforward, considering the absence of competing reactions and the generation of the desired products in the gaseous phase, this would not be true for ECO2R. While electrolysers number up instead of scaling up and have quick start-up times (at least for some configurations, such as PEM), the opposite is true for separation systems which present economy of scale advantages and benefit from steady operations. The definition of optimal capacities in the system is still an unanswered question.
Some lessons can be learned from the bioprocess community, which has also faced the challenge of diluted streams. The energy required by the separation steps can be reduced through process integration and intensification, but these analyses should also take into consideration the possible dynamic and flexible nature of these productions, which might come to clash with a very integrated plant. Separation duties could be reduced by accepting trace by-products as tolerable components, depending on the application and/or market of the products and their need for further processing.
2.5 Products
Several products have been obtained at laboratory scale via ECO2R (Grim et al., 2023), but the choice for which are the most interesting ones for possible industrial applications is not obvious. If the vision for ECO2R is to help mitigate the greenhouse gas (GHG) emissions from the chemical industry, then the focus should be on the products with the largest market and the highest impact. Therefore a product such as formic acid, which is the centre of many electrolysis papers, would have only a very limited impact given its small market, but there could still be an interest for alternative processes for the production of the chemical itself–in particular in the case of its proposed use as an hydrogen carrier (Kim et al., 2023).
• Chemicals. Several C1-C3 products can be obtained from ECO2R, including CO, acids, alcohols, aldehydes and fully reduced hydrocarbons (Grim et al., 2023). The products that have received the most attention are carbon monoxide, which can be paired with hydrogen to produce syngas; formate/formic acid, which can be produced with high FE (Fan et al., 2020); and C2+ products, chiefly ethylene. Methanol remains challenging to produce with high FE, while ethanol and fully reduced hydrocarbons require vast amounts of energy. Multi-product facilities, while possibly benefitting from multiple revenue streams, might also suffer from diseconomies of scope (Shin, Hansen, and Jiao, 2021).
• Fuels. Utilising products of ECO2R, such as methane, methanol, ethanol or hydrocarbons, as fuels holds the potential of substituting fossil fuels for hard to decarbonise sectors. However, the amount of primary energy required to produce circular e-fuels by these means amply surpasses the energy output of the combustion of the fuels. As with biofuels (Murphy et al., 2022), the energy return on investment (EROI) is of paramount importance, and it is still very low for ECO2R products (see Gray et al., 2022 for a discussion on e-fuels in general).
• By-products. Gas by-products, in particular the almost inevitable hydrogen, but also CO and CH4, could result in fugitive emissions (Frazer-Nash Consultancy, 2022) which must be carefully prevented through proper design and operation of the plant. Such emissions would prove particularly harmful, given the high Global Warming Potential of H2 (Derwent, 2023) and CH4 (United States Environtmental Protection Agency, 2023). PSE could help addressing the issues related to fugitive emissions by reducing the frequency of start-up and shutdowns, thus minimising the venting of products, or directing streams which might contain GHGs to proper treatment (for example, recombining catalyst, if oxygen is present). The oxygen produced at the anode, as mentioned in Section 2.3, provides a possibility of integration with other productions, thus allowing for its valorisation.
ECO2R could be a part of the chemical industry of the future, alongside biomass-based productions, overall electrification and green hydrogen, which could still be coupled with CO2 capture for the production of chemicals following hybrid routes (Palys and Daoutidis, 2022). The selection of the best technology and production route for different products rests on high level considerations that can be addressed through PSE methods and tools, with LCAs covering a crucial role in this context. For example, Detz et al., 2023 calculated that only very restrictive conditions would enable ECO2R production of ethylene to result in lower emissions than the conventional fossil-based route. Overall, PSE allows studying the integration of these productions in the wider context, such as that of e-fuels, hydrogen carriers or methanol for a methanol economy, or how intermediates could be produced via ECO2R and then converted into higher added value products developing new supply chains.
3 Opportunities
ECO2R holds the promise of valorising CO2 by producing bulk chemicals. On one side, the products that can be obtained have large and well established markets which are expanding globally (Meng et al., 2023), on the other these products contribute largely to the emissions of the chemical industry, leaving a space for novel technologies to tackle them. Therefore, even though the challenges are plentiful, the low level of technological development of ECO2R and the potential benefits of its application still make it an interesting technology with several opportunities. The economic viability of these technologies could be strengthened in case of increases in fossil feedstock prices and/or by taxation on CO2 emissions (Wolde-Rufael and Mulat-weldemeskel 2023). This is particularly true for the European context, which is substantially dependent on energy imports (Eurostat, 2024).
