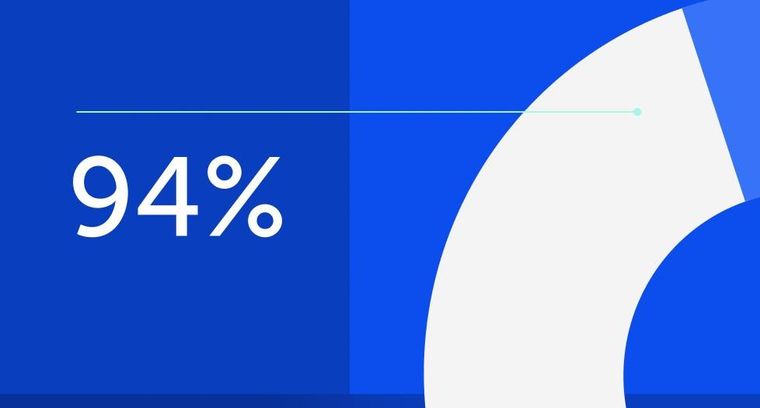
94% of researchers rate our articles as excellent or good
Learn more about the work of our research integrity team to safeguard the quality of each article we publish.
Find out more
REVIEW article
Front. Energy Res., 23 January 2024
Sec. Process and Energy Systems Engineering
Volume 11 - 2023 | https://doi.org/10.3389/fenrg.2023.1344216
This article is part of the Research TopicAdvancements and Future Directions in Process Design for Sustainable Energy SystemsView all 5 articles
Amid escalating energy demands and growing environmental concerns, educational institutions are transforming into crucibles for enduring innovation. This comprehensive review summarizes the complex relationship between Energy Efficiency and Renewable Energy Systems (EERES) within the sphere of educational institutions. By instituting Energy Efficiency initiatives, organizations can curtail energy consumption, resulting in substantial cost savings and a diminished carbon footprint. Moreover, the integration of renewable energy technologies empowers the localized generation of electricity, ensuring a reliable and sustainable energy source. Noteworthy in this study is the novel linkage of energy usage indicators to the comfort levels experienced in university settings during both summer and winter. An exhaustive examination of consumption indicators, rooted in the diverse activities on college campuses, further enriches the investigation. Beyond technical intricacies, this study scrutinizes the economic viability, environmental advantages, and educational significance of these integrated systems. Embracing EERES not only aligns with the commitment of educational institutions to environmental stewardship but also establishes a paradigm for broader communities. These institutions aspire to exemplify sustainable practices, influencing larger societal behavior positively. Various factors, including the institution’s type, energy source, facility nature, building construction, internal activities, weather conditions, and user behavior, wield substantial influence over energy utilization. This study offers a nuanced exploration, shedding light not only on the technical dimensions but also on the broader economic, environmental, and educational implications of adopting EERES.
In the modern period, as the world grapples with the dual issues of rising energy consumption and climate change, educational institutions serve as forerunners of sustainable progress. The incorporation of Energy Efficiency and Renewable Energy Systems (EERES) in educational facilities constitutes a watershed moment in the transition to a greener, more energy-conscious future. By integrating EERES technology, educational institutions can not only make a substantial contribution to the worldwide fight against climate change but also function as instructional hubs, imparting essential lessons on sustainability. This research lays the groundwork for further investigation of the symbiotic link between EERES in educational environments. By implementing energy-efficient procedures, institutions can reduce their overall energy usage, resulting in significant financial savings and a lower carbon footprint. Concurrently, the incorporation of solar energy systems provides the opportunity to generate clean electricity on-site, offering a reliable and sustainable energy supply. This study will also untangle the web of energy-saving techniques, solar technology, and their seamless incorporation into the fabric of educational infrastructure.
Implementing smart lighting systems that respond to natural light patterns and use renewable energy (RE) to illuminate educational buildings around the world benefits not only the economic, environmental, and educational aspects of embracing EERES but also closely aligns with achieving the United Nations’ sustainable development goals. These technologies dramatically cut operating costs, reduce carbon emissions, and promote resource conservation, all of which contribute directly to goals such as affordable and clean energy (Goal 7), sustainable cities and communities (Goal 11), and climate action (Goal 13). This investigation will inspire educational institutions to embrace the transformative power of EERES, instilling a sense of environmental responsibility in students and teachers and cultivating a future generation of global citizens committed to the broader UN sustainability agenda, promoting both academic excellence and a sustainable future for future generations. University campus infrastructure is being built swiftly as a result of the increased demand for power caused by rising student enrollment and the growth of research facilities. As a result, environmental and sustainability considerations are given top importance in the development and operation of institutions. Universities accounted for 19.7% of the government sector’s global electrical energy consumption in 2021 (Saudi Electric Company, 2021). The whole energy balance of buildings, including the university campus, is depicted in Figure 1 There are frequently open, spacious areas on campus that might be used with RE resources. On the vertical axis, three uses of the sanctuary are depicted:
1. Without reducing energy consumption and using renewable energy RE sources, the total load in the first scenario,
2. The load after considering options for reducing energy consumption and improving efficiency, such as increasing the energy efficiency (EE) of obsolete equipment and lighting systems and improving the thermal insulation and airtightness of the structure.
3. The load after reducing energy consumption by adopting energy-saving strategies, improving EE, and gaining access to RE sources. In this circumstance, there are three phases: keeping a fraction of the campus load covered by fossil fuels, constructing a virtually zero-energy campus, and ultimately, creating a nearly zero-bill campus (Alfaoyzan and Almasri, 2023a).