3.1 CO2 valorisation and emission mitigation
The most relevant point of interest for these technologies is their potential in mitigating CO2 emissions. PSE allows a holistic, systematic analysis to understand what technologies, products and processes would afford the largest overall benefits in terms of reduction of greenhouse gas emissions, taking into consideration the carbon intensity of the power generation. A further incentive is provided by the possibility of valorising CO2 as the backbone of organic molecules and hydrocarbons, which would not be derived anymore from fossil resources.
3.2 Integration with point source CO2 emitters
Europe has a strong chemical industry (Cefic, 2023) and ambitious sustainability goals pushing towards the reduction of the climatic impact of the industry at large (European Commission, 2023).
• Heavy industry. Part of the European heavy industry, such as cement and steel, which are hard to decarbonise sectors, might benefit from the integration of these technologies. The concentration and amount of impurities of the CO2 must be taken into account to assess the suitability of these sources for ECO2R processes. Europe also has established markets that would be able to absorb the production of chemicals through novel pathways based on process intensification (Kiss and Smith, 2020).
• Future industries. As the European industry moves towards decarbonisation, new processes will emerge which might constitute possible sources of CO2 or which could be coupled in different ways with CCU technologies, including ECO2R. Biomass processing, plastic recycling and biogas production from organic waste, among others, could lead to a suitable feedstock for ECO2R.
Furthermore, the possibility of disengaging the chemical industry from fossil resources should be of great interest in Europe, notoriously lacking in natural resources (except for coal, North Sea oil, and Dutch natural gas, which is also being phased out). Combining widely available water, CO2 and possibly renewable electricity would improve the independence of the European industry in the face of uncertain international markets.
The integration of different industries with ECO2R can be assessed using the tools of PSE, first of all to understand the suitability of the emissions for use in CO2 electroreduction and the costs connected with its capture and treatment. The mutual benefits of this integration can be evaluated through TEAs, taking into account the current EU ETS incentives.
3.3 Add-ins/on-site ECO2R (capture and utilisation)
Given the amount of energy required to produce base chemicals at industrial scale, it might be of interest to investigate possible ways to integrate CO2 electrolysis into established productions (for example, ethylene for ethylene oxide or vinyl acetate productions (Barecka, Ager, and Lapkin, 2021; Ramdin et al., 2021)), instead of aiming for the substitution of conventional productions. Another possibility is to integrate these processes into hybrid production routes, where they could supplement conventional thermochemical processes (Van Bavel et al., 2020): this is of particular interest for carbon monoxide, which can be produced efficiently via ECO2R (Almajed et al., 2023), whereas its classic counterpart (steam reforming of natural gas) leads to large exergy losses (Moulijn et al., 2013).
The process design and optimisation efforts required by these integrations might be rewarded by improvements in the sustainability performance of the processes via the recycling of CO2 that would otherwise be emitted, while at the same time absorbing part of the costs through a reduction of the original feed consumption.
3.4 Process integration and intensification
The reduction of the primary energy demand is of paramount importance for ECO2R. This decrease can occur at both the reaction and at the overall process level, with the following strategies.
• Electrolyser. While tackling the electrolytic cell energy consumption (for example, the minimisation of the overpotentials) is done primarily by experimentalists, PSE can contribute by guiding the optimal design of the electrolysers through multiscale modelling.
• Reaction-separation-recycle systems. Synergies could be found and exploited between USP and DSP units, for example, with respect to CO2 capture and its recycle after the reaction step. PSE can also provide relevant contributions through process and energy integration and intensification, taking into account the interaction between the system components (reaction-separation-recycle, possibly with different operation modes between units) and by means of process dynamics and control (to operate at optimal process conditions). There is also potential for further investigations into electrification of the upstream and downstream processes, for example, by means of electric boilers (Marsidi, 2018), resistive heating (Mallapragada et al., 2023) or heat-pump assisted distillation (Kiss and Smith, 2020).
• Heat integration. A possible aspect of great potential is the use of the large quantities of heat generated by the electrolysers, albeit typically of low quality in low temperature ECO2R (temperature <100 °C (Masel et al., 2021)), for example, upgrading it to higher temperature levels using heat pumps. In the proposed designs this topic has not been addressed extensively, and avoiding large amounts of energy being dissipated to the environment at the cooling towers would likely influence positively the overall performance of the process. Investigations in this direction could also benefit the water electrolysis community, where a suggestion has been made to reuse excess heat for feed water generation (Alfa Laval, 2023).
3.5 Improve dialog among stakeholders
Tighter communication among the different stakeholders would certainly lead to strong benefits for the advancement of the field. PSE researchers would garner significant advantages from the understanding of the experimentalists, while injecting in the field a more application-oriented mindset, possibly leading to a more targeted development of the technologies.
For example, the product streams composition and the single-pass CO2 conversion are often not clearly reported in experimental papers, not allowing for an easy implementation of the findings into broader process designs by PSE researchers. From the other side, the holistic nature of system-wide PSE studies could be used to bridge possible gaps and to facilitate the discussion towards the implementation and further development of the technologies.
4 Conclusion
An evaluation of the biggest technical and system challenges in the field of ECO2R has been provided, identifying at the same time the most relevant opportunities for PSE studies with respect to these novel technologies, as illustrated in Figure 2. Table 1 expands the overview with suggestions for possible approaches and PSE applications to the challenges faced by ECO2R.
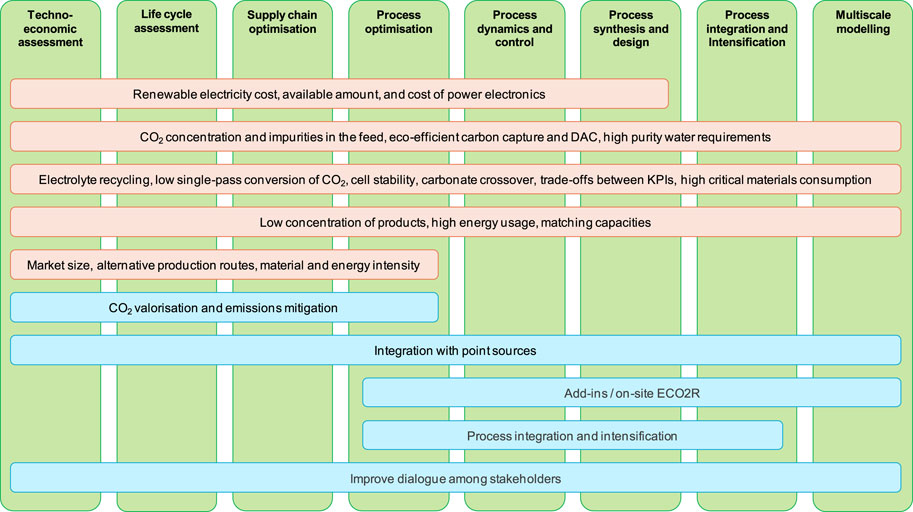
FIGURE 2. Overview of the possible contribution of PSE to ECO2R with challenges in orange and opportunities in blue (derived from (Avraamidou et al., 2020)).
The possible application of PSE tools to ECO2R can be summarised as follows:
• Renewable electricity generation can be integrated into dynamic and demand side management studies to evaluate the best operational modes for the ECO2R plant, considering relevant capacities given the availability of low carbon electricity and possibly factoring in variable pricing regimes.
• Supply chain analysis can be exploited with respect to the CO2 feedstock, exploring the possibilities of integrating ECO2R into established productions.
• The experimental community can find support in the development of the electrolysers from multiscale modelling studies, which would allow to eliminate a priori solutions that would prove unfeasible once scaled up.
• The complex nature of the full reactor-separation-recycle system can be assessed through integrated process design and control, using non-linear analysis.
• The scope of ECO2R in terms of products could be narrowed by taking into account other possible sustainable productions and the scale of renewable energy generation required.
ECO2R can become an industrially relevant technology for the production of chemicals at lower environmental costs than current fossil-based, state-of-the-art industrial productions. However, the deployment at scale of those novel technologies seems to conflict with several issues, hindering their implementation and rollout. The role of ECO2R in the process industry of the future will be determined by a multitude of factors, but its potential to combat the issues of CO2-driven-climate-change must be clarified with systematic and holistic life cycle assessments.
Data availability statement
The raw data supporting the conclusion of this article will be made available by the authors, without undue reservation.
Author contributions
RDM: Writing–review and editing, Writing–original draft, Visualization, Validation, Methodology, Formal Analysis, Conceptualization, Writing–review and editing. AS-T: Writing–review and editing, Validation, Methodology, Formal Analysis. MP-F: Writing–review and editing, Validation, Methodology, Conceptualization. RK: Writing–review and editing, Validation, Methodology, Conceptualization. AK: Writing–review and editing, Writing–original draft, Validation, Supervision, Resources, Project administration, Methodology, Formal Analysis, Conceptualization.