Most sources claim that energy-saving strategies do not necessitate additional spending, although increasing EE does necessitate installation costs, which are often economically justifiable. This also improves the environment and boosts thermal comfort due to the thermal insulation of the building envelope. Enhancing efficiency and optimizing energy consumption are well-known approaches. The cost of establishing it and the cost of a conventional energy unit are the two most critical parameters that impact its economic feasibility (Sait, 2013; Mytafides et al., 2017; Emil and Diab, 2021; Hamida et al., 2021; Alfaoyzan and Almasri, 2023b). There is a strong consensus among experts and policymakers that energy efficiency and renewable energies are essential components of a sustainable energy future. While EE focuses on using energy more wisely, renewable energies offer a clean and inexhaustible source of power. The integration of both approaches is crucial for achieving a resilient, low-carbon, and sustainable energy system on a global scale. It should be mentioned here that EE is often less expensive than implementing RE (Esmaeil et al., 2019).
On the other hand, the integration of EE and RE, and into study plans can offer numerous benefits to students pursuing various academic disciplines. The integration of energy management systems, renewable energy, and energy efficiency into study plans not only enhances the academic experience but also equips students with the knowledge and skills needed to address global challenges, contribute to sustainable development, and thrive in a rapidly evolving energy landscape. It aligns education with the principles of environmental stewardship, economic viability, and social responsibility, preparing students to be leaders in the pursuit of a more sustainable and resilient future.
Several countries have conducted studies on energy use and EE in buildings, which will be discussed in this paragraph. However, research has paid little attention to the energy situation of academic buildings (Mohd Shukri et al., 2022). Investigated the energy usage of Universiti Tun Hussein Onn Malaysia in order to develop an EE standard for higher education facilities. According to research, the typical achievable yearly energy use range was 72.5–141.0 kWh/m2. Buildings with an annual energy use intensity (EUI) of 72.5 kWh/m2 are considered the most energy-efficient, while those with intensities greater than 141.0 kWh/m2 are considered inefficient (Liu and Ren, 2020). Examined energy use in accommodations and libraries to investigate the relationship between system design and EE in order to determine whether the 65% energy-saving target was met (Gaspar et al., 2022). Investigated the impact of the COVID-19 lockout on energy consumption in the Universitat Politècnica de Catalunya-Barcelona buildings in Spain. Based on how the buildings were used, they discovered that energy consumption was reduced by 57.1%–74.2% from before the lockdown. King Saud University conducted a field survey on electricity consumption. The institution spends almost four million riyals per year on power, with “air conditioning,” “lighting,” and “computer devices” topping the list (MSPI, 2022; Davis and Nutter, 2010). Classified the occupancy profiles as administrative, library, recreational, service, research, and classroom facilities. This classification was used to compare the EUI of university buildings in this study, with the exception of the table that analyzed energy usage across the university campus. In 2017, Ntsaluba and Mukadi presented an analysis of the energy consumption of the University of Johannesburg campus buildings (Ntsaluba and Mukadi, 2019). There are three types of structures on campus: student homes, student centers, and main campus buildings (which include labs, libraries, offices, and classrooms). According to the findings, student centers utilized 3% of the energy, dorms 33%, and main campus structures 64%. The findings revealed a significant relationship between energy use and ambient temperature in primary campus buildings and dorms, but only a minor relationship between energy use and temperature in student centers (Chen et al., 2021). Created a system that uses artificial neural network and fuzzy logic approaches to estimate the real-time occupancy rate and power usage of the University of Glasgow campus based on a variety of meteorological parameters. According to the authors’ findings, the fitted power demand closely approaches the observed real power usage.
It has been noted that there are multiple studies on energy consumption in general and fewer on opportunities for energy conservation. Many institutions have focused on energy efficiency measures, such as retrofitting buildings with energy-efficient technologies and optimizing heating, ventilation, and air conditioning (HVAC) systems. However, the impact of individuals’ behavior on consumption is still unclear, which makes it a necessity for study. Future research endeavors could address the gaps by establishing standardized metrics for evaluating energy performance in higher education settings. Comparative analyses should extend beyond regional considerations to encompass diverse institutional profiles, taking into account variations in size, academic focus, and financial resources. Longitudinal studies tracking the evolution of sustainability initiatives over extended periods would provide insights into the durability and scalability of implemented strategies. Furthermore, investigating the socio-economic factors influencing the adoption of sustainable practices in different institutional contexts could contribute to a more comprehensive understanding of the challenges and opportunities in the higher education energy landscape.
Numerous studies on the energy consumption and effectiveness of university buildings have been conducted in Greece (Stavropoulos et al., 2016; Droutsa et al., 2017; Mytafides et al., 2017). Mytafides and others investigated energy-saving solutions at a university in northeastern Greece (Mytafides et al., 2017). According to the study’s findings, while the new measures reduced the annual EUI to 41 kWh/m2 and the amount of air pollution to 9%–109%, the baseline annual EUI was less than 167 kWh/m2 (Stavropoulos et al., 2016). Employed smart grids to create an integrated platform for energy supply and monitoring for the buildings of the International Hellenic University. They stated that the device reduced daily usage in the average university workplace by 16%. The study (Droutsa et al., 2017) on energy use in non-residential buildings by Droutsa et al. looked at how much energy non-residential buildings in Greece utilized. The annual EUI for the university was reported to be 321.6 kWh/m2.
According to the EUI indicators, American schools use more energy than other countries’ colleges, but Japanese universities use less energy ((Ma et al., 2015)). According to the authors, university energy usage indexes vary greatly. For example, whereas total annual energy consumption ranged between 250 and 800 kWh/m2, annual electrical EUI values ranged from 25 to 427 kWh/m2 (Li and Chen, 2021). Evaluated the relationship between EUI values and floor-area percentages of buildings on a university main campus in Calgary, Canada. The writers classified university construction activities as laboratories, school services, public services, and others. The lab areas had a yearly average EUI of 800 kWh/m2, while school services (classrooms, offices, and healthcare rooms) and public services (food service and facilities, washroom, restroom, library, and so on) contributed 405 and 338 kWh/m2, respectively. Following a comparison of public and educational facilities, the authors hypothesized that limiting public access and regulating occupancy could help campus buildings consume less energy. Jradi et al. (2017) investigated the OU44 Building, an extremely energy-efficient teaching facility at the University of Southern Denmark in Odense, Denmark. According to the study, there is a 55% disparity in energy use between Danish BE10 software and actual data. Using EnergyPlus software, this difference can be reduced to roughly 11%. Guan et al. (2016) used descriptive statistics to evaluate energy utilization on a Norwegian university campus. According to the authors, the campus used an average of 60,070 MWh of electricity and 30,343 MWh of heat energy every year between 2008 and 2013. As a result, the yearly EUI for heating and electricity in the building area ranged from 85 to 121 kWh/m2 and 182 to 206 kWh/m2, respectively. The total yearly electricity use increased somewhat over time, possibly due to rising demand.
Sait (2013) investigated the amount of power utilized in a school in Rabigh City, Kingdom of Saudi Arabia (KSA). HVAC account for up to 91% of all electric energy used, according to the author, and efficiency may increase by 31%. It was discovered that the thermostat was set lower than the recommended 23°C, squandering electricity. A two-degree temperature difference, up to 23°C instead of 21°C, was discovered to save up to 37% of total energy consumed. According to an analysis of the recommended changes, energy savings of up to 45% are possible. (Sait, 2013), reported that in KSA the annual electricity consumption of 2,400 kWh per person, while 12,987–16,351 kWh by Alfaoyzan and Almasri (2023b) calculated a range of 12,987 to 16,351 kWh. In a similar vein, Al-Tamimi claimed that the Kingdom of Saudi Arabia’s per capita power usage was 9,137 kWh (Al-Tamimi, 2017). When all of the energy conservation measures (ECMs) advised by Hamida et al. (2021) were implemented, the EUI decreased from 217.1 to 167.5 kWh/m2. According to the authors’ findings, air conditioning (AC) used more than 73% of the electricity (Chung and Rhee, 2014). Assessed the current buildings at Soongsil University in South Korea to evaluate how much energy was being used. They suggested that usage might be cut by 6%–29% (Bayoumi, 2018). Investigated various techniques of incorporating solar energy at King Abdulaziz University (KAU)’s main campus in Jeddah, Saudi Arabia. According to the author, the academic region has an average yearly EUI of 956 kWh/m2, which accounts for 64% and 65% of university cooling and electric energy demand, respectively (Emil and Diab, 2021). Used EnergyPlus to evaluate ECMs in a building at Egypt’s Ain Shams University. The authors stated that changes to the HVAC system and a 36% reduction in the building’s energy use resulted in more than 50% energy savings. According to the findings, the yearly EUI of the building might be reduced from 230 to 106 kWh/m2.
Chang et al. (2019) discussed the relationships between urban geometric shapes and performance requirements based on a multivariate study. According to the study, a building coverage ratio greater than 0.17 is advised to preserve optimal solar potential. A sky view factor of 54.17% is recommended to maintain optimal energy balance. These connections help to build sustainability design ideas for campuses. The EnergyPlus simulation was used to undertake an energy study of the annual EUI and prospective solar power output on the Georgia Institute of Technology campus in Shenzhen, China.
Rey-Hernández et al. (2023) discussed the usage of a biomass-fueled District Heating System (DHS) at Spain’s University of Valladolid as part of an energy rehabilitation project to build campuses with minimal carbon dioxide emissions. According to the authors, the DHS has reduced overall primary energy use by 23.17% when compared to earlier systems. The usage of renewable primary energy has increased by 94.7%, increasing yearly consumption by 14,907 MWh. Before the refurbishment, the various building components required 60–430 kWh/m2 of fossil fuel every year for these reasons. This method allows for a yearly average use of 100–120 kWh/m2 (Bourdeau et al., 2018). Investigated the total energy (gas and electricity) consumption on three campuses in the Paris region of France. They discovered that the annual average EUI ranged between 52 and 517 kWh/m2. According to Bayoumi (2018), the EUI value with the highest energy use is electrical energy. This could be caused by the region’s harsh temperature, human behavior, or insufficient thermal insulation. The average yearly EUI, cooling-degree days (CDD), and heating-degree days (HDD) are shown in Table 1. It also demonstrates that the EUI differs amongst campuses. The HDD and CDD were calculated using (Worldwide, 2022) based on a 5-year average (2018–2022) and a base temperature of 20°C and 24°C, respectively. Usually, one temperature is used for HDD and CDD. In this work, two values were set, one for cooling and the other for heating. Based on these values, the trend of energy consumption for cooling or heating can be known. When the CDD values are high, it indicates high electricity consumption for cooling and vice versa. Most international codes used internal comfort temperatures as a reference temperature based on the seasons. Although the EUI values ranged from 40 to 956 kWh/m2, the bulk of the situations in the table show an EUI of 150–250 kWh/m2. These figures, according to YILDIZ and KOCYIGIT (2021) varied from 68 to 506 kWh/m2. According to the authors, the indication used both thermal and electrical energy (Birch et al., 2019). Used data from the building energy management system to measure uncontrolled power use in United Kingdom (United Kingdom) universities, which included elevators, server rooms, and tiny power loads. According to the authors, the yearly electrical usage was found to be generally high, ranging from 92 to 573 kWh/m2. Unregulated electricity consumption ranged from 85 to 97 kWh/m2 in the specific laboratory case study (Li et al., 2020). Proposed a method for analyzing the power usage of the university building portfolio at the University of Wollongong’s main campus in Australia. The profiles of daily and annual electricity consumption were developed. After calculating the estimated building EUI, the ratio of measured to expected EUI was calculated.
Using machine learning techniques (Quevedo et al., 2023), set a benchmark for energy use in Brazilian institutions. The actual yearly EUI at Federal University of Santa Catarina was 98.6 kWh/m2, compared to the Support Vector Machine model’s prediction of 98.3 kWh/m2. Annual EUIs ranged from 98.3 to 159.6 kWh/m2 for the models in use. In addition, the authors demonstrated that an efficient university campus requires an annual EUI of less than 90.71 kWh/m2 (Mohamed et al., 2022). Developed energy analysis techniques for Jordan’s Princess Sumaya University of Technology. A Solar System meets the majority of the building’s electrical needs (78% of total power used). According to the authors, implementing the two suggested ECMs will reduce the EUI from 352 to 211 kWh/m2 (Jami et al., 2021). Investigated the energy behavior of students living in dorms on the campus of Shahid Beheshti University in Tehran, Iran. According to the scientists, utilizing ECMs could result in an increase in energy efficiency of 32%–60%.