Funding
The author(s) declare that no financial support was received for the research, authorship, and/or publication of this article.
Conflict of interest
The authors declare that the research was conducted in the absence of any commercial or financial relationships that could be construed as a potential conflict of interest.
Publisher’s note
All claims expressed in this article are solely those of the authors and do not necessarily represent those of their affiliated organizations, or those of the publisher, the editors and the reviewers. Any product that may be evaluated in this article, or claim that may be made by its manufacturer, is not guaranteed or endorsed by the publisher.
References
Alerte, T., Edwards, J. P., Gabardo, C. M., O’Brien, C. P., Gaona, A., Joshua, W., et al. (2021). Downstream of the CO2 electrolyzer: assessing the energy intensity of product separation. ACS Energy Lett. 6 (12), 4405–4412. doi:10.1021/acsenergylett.1c02263
Almajed, H. M., Guerra-Fernández, O. J., Smith, W., Hodge, B.-M., and Somoza-Tornos, A. (2023). Evaluating the techno-economic potential of defossilized air-to-syngas pathways. Energy and Environ. Sci. 10, 1039. D3EE02589F. doi:10.1039/D3EE02589F
Amore, F. d’, Romano, M. C., and Bezzo, F. (2021). Carbon capture and storage from energy and industrial emission sources: a europe-wide supply chain optimisation. J. Clean. Prod. 290, 125202. March. doi:10.1016/j.jclepro.2020.125202
Amore, F. d’, and Bezzo, F. (2020). Optimizing the design of supply chains for carbon capture, utilization, and sequestration in Europe: a preliminary assessment. Front. Energy Res. 8, 190. September. doi:10.3389/fenrg.2020.00190
Avraamidou, S., Baratsas, S. G., Tian, Y., and Pistikopoulos, E. N. (2020). Circular economy - a challenge and an opportunity for process systems engineering. Comput. Chem. Eng. 133, 106629. February. doi:10.1016/j.compchemeng.2019.106629
Bains, P., Psarras, P., and Wilcox, J. (2017). CO2 capture from the industry sector. Prog. Energy Combust. Sci. 63, 146–172. November. doi:10.1016/j.pecs.2017.07.001
Barecka, M. H., Ager, J. W., and Lapkin, A. A. (2021). Economically viable CO 2 electroreduction embedded within ethylene oxide manufacturing. Energy and Environ. Sci. 14 (3), 1530–1543. doi:10.1039/D0EE03310C
Bazzanella, A. M., and Ausfelder, F. (2017). Low carbon energy and feedstock for the European chemical industry. Frankfurt am Main: DECHEMA/CEFIC. Available at: https://dechema.de/dechema_media/Downloads/Positionspapiere/Technology_study_Low_carbon_energy_and_feedstock_for_the_European_chemical_industry.pdf.
Berenschot (2019). Oxygen synergy for hydrogen production. Available at: https://projecten.topsectorenergie.nl/storage/app/uploads/public/5e9/47d/800/5e947d8007aed241013086.pdf.
Bruninx, K., Moncada, J. A., and Ovaere, M. (2022). Electrolytic hydrogen has to show its true colors. Joule 6 (11), 2437–2440. doi:10.1016/j.joule.2022.09.007
Cai, Y., Wu, J., Shi, S. Q., Li, J., and Kim, K.-H. (2023). Advances in desalination technology and its environmental and economic assessment. J. Clean. Prod. 397, 136498. April. doi:10.1016/j.jclepro.2023.136498
Cefic (2023). 2023 facts and figures of the European chemical industry. Available at: https://cefic.org/a-pillar-of-the-european-economy/facts-and-figures-of-the-european-chemical-industry/.
Chang, B., Pang, H., Raziq, F., Wang, S., Huang, K.-W., Ye, J., et al. (2023). Electrochemical reduction of carbon dioxide to multicarbon (C 2+) products: challenges and perspectives. Energy and Environ. Sci. 10, 4714–4758. 1039.D3EE00964E. doi:10.1039/D3EE00964E
Chung, W., Jeong, W., Lee, J., Kim, J., Roh, K., and JayLee, H. (2023). Electrification of CO2 conversion into chemicals and fuels: gaps and opportunities in process systems engineering. Comput. Chem. Eng. 170, 108106. February. doi:10.1016/j.compchemeng.2022.108106
Consultancy, F.-N. (2022). Fugitive hydrogen emissions in a future hydrogen economy. Available at: https://assets.publishing.service.gov.uk/media/624ec79cd3bf7f600d4055d1/fugitive-hydrogen-emissions-future-hydrogen-economy.pdf.