These detailed figures offer a nuanced understanding of the energy performance of individual campuses. Synthesizing findings from different studies reveals common trends and variations. Across multiple studies, it is evident that energy efficiency measures, such as retrofitting buildings and optimizing lighting systems, consistently contribute to lower EUI values. Despite the insightful quantitative data provided by these studies, it is important to note the variations in reporting metrics and methodologies, which may influence the comparability of results. Standardizing measurement and reporting practices could enhance cross-study analysis and facilitate a more comprehensive understanding of the impact of sustainability initiatives on campus energy use. As the research landscape evolves, future studies should aim for a harmonized approach to data collection and reporting, allowing for more robust comparisons and generalizable findings.
The current section is about EUI in the classroom. According to Bayoumi (2018), the average yearly EUI for classrooms was between 525 and 1,500 kWh/m2 (Bonnet et al., 2002). Calculated the annual electricity consumption for each activity on the campus of the University of Bordeaux in France. The authors calculated the annual electricity consumption for each activity on the University of Bordeaux’s French campus. According to them, the building surface area to electricity usage ratios ranged from 1 to 10. This criterion, however, did not produce comparable results, particularly in terms of building quality, energy systems, consumption, and size (Khoshbakht et al., 2018). Investigated energy use by building type to create an energy benchmark system for Griffith University as a case study in Australia. According to the authors, annual EUI values in academic buildings ranged from 121 to 379 kWh/m2 depending on activity. According to their findings, research buildings had the highest benchmark value of 216 kWh/m2, while academic office buildings had the lowest benchmark value of 137 kWh/m2. In terms of fields, the benchmark EUI value for research was 164 kWh/m2, whereas the value for health was 136 kWh/m2. According to their findings, academic office buildings had the lowest annual EUI value, measuring 137 kWh/m2, while research buildings had the greatest value, measuring 216 kWh/m2 (Rey-Hernández et al., 2023). Discovered that the annual average EUI for classrooms at the University of Valladolid was between 65 and 255 kWh/m2, whilst (Chihib et al., 2020) discovered that it was between 41 and 120 kWh/m2 for various activities at the University of Almeria in Spain.
Table 2 shows a comparison of the EUI of classrooms under various settings. While (Khoshbakht et al., 2018; Gui et al., 2021; Amber et al., 2017; Litardo et al., 2021) provided medium values (Bonnet et al., 2002; Chihib et al., 2020; Sun et al., 2021), provided low values (Bayoumi, 2018; Rey-Hernández et al., 2023) provided extremely high values, especially Bayoumi (2018). User behavior plays a significant role in influencing energy consumption patterns. The way individuals and organizations use energy can have both direct and indirect impacts on overall energy consumption. It is important to note that occupants and space usage have a significant impact on energy consumption when it comes to university typology and benchmarking. As a result, according to Quevedo et al. (2024) report, the spaces should be considered in university energy benchmarks. Synthesizing findings across studies underscores the influence of several factors on classroom EUI values. Building characteristics, such as age, insulation, and architectural design, play a crucial role. Older buildings may have lower energy efficiency and higher EUI values compared to modern, well-designed structures. Local climate conditions, with extremes in temperature, can impact HVAC requirements, affecting EUI values. Lastly, user behavior, including patterns of occupancy, lighting preferences, and technology use, introduces another layer of variability.
TABLE 2. Average annual EUI for classrooms, and cooling- and heating-degree days for different universities globally.
While these studies provide valuable insights into classroom EUI, it is essential to consider the complexity of the factors contributing to variations in energy consumption. Standardizing reporting methodologies and accounting for these influencing factors will be crucial for more accurate cross-institutional comparisons and the development of targeted energy efficiency strategies. As research in this field progresses, a more nuanced understanding of the interplay between these factors can inform tailored interventions to enhance energy efficiency in classrooms across diverse higher education settings. The type of university, and the energy supply, are also a few examples of influences.
This section goes over the annual EUI by administrative region. According to Bayoumi (2018), the average yearly EUI for administrative use was between 700 and 1,000 kWh/m2 (Ligade and Razban, 2019). Used the eQuest energy program to conduct an energy-efficient retrofit assessment for the Indiana University office building in the United States. The building’s electric EUI was 421, while the standard guideline in the US is 140 kWh/m2. The model was then subjected to two ECMs. In the second ECM, a single-duct variable air volume system supplied chilled water and electric reheat, while a dual-fan dual-duct system supplied steam heating and chilled water. The single duct variable air volume system with chilled water and electric reheat was determined to be the most energy-efficient system, saving 28% on utility expenses. It was also fascinating to note that the initial ECM cut annual utility expenses by 18% despite not affecting energy savings. The Paricarana Campus buildings of the Brazilian Federal University of Roraima were the focus of Almeida et al. (2021) energy use analysis. Although teaching rooms account for the bulk of campus rooms (22%), their yearly energy use varies greatly, ranging from 132 to 297 kWh/m2. According to the findings of the inquiry, offices and classrooms consumed 48% of total energy. Air conditioning accounted for 63% of utilization, lighting for 18%, personal use for 15%, and other uses for 4%, according to the authors. Table 3 compares the EUI of the administrative area for various instances. The type of university, the energy supply, the structure, the climate, and user behavior are all potential factors.
TABLE 3. Average annual EUI for administrative use, and cooling- and heating-degree days for different universities globally.
The average annual EUI in labs is the subject of the current sentence. The typical yearly EUI for labs, according to Bayoumi (2018) in KSA, varied between 500 and 1,400 kWh/m2, and according to Rey-Hernández et al. (2023) in Spain, ranged from 35 to 58 kWh/m2. The main reason for this discrepancy may be the climate, the nature of the labs at the university, the behavior of users, and the price of energy. It is also noted from Table 4 that EUI in Malaysia is low, while in Australia it is high may be due to the harsh climate.
TABLE 4. Average annual EUI for lab, and cooling- and heating-degree days for different universities globally.
The average annual EUI of the library is discussed in the present sentence. According to Bayoumi (2018), the average annual EUI for the library was 3,500 kWh/m2, which is too high compared to other cases. Even though the meteorological conditions and methods of application were the same in each of the three situations, (Bonnet et al., 2002; Khoshbakht et al., 2018; Chihib et al., 2020), the average annual EUI varied, see Table 5. The type of university, the type of energy used, the structure, the start date of study, and user behavior are possible factors.
TABLE 5. Average annual EUI for library, and cooling- and heating-degree days for different universities globally.
The present sentence discusses the typical yearly EUI for leisure and services. Although the climate index is similar between France and Spain, it is noted in Table 6. The EUI of sports activity venues is different, as reported by Chihib et al. (2020); Rey-Hernández et al. (2023) 47 kWh/m2 and 215 kWh/m2 in Spain, respectively, while Bonnet et al. (2002) stated 37 kWh/m2 for France. It is noted from Table 6 that the average annual EUI of university housing in areas is 39–100 kWh/m2 for all cases.
Most universities largely use electrical energy, except for those in cold climates, which use thermal energy for heating. Table 7 shows that there is a disconnect between consumption rates and effectiveness. Table 7 shows that (Ghenai and Bettayeb, 2019) in the United Arab Emirates (Alfaoyzan and Almasri, 2023b), in the middle of the Kingdom of Saudi Arabia, and Almeida et al. (2021) in Brazil consume a high amount of AC electricity. According to Alfaoyzan and Almasri (2023b) lighting, other devices, and AC systems account for 7, 14, and 79% of total energy use, respectively. The AC load is mostly at its maximum. It should be noted that claimed consumption percentages vary widely. This is most likely due to the weather, the building’s design, or the difficulty in determining the proportion of consumption based on use, as reported by Al-Tamimi (2022).
Climate plays a pivotal role in influencing electricity consumption, with regions experiencing extreme weather conditions requiring more energy for cooling purposes. In colder climates, energy-intensive heating systems may contribute to higher consumption. This weather-related variation in energy needs contributes to the reported disparities in consumption percentages. Building design and infrastructure also impact electricity consumption rates. Older buildings with outdated insulation and inefficient appliances may require more energy for heating, and cooling. Conversely, modern, energy-efficient structures equipped with smart technologies tend to have lower consumption rates. Therefore, the age and efficiency of building stock in a region can contribute significantly to reported variations in consumption figures. User behavior is another crucial element influencing electricity consumption. Cultural practices, lifestyle choices, and awareness of energy-efficient practices contribute to the differences observed in consumption percentages. Regions or countries with a higher emphasis on sustainability, energy conservation, and awareness campaigns tend to exhibit more efficient energy usage patterns. In addressing the wide variation in claimed consumption percentages, it is essential to recognize the multifaceted nature of the factors at play.
This has previously been investigated since there is a link between energy expenditure and reaching thermal comfort and air quality in interior conditions. At King Saud University (Saeed, 1993), investigated how well the thermal comfort conditions matched the criteria of the engineering and architecture colleges. According to the statistics, the majority of the samples were able to achieve interior temperature comfort levels of 23°C–29°C in the winter and 24°C–26°C in the summer, indicating that they are high in the winter and acceptable in the summer (Fabozzi and Dama, 2019). Conducted field research at the Polytechnic University of Milan, Italy, in classrooms with mechanical AC and natural ventilation to assess thermal comfort. They claimed that the Fanger model could reasonably predict thermal comfort in an air-conditioned environment. As opposed to this, it was a little bit higher in classrooms with enough natural ventilation as per ASHRAE 55 and temperatures as per EN 15251. To forecast the thermal comfort conditions of two university buildings, Universiti Teknologi Malaysia and Universiti Teknologi MARA, in Malaysia (Damiati et al., 2017), conducted field measurements and surveys. They enquired about thermal comfort filling from the employees, postgraduate researchers, and students. They reported the survey’s thermal sensation vote, which came in at 24.9°C, more than they had anticipated (Mahyuddin et al., 2013). Investigated the CO2 distribution effects of the indoor environment in four classrooms at the University of Reading. In addition to heights between 1 and 1.2 m, they claimed that higher CO2 concentration values can also be detected in a room at higher levels. Additionally, it was shown that the location of air supply and extract apertures, external and internal environmental conditions, the number of occupants, occupants’ sitting posture, occupancy times, and other characteristics could affect CO2 concentrations in addition to the room ventilation condition. The indoor air quality levels in university classrooms were quite bad during occupancy periods, according to Awbi (1995). The CO2 concentrations in this study were more than 1,000 parts per million (ppm), and in certain cases, amounts of more than 5,000 ppm were noted.
The thermal comfort conditions observed in different studies directly impact energy consumption patterns and indoor air quality. Achieving an optimal balance requires a holistic approach that considers building design, HVAC systems, occupant behavior, and smart technologies. Enhancing thermal comfort conditions while maintaining energy efficiency contributes to sustainable building practices and occupant wellbeing. Additionally, monitoring and improving indoor air quality, as reflected in CO2 concentration levels, are integral to creating healthier and more productive indoor environments.
The current paragraph addresses studies related to campus sustainability on a global scale. The relevant rules and technologies required for the decarbonization of the operation of the campus, it was introduced by Aghamolaei and Fallahpour (2023). The authors provided a thorough evaluation of significant parameters that are practical to take into account when developing new or renovating campuses. These elements include spatial planning and landscape, energy systems, clean and RE, building envelopes, green transportation, control and management, smartness, and human-related performance. The writers discussed the technologies and knowledge gaps that will help decarbonize campuses. The promotion of decarbonization and sustainable development is a major responsibility of higher education institutions. College campuses must develop energy-efficient and low-carbon projects (Renedo et al., 2014). Investigated the use of a cold water condenser in a fire tank instead of an air condenser for an AC system in a building at Spain’s University of Cantabria. They claimed that the system’s coefficient of performance increased and that the chiller’s annual energy consumption was reduced by 18.6%, demonstrating that the system is sustainable.