Darton, R. C., and Yang, A. (2020). “Removing carbon dioxide from the air to stabilise the climate,” in Advances in carbon management technologies. Editors S. K. Sidkar, and F. Priciotta (Boca Raton: CRC Press), 3–22.
De Luna, P., Hahn, C., Higgins, D., Jaffer, S. A., Jaramillo, T. F., and Sargent, E. H. (2019). What would it take for renewably powered electrosynthesis to displace petrochemical processes? Science 364 (6438), eaav3506. doi:10.1126/science.aav3506
Derwent, R. G. (2023). Global warming potential (GWP) for hydrogen: sensitivities, uncertainties and meta-analysis. Int. J. Hydrogen Energy 48 (22), 8328–8341. doi:10.1016/j.ijhydene.2022.11.219
Detz, R. J., Ferchaud, C. J., Kalkman, A. J., Kemper, J., Sánchez-Martínez, C., Saric, M., et al. (2023). Electrochemical CO 2 conversion technologies: state-of-the-art and future perspectives. Sustain. Energy and Fuels 10, 5445–5472. 1039.D3SE00775H. doi:10.1039/D3SE00775H
European Commission. Joint Research Centre (2020). Climate change and europe’s water resources. LU: Publications Office. doi:10.2760/15553
European Commission (2020). An EU strategy to harness the potential of offshore renewable energy for a climate neutral future. Available at: https://eur-lex.europa.eu/legal-content/EN/TXT/PDF/?uri=CELEX:52020DC0741.
European Commission (2022). REPowerEU: a plan to rapidly reduce dependence on Russian fossil fuels and fast forward the green energy transition. Available at: https://ec.europa.eu/commission/presscorner/detail/en/IP_22_3131.
European Commission (2023). A green deal industrial plan for the net-zero age. Available at: https://commission.europa.eu/system/files/2023-02/COM_2023_62_2_EN_ACT_A%20Green%20Deal%20Industrial%20Plan%20for%20the%20Net-Zero%20Age.pdf.
Fan, L., Xia, C., Zhu, P., Lu, Y., and Wang, H. (2020). Electrochemical CO2 reduction to high-concentration pure formic acid solutions in an all-solid-state reactor. Nat. Commun. 11 (1), 3633. doi:10.1038/s41467-020-17403-1
Farooqi, S. A., Farooqi, A. S., Sajjad, S., Yan, C., and Victor, A. B. (2023). Electrochemical reduction of carbon dioxide into valuable chemicals: a review. Environ. Chem. Lett. 21 (3), 1515–1553. doi:10.1007/s10311-023-01565-7
Gray, N., O’Shea, R., Smyth, B., Lens, P. N. L., and Murphy, J. D. (2022). What is the energy balance of electrofuels produced through power-to-fuel integration with biogas facilities? Renew. Sustain. Energy Rev. 155, 111886. March. doi:10.1016/j.rser.2021.111886
Grim, R. G., Ferrell, J. R., Huang, Z., Tao, L., and Resch, M. G. (2023). The feasibility of direct CO2 conversion technologies on impacting mid-century climate goals. Joule 7 (8), 1684–1699. doi:10.1016/j.joule.2023.07.008
Guerra, O. J., Hussain, M. A., Wilson, A. S., Ana, S.-T., and Hodge, B.-M. S. (2023). Barriers and opportunities for the deployment of CO2 electrolysis in net-zero emissions energy systems. Joule 7 (6), 1111–1133. doi:10.1016/j.joule.2023.05.002
Harmon, N. J., and Wang, H. (2022). Electrochemical CO 2 reduction in the presence of impurities: influences and mitigation strategies. Angew. Chem. Int. Ed. 61 (52), e202213782. doi:10.1002/anie.202213782
Hydrohub Innovation Program (2022). A one-GigaWatt green-hydrogen plant. Available at: https://ispt.eu/media/Public-report-gigawatt-advanced-green-electrolyser-design.pdf.
Icha, P., and Lauf, T. (2023). Entwicklung der spezifischen Treibhausgas-Emissionen des deutschen Strommix in den Jahren 1990 - 2022. Available at: https://www.umweltbundesamt.de/sites/default/files/medien/1410/publikationen/2023_05_23_climate_change_20-2023_strommix_bf.pdf.
IEA (2019). Share of electricity final consumption by sector, 2019. Available at: https://www.iea.org/data-and-statistics/charts/share-of-electricity-final-consumption-by-sector-2019.