Amaral et al. (2020) reviewed the literature on efforts that have been put in place and case studies that focus on the most recent developments on sustainable campuses. The findings indicate that the two most widely implemented measures are the growth in energy generation on campus and the decrease in energy usage in buildings. According to the authors, energy and buildings account for the biggest percentage of efforts (29% and 28%, respectively), and there is a dearth of actual evidence from policies and activities. Campus sustainability problems can be attributed to technical, economic, climatic, and behavioral factors, according to Amaral et al. (2021). As a result, there is poor design, poor maintenance, low return on investment, and inconsistencies between the local weather and the guidelines. They also discussed methods for increasing sustainability on college campuses. They proposed creating an integrated framework to communicate impact, track the sustainability of notable efforts, and promote the goal of energy resource conservation on campus.
Mohammadalizadehkorde and Weaver (2018) made an effort to describe how colleges and universities presently manage sustainability, as well as how campuses might become more devoted to it, particularly in terms of energy use. The authors identified several thoroughly researched impediments to implementing sustainability efforts on college campuses, the majority of which are financial (Sufian Hasim et al., 2019). Addressed a variety of strategies used by various organizations to assist in reducing energy use in order to be sustainable. Load control, the use of prepayment metering systems, energy awareness programs, and the use of energy-efficient equipment and appliances were among the most commonly used techniques. Everyone involved in developing a sustainable campus at a college, according to the authors, must be committed to doing so (Leal Filho et al., 2019). Examined a sample of 50 higher education institutions globally to determine their involvement in ECMs and the types of RE being employed at the time. According to the findings, RE sources account for only 1%–20% of the energy used in more than half of the universities (PV accounts for 70% of all sources) (Abdou et al., 2019). Investigated the energy consumption of university buildings in order to devise a strategy for reducing energy consumption and transforming these structures into self-sustaining ones. They propose that the core of new building designs should be an energy awareness strategy that includes creating a department in charge of regulating and controlling energy use, encouraging the use of RE sources, and implementing smart building practices. At the Iranian University of Tehran, Heravi et al. (2021) created an eco-friendly model to measure the degree of ecological friendliness. According to their findings, the university’s overall grade point average grew by 64% between 2016 and 2020. They also learned how to reduce energy waste and regulate heating and cooling systems to increase the university’s energy sustainability (Olivieri et al., 2020). Evaluated the environmental, economic, and technological viability of utilizing photovoltaic to partially power a building at the Universidad Politécnica de Madrid in Spain. The findings revealed that the campus’s maximum solar potential, estimated to be roughly 3.3 MW, aligns with the ideal position of PV power to maximize pollution reductions and financial viability. As a result, around 40% of total electricity use—or approximately 77% of PV electricity—would be used locally (Hasapis et al., 2017). Gave information on the design of a 2 MWp grid-connected solar power plant on the campus of the Technical University of Crete in Greece. According to the authors’ assessment, the campus consumed between 3,700 and 4,400 MWh of electricity each year from 2009 to 2014.423 kW of power must be created to produce 3,700 MWh of energy every year. When the first phase of the project is completed, it will generate approximately 1,899 MWh of power each year. The authors suggested that more research be conducted on possible investors’ involvement in the development of this initiative.
Yıldırım et al. (2006); Yildirim et al. (2010) thoroughly researched a geothermal heating system case study because a 33°C geothermal source is adjacent to the Izmir Institute of Technology campus. The authors demonstrate how the proximity of the source results in lower operating and investment expenses. A heat pump central heating system has the greatest initial cost but the lowest recurrent expenses, according to the research. The options were assessed using the internal rate of return technique, which calculates investment profit. When compared to a central heating system with fuel boilers, the heat pump system created a profit margin of at least 3.02% over 20 years. The study by Niemelä et al. (2016) looked at the financial sustainability of several regeneration efforts performed on the campus of Finland’s Lappeenranta University of Technology. For newly constructed almost zero-energy educational buildings, the recommended energy performance target level was close to the cost-optimal energy performance level. According to the study, solar-powered PV power generation systems provide the greatest gains in EE for educational buildings.
At Sulaiman Al-Rajhi University in the KSA (Alfaoyzan and Almasri, 2023a), examined the use of PV solar energy. There were two PV Solar System installation scenarios considered: creating a system that would cover every building on campus and installing a system that would secure the university for almost no energy expenses. To achieve Nearly Zero-Bill Campus, the first scenario produces around 24% of the load, while the second scenario produces approximately 113% of the load. According to the authors, the Levelized Cost of Energy (LCOE) ranges from 0.026 to 0.028 US dollars per kWh (Castrillón-Mendoza et al., 2020). From Colombia’s University Autónoma de Cali used a cutting-edge energy technology model to investigate the problem of photovoltaic integration as the cornerstone of an energy-producing system. The authors demonstrated the PV system’s energy supply and demand for the university campus. Even in the month with the highest energy production, the PV subsystem only produced 5.6 MWh/month, despite their estimate that the energy requirement is greater than 200 MWh/month (Bayoumi, 2018). Assessed the use of PVs to generate electricity, solar thermal energy for cooling, and a hybrid system that incorporates both technologies at the KAU main campus in Jeddah, KSA. Numerous assessments of potential energy outputs have found optimistic results in the energy demand sectors of cooling and electrical energy. It is suggested that an energy policy be developed in collaboration with stakeholders at various levels in order to establish synergy between passive/active solutions to meet user comfort while using the least amount of energy and technological complexity in existing and future buildings (Zeidan et al., 2023). Researched how Jordan’s electrical grid will be affected by the inclusion of pumped hydroelectric energy storage systems and PV. The authors looked at the locations of this system installation at Mutah University in Jordan. Based on the findings of this study, it can be concluded that the system would meet the annual need of Mutah University (9,230.89 MWh) and save $287,607,993.