IEA (2022a). Renewables 2022. Paris: IEA. Available at: https://iea.blob.core.windows.net/assets/ada7af90-e280-46c4-a577-df2e4fb44254/Renewables2022.pdf.
IEA (2022b). The role of critical minerals in clean energy transitions. Paris: IEA. Available at: https://iea.blob.core.windows.net/assets/ffd2a83b-8c30-4e9d-980a-52b6d9a86fdc/TheRoleofCriticalMineralsinCleanEnergyTransitions.pdf.
IEA (2023). World energy balances highlights. Available at: https://www.iea.org/data-and-statistics/data-product/world-energy-balances-highlights.
Jouny, M., Wesley, L., and Jiao, F. (2018). General techno-economic analysis of CO 2 electrolysis systems. Industrial Eng. Chem. Res. 57 (6), 2165–2177. doi:10.1021/acs.iecr.7b03514
Kim, C., Lee, Y., Lee, H., Lee, U., and Kim, K. (2023). Economic and environmental potential of green hydrogen carriers (GHCs) produced via reduction of amine-captured CO2. Energy Convers. Manag. 291, 117302. September. doi:10.1016/j.enconman.2023.117302
Kiss, A. A., and Smith, R. (2020). Rethinking energy use in distillation processes for a more sustainable chemical industry. Energy 203, 117788. July. doi:10.1016/j.energy.2020.117788
Kojima, H., Nagasawa, K., Todoroki, N., Ito, Y., Matsui, T., and Nakajima, R. (2023). Influence of renewable energy power fluctuations on water electrolysis for green hydrogen production. Int. J. Hydrogen Energy 48 (12), 4572–4593. doi:10.1016/j.ijhydene.2022.11.018
Krause, R., Reinisch, D., Reller, C., Eckert, H., Hartmann, D., Taroata, D., et al. (2020). Industrial application aspects of the electrochemical reduction of CO 2 to CO in aqueous electrolyte. Chem. Ing. Tech. 92 (1–2), 53–61. doi:10.1002/cite.201900092
Laval, A. (2023). Water purification for green hydrogen production. Available at: https://www.alfalaval.com/industries/energy-and-utilities/sustainablesolutions/sustainable-solutions/clean-energy/clean-hydrogen/water-purification-for-green-hydrogen/(Accessed February 29, 2024).
Luc, W., Ko, B. H., Kattel, S., Li, S., Dong, Su, Chen, J. G., et al. (2019). SO 2 -induced selectivity change in CO 2 electroreduction. J. Am. Chem. Soc. 141 (25), 9902–9909. doi:10.1021/jacs.9b03215
Luo, J., Moncada, J., and Ramirez, A. (2022). Development of a conceptual framework for evaluating the flexibility of future chemical processes. Industrial Eng. Chem. Res. 61 (9), 3219–3232. doi:10.1021/acs.iecr.1c03874
Mallapragada, D. S., Dvorkin, Y., Modestino, M. A., Esposito, D. V., Smith, W. A., Hodge, B.-M., et al. (2023). Decarbonization of the chemical industry through electrification: barriers and opportunities. Joule 7 (1), 23–41. doi:10.1016/j.joule.2022.12.008
Marsidi, M. (2018). Technology factsheet. Electric industrial boiler. Available at: https://energy.nl/wp-content/uploads/electric-industrial-boiler-7.pdf.
Masel, R. I., Liu, Z., Yang, H., Kaczur, J. J., Carrillo, D., Ren, S., et al. (2021). An industrial perspective on catalysts for low-temperature CO2 electrolysis. Nat. Nanotechnol. 16 (2), 118–128. doi:10.1038/s41565-020-00823-x
Meng, F., Wagner, A., Kremer, A. B., Kanazawa, D., Leung, J. J., Goult, P., et al. (2023). Planet-compatible pathways for transitioning the chemical industry. Proc. Natl. Acad. Sci. 120 (8), e2218294120. doi:10.1073/pnas.2218294120
Minke, C., Suermann, M., Bensmann, B., and Hanke-Rauschenbach, R. (2021). Is iridium demand a potential bottleneck in the realization of large-scale PEM water electrolysis? Int. J. Hydrogen Energy 46 (46), 23581–23590. doi:10.1016/j.ijhydene.2021.04.174
Moore, T., Oyarzun, D. I., Li, W., Lin, T. Y., Goldman, M., Wong, A. A., et al. (2023). Electrolyzer energy dominates separation costs in state-of-the-art CO2 electrolyzers: implications for single-pass CO2 utilization. Joule 7 (4), 782–796. doi:10.1016/j.joule.2023.03.015
Moulijn, J. A., Makkee, M., Annelies, van D., and Annelies, E. van D. (2013). Chemical process technology. 2. Chichester: Wiley.
Murphy, D. J., Raugei, M., Carbajales-Dale, M., and Rubio Estrada, B. (2022). Energy return on investment of major energy carriers: review and harmonization. Sustainability 14 (12), 7098. doi:10.3390/su14127098
Orella, M. J., Brown, S. M., Yuriy Román-Leshkov, M. L. E. L., Brushett, F. R., and Brushett, F. R. (2020). A general technoeconomic model for evaluating emerging electrolytic processes. Energy Technol. 8 (11), 1900994. doi:10.1002/ente.201900994
Pal, D. P. (2023). Recent technologies for waste to clean energy and its utilization. Singapore: Springer.
Palys, M. J., and Daoutidis, P. (2022). Power-to-X: a review and perspective. Comput. Chem. Eng. 165, 107948. September. doi:10.1016/j.compchemeng.2022.107948
Popović, S., Smiljanić, M., Jovanovič, P., Jan, V., Buonsanti, R., and Hodnik, N. (2020). Stability and degradation mechanisms of copper-based catalysts for electrochemical CO 2 reduction. Angew. Chem. Int. Ed. 59 (35), 14736–14746. doi:10.1002/anie.202000617
Rabinowitz, J. A., and Kanan, M. W. (2020). The future of low-temperature carbon dioxide electrolysis depends on solving one basic problem. Nat. Commun. 11 (1), 5231. doi:10.1038/s41467-020-19135-8
Ramdin, M., Bert De MotMorrison, A. R. T., Breugelmans, T., Leo, J. P. V. D. B., Martin Trusler, J. P., Kortlever, R., et al. (2021). Electroreduction of CO2/CO to C2 products: process modeling, downstream separation, system integration, and economic analysis. Industrial Eng. Chem. Res. 60 (49), 17862–17880. doi:10.1021/acs.iecr.1c03592
Roh, K., Brée, L. C., Schäfer, P., Strohmeier, D., and Mitsos, A. (2022). Flexible operation of modular electrochemical CO2 reduction processes. IFAC-PapersOnLine 55, 298–303. doi:10.1016/j.ifacol.2022.07.460
Seto, K. C., Davis, S. J., Mitchell, R. B., Stokes, E. C., Unruh, G., and Ürge-Vorsatz, D. (2016). Carbon lock-in: types, causes, and policy implications. Annu. Rev. Environ. Resour. 41 (1), 425–452. doi:10.1146/annurev-environ-110615-085934
Shin, H., Hansen, K. U., and Jiao, F. (2021). Techno-economic assessment of low-temperature carbon dioxide electrolysis. Nat. Sustain. 4 (10), 911–919. doi:10.1038/s41893-021-00739-x
Sisler, J., Khan, S., Ip, A. H., Schreiber, M. W., Jaffer, S. A., Bobicki, E. R., et al. (2021). Ethylene electrosynthesis: a comparative techno-economic analysis of alkaline vs membrane electrode assembly vs CO 2 –CO–C 2 H 4 tandems. ACS Energy Lett. 6 (3), 997–1002. doi:10.1021/acsenergylett.0c02633
Smith, W. A., Thomas Burdyny, D. A. V., Geerlings, H., and Geerlings, H. (2019). Pathways to industrial-scale fuel out of thin air from CO2 electrolysis. Joule 3 (8), 1822–1834. doi:10.1016/j.joule.2019.07.009
Somoza-Tornos, A., Guerra, O. J., Crow, A. M., Smith, W. A., and Hodge, B.-M. (2021). Process modeling, techno-economic assessment, and life cycle assessment of the electrochemical reduction of CO2: a review. iScience 24 (7), 102813. doi:10.1016/j.isci.2021.102813
Tomberg, M., Heddrich, M. P., Ansar, S. A., and Andreas Friedrich, K. (2023). Operation strategies for a flexible megawatt scale electrolysis system for synthesis gas and hydrogen production with direct air capture of carbon dioxide. Sustain. Energy and Fuels 7 (2), 471–484. doi:10.1039/D2SE01473D
Ueckerdt, F., Verpoort, P. C., Anantharaman, R., Bauer, C., Beck, F., Longden, T., et al. (2024). On the cost competitiveness of blue and green hydrogen. Joule 8 (1), 104–128. doi:10.1016/j.joule.2023.12.004
United Nations Educational Scientific And Cultural Organization (2023). United nations world water development report 2023: partnerships and Cooperation for Water. S.l.: united Nations. Available at: https://unesdoc.unesco.org/ark:/48223/pf0000384655.