Education for sustainable development is seen as a crucial component of quality, according to Fernandez-Antolin et al. (2021). Nonetheless, soon-to-be graduates of architecture programs believe that cutting-edge instructional resources, like building simulation performance tools, were lacking during their training. The authors concluded that the use of building simulation performance tools had to be quickly incorporated into the syllabuses of the architectural project topics within the degree in architecture, and they recommended universities take action on this matter. To achieve sustainability on the campus of Al-Azhar University, Egypt (Soliman and Mehanna, 2023), reviewed local and worldwide studies, documentation, and guidelines on sustainability. The findings indicate that a percentage of 53.64 percent reduction in the building’s electricity consumption was achieved. An average of the building’s consumption, or around 83.9 percent of its yearly energy demand, was generated by a solar energy unit. With the revised scenario they proposed, they calculated a 53.64 percent reduction in CO2 carbon emissions. According to Lauder et al. (2015), there is not much information about campus sustainability in higher education on the worldwide rankings. They provided an analysis of the GreenMetric World University Ranking. The rating was criticized for failing to take into account the trade-off between practical and scientific considerations, university rankings, and different interpretations of sustainability. The analysis improved understanding of the term sustainability by concentrating on conceptual problems related to its definition (Quevedo et al., 2024). Mentioned that the complexity of university campuses and the emphasis on standards based on end-use and space use in future research make it challenging to apply the standard there. Further studies should increase the complexity of development to benchmark the energy consumption by end-uses; this can help identify the systems with high energy demand develop specific retrofit strategies; and fill the gaps in this research field by developing more representative benchmarking.
The following material discusses international research on hybrid systems. Numerous studies on hybrid systems for energy production have also been conducted in institutions such as colleges (Muqeet et al., 2021). Presented a techno-economic assessment of the most recent research on campus microgrid systems. Scenarios for wind, energy storage, solar PV, and electric vehicle charging and discharging were discussed. The authors investigated various energy-harvesting components and identified issues with the campus microgrid. They claimed that many campus microgrids around the world lack proper energy management. Eisapour et al. examined an optimally smart hybrid RE system to suit Shiraz University’s Eram Campus’s heating and electrical needs in Iran (Eisapour et al., 2021). As a result of the findings, the proposed system includes a combined power plant, thermal boilers, converters, a solar array, pumped hydro storage units, and a predictive controller. The Campus’s annual carbon dioxide emissions are decreased by 8,000 megatonnes when compared to using the national energy grid (Ghenai and Bettayeb, 2019). Investigated the efficacy of a freestanding hybrid power system at the administration building of the University of Sharjah in the United Arab Emirates. This system provides the optimum mix of cheap energy costs (92 cents per MWh) and excellent energy security (between 66.1% and 75.8%). According to the findings, the recommended adjustments might provide a RE percent of 66.1%–75.8%. The authors propose that better tools be used to improve system performance to reduce demand and that dust-cleaning solar PV technology be used to increase supply. According to the authors, lighting accounts for 10% of annual electricity consumption, while the HVAC system accounts for 72%.
Chedid et al. (2020) used dynamic programming to design a unique optimization technique on the American University of Beirut campus in Lebanon. The results showed that installing optimal PV and battery system capacities essentially eliminated the need for diesel generators, leaving only 3.5% of the system’s expected capacity. The proposed solution lowered the system’s total lifespan costs from 13.7 cents per kWh in the first year to 8.8 cents per kWh in the first year and 14.4 cents per kWh in the 10th year (Mokhtara et al., 2021). Used Hybrid Optimization of Multiple Energy Resources (HOMER) software to design a grid-connected hybrid RE system that maximized self-sufficiency for a university campus in Ouargla, Algeria. This is seen by the outcomes of the first choice, which sets a 100% maximum for non-renewable usage. A PV system without hydrogen (H2) with an LCOE of 0.016 $/kWh, establishing grid parity and consuming 13% non-renewable energy is the best choice. However, by limiting the maximum non-renewable consumption of the second option to 1%, an optimal configuration of the grid, PV, electrolyzer, fuel cell, and storage tank is created. The LCOE of 0.1 dollars per kWh and a non-renewable usage of 0% was achived. In second scenario, a considerable decrease in LCOE and non-RE consumption is found after an increase in H2 demand. As a result, while considering economic issues, it is argued that the grid/PV combination is the best option for the system under evaluation. However, given the increasing demands of future energy systems, grid-connected PV with H2 will be the best option.
Tazay (2021) conducts a feasibility analysis for grid-connected hybrid RE system designs using HOMER software at four different institutions in the Kingdom of Saudi Arabia. The results reveal that integrating a 62 kW PV array with a grid is the best solution, with a low LCOE of 0.0688 $/kWh and sell-back energy accounting for 9.16% of Al Baha University’s total energy consumption. The LCOEs for Tabuk, Sattam, and Jeddah were $0.0714, $0.0753, and $0.0702, respectively, for the remaining institutions. Furthermore, optimization modeling considers the fact that a hybrid RE system can only supply the total load demand without running out of electricity, with battery banks accounting for 10.2%, wind energy accounting for 11.3%, and solar PV accounting for the rest of the 78.5% contribution. Using HOMER software (Baseer et al., 2019), investigated the potential application of hybrid energy systems, such as PV/wind/diesel with and without battery, for three compounds in Jubail Industrial City, KSA. Three complexes were evaluated, with peak loads of 685, 463, and 270 kW. The minimal LCOE for the hybrid energy systems for compounds 1, 2, and 3 was found to be 0.183, 0.224, and 0.244 US$/kWh, respectively. The lowest LCOE for PV/wind/battery solutions for all three compounds was discovered to be 0.25 US dollars/kWh with 100% RE penetration.