United States Environmental Protection Agency (2023). Understanding global warming potentials. Available at: https://www.epa.gov/ghgemissions/understanding-global-warming-potentials#:∼:text=Methane%20(CH4)%20is%20estimated,less%20time%20than%20CO2.
Van Bavel, S., Verma, S., Negro, E., and Bracht, M. (2020). Integrating CO2 Electrolysis into the Gas-to-Liquids–Power-to-Liquids Process. ACS Energy Lett. 5 (8), 2597–2601. doi:10.1021/acsenergylett.0c01418
Van Daele, S., Hintjens, L., Hoekx, S., Bohlen, B., Sander, N., Daems, N., et al. (2024). How flue gas impurities affect the electrochemical reduction of CO2 to CO and formate. Appl. Catal. B Environ. 341, 123345. February. doi:10.1016/j.apcatb.2023.123345
Vass, Á., Endrődi, B., and Janáky, C. (2021). Coupling electrochemical carbon dioxide conversion with value-added anode processes: an emerging paradigm. Curr. Opin. Electrochem. 25, 100621. February. doi:10.1016/j.coelec.2020.08.003
Vass, Á., Kormányos, A., Kószó, Z., Endrődi, B., and Janáky, C. (2022). Anode catalysts in CO 2 electrolysis: challenges and untapped opportunities. ACS Catal. 12 (2), 1037–1051. doi:10.1021/acscatal.1c04978
Verma, S., Lu, S., and Kenis, P. J. A. (2019). Co-electrolysis of CO2 and glycerol as a pathway to carbon chemicals with improved technoeconomics due to low electricity consumption. Nat. Energy 4 (6), 466–474. doi:10.1038/s41560-019-0374-6
Wolde-Rufael, Y., and Mulat-weldemeskel, E. (2023). Effectiveness of environmental taxes and environmental stringent policies on CO2 emissions: the European experience. Environ. Dev. Sustain. 25 (6), 5211–5239. doi:10.1007/s10668-022-02262-1
Wuttig, A., and Surendranath, Y. (2015). Impurity ion complexation enhances carbon dioxide reduction catalysis. ACS Catal. 5 (7), 4479–4484. doi:10.1021/acscatal.5b00808
Xia, C., Zhu, P., Jiang, Q., Pan, Y., Liang, W., Stavitski, E., et al. (2019). Continuous production of pure liquid fuel solutions via electrocatalytic CO2 reduction using solid-electrolyte devices. Nat. Energy 4 (9), 776–785. doi:10.1038/s41560-019-0451-x
Xie, Ke, Miao, R. K., Ozden, A., Liu, S., Chen, Z., Dinh, C.-T., et al. (2022). Bipolar membrane electrolyzers enable high single-pass CO2 electroreduction to multicarbon products. Nat. Commun. 13 (1), 3609. doi:10.1038/s41467-022-31295-3
Young, J., McQueen, N., Charalambous, C., Foteinis, S., Hawrot, O., Ojeda, M., et al. (2023). The cost of direct air capture and storage can Be reduced via strategic deployment but is unlikely to fall below stated cost targets. One Earth 6 (7), 899–917. doi:10.1016/j.oneear.2023.06.004
Keywords: CO2 electroreduction, CO2 utilisation, green processing, electrification, process systems engineering
Citation: Dal Mas R, Somoza-Tornos A, Pérez-Fortes M, Kortlever R and Kiss AA (2024) Challenges and opportunities for CO2 electroreduction from a process systems engineering perspective. Front. Energy Res. 12:1340622. doi: 10.3389/fenrg.2024.1340622
Received: 18 November 2023; Accepted: 06 March 2024;
Published: 19 March 2024.
Edited by:
Rajagopalan Srinivasan, Indian Institute of Technology Madras, IndiaReviewed by:
Niket Kaisare, Indian Institute of Technology Madras, IndiaCopyright © 2024 Dal Mas, Somoza-Tornos, Pérez-Fortes, Kortlever and Kiss. This is an open-access article distributed under the terms of the Creative Commons Attribution License (CC BY). The use, distribution or reproduction in other forums is permitted, provided the original author(s) and the copyright owner(s) are credited and that the original publication in this journal is cited, in accordance with accepted academic practice. No use, distribution or reproduction is permitted which does not comply with these terms.
*Correspondence: Anton A. Kiss, YS5hLmtpc3NAdHVkZWxmdC5ubA==