Wiryadinata et al. (2019) suggested a method for determining the important technological and economic elements impacting the adoption of a carbon-neutral energy system by 2025 at the University of California, Davis (UCD), United States. As system configurations, they concentrated on heat pumps, solar energy, biomass boilers, and coupled cycles. The analysis period is from 2016 to 2035, with equipment phasing limited to 2020–2025 and capacity limited to peak campus demands of 40 MWe for electricity and 80 MWt for thermal. According to the authors, regulating the supply-demand imbalance is the key impediment to UCD’s high renewable penetration rate; as a result, modifying thermal storage and production systems is critical. All alternate instances are expected to cost between $629 million and $704 million compared to the basic 20-year current cost of $634 million. According to Opel et al. (2017), in 2014, Leuphana University in Lueneburg, Germany, made the switch to RE and created the first climate-neutral energy balance. The heating network is built around two combined heat and power plants, each with a 525 kWel output. PV output of 720 kWp can supply more than 20% of the required electricity, assuming a 95% self-consumption rate. A high-temperature aquifer thermal energy storage project might generate an additional 2.3 GWh/a of renewable energy (Şevik, 2022). Focused on reducing campus energy use and employing RE to establish a green campus at the Hitit University Vocational College in Orum, Turkey. PV utilization and integration with a gas-fired three-generation system, as well as solar hydrogen generation without energy storage, were also considered on campus. Three alternative situations were constructed using simulation from design software (HelioScope). Based on the modeling results, the PV system may be able to maintain it by producing up to 25% more electrical energy than the existing system. The levelized cost of hydrogen generation on campus—a place with significant solar potential—ranged from 0.054 $/kWh to 0.103 $/kWh (Esfilar et al., 2021). Evaluated the techno-economic feasibility of hybrid systems integrating photovoltaics, wind turbines, and biomass gasifiers, as well as multiuse waste-to-energy technologies at University of Victoria campus. To determine the most cost-effective system layout, the HOMER simulation software was employed. The hybrid system is complete with 46 batteries, a 250 kW biomass gasifier, 175 kW wind, and 245 kW solar electricity. According to the authors, the LCOE was 0.129 cents per kWh. Table 8 lists the electrical systems of university buildings from throughout the world. A variety of factors can explain the variation in indices, including capacity, source fractions, climate, economic concerns, system makeup, and research period.
The current sentence discusses research on simple payback time (SPT) conducted globally. The payback period for energy-saving strategies and single or hybrid RE installations is discussed in the present paragraph (Levy et al., 2015). Reported that using the three ECMs (lowering the cleanroom freeze stat setpoint temperature, lowering the air change rate, and using exhaust air energy recovery), can cut cleanroom energy consumption by an estimated 21.6 percent and it is predicted that the project’s SPT will be less than 2 years (Mohamed et al., 2022). Proposed energy analysis techniques for Princess Sumaya University for Technology in Jordan. The first of the two possible ECMs is based on optimization AC operating times, which results in an average savings of 12.5% and a SPT of 9.27 years. Thermal building insulation, the second strategy, achieves cost savings of 38% with an SPT ranging from 2.89 to 19.16 years (Celniker et al., 2021). Estimated the potential energy savings from cool paint improvements for 49 buildings on the main campus of the University of California, Davis, United States. The authors estimated that cool paints could improve 35 percent of campus buildings. Up to 861.11 MWh of energy might be saved by the university annually. If cool paints are chosen when buildings are routinely repainted, SPT would be nil because light-colored paints do not cost any more than dark paints. The straightforward SPT for some campus projects is shown in Table 9. Following is a summary of the findings:
❖ The simple SPTs in energy-saving schemes are less than in RE systems.
❖ Solar PV systems have better SPT than other renewable systems, even hybrid ones.
❖ On the other hand, the SPT for wind systems is the highest in the studies presented for this application (not a large capacity).
The present paragraph highlights the influence of energy-saving measures on campus, as well as the use of single or hybrid RE systems. The largest energy savings were measured by Samira and Nurmammad (2018) at the University of Azerbaijan. According to the study, energy savings ranging from 20% to 56% had a significant positive influence on the environment (Batlle et al., 2020). Proposed a methodology for establishing energy baselines and energy performance indicators in higher education institution buildings using ISO 50001: 2011 and ISO 50006: 2014 recommendations in order to identify potentials for electrical energy consumption reduction in buildings. At Federal University of Itajubá in Brazil, the study was put into practice in three buildings. According to the findings, without making any financial investments, the campus’s yearly electric energy usage may be reduced by 7.19%–9.6%, resulting in a 20.3 CO2eq reduction. At the University of Valladolid in Spain (Rey-Hernández et al., 2023), discussed the energy renovation project to create campuses with a low carbon dioxide emissions level. Only 317 tonnes of CO2 are produced annually, which is 92.69% less than was the case when the outdated heating system was in use (Mohamed et al., 2020). Addressed the important elements that should be considered in the environmental sustainability initiatives plan. According to the authors, to evaluate the effectiveness of the plan, all parties involved in the university—students, employees, management, and the local community—must make sure that periodic monitoring can be carried out with complete cooperation from all stakeholders (Leon et al., 2018). Used the Neighbourhood Evaluation for Sustainable Territories to discuss the environmental effects of the four largest university campuses in the Basque Country, Spain. The evaluation started with a baseline environmental impact analysis, followed by a variety of campus renovation scenarios presented following national and international declarations regarding sustainable development in higher education. Finally, consideration was given to the Corporate Carbon Footprint. The relationship between faculty members and CO2 emissions at the University of Agriculture in Faisalabad, Pakistan, was investigated by Ali et al. (2018). They stated that males generate more CO2 than females and that faculty members’ average annual CO2 emissions were 10.06 metric tons (mt) (Makino et al., 2021). Assessed how a Tokyo University of Agriculture and Technology in Japan campus energy use reduced greenhouse gas (GHG) emissions. A life-cycle analysis was used to examine the GHG balance. Compared to the current off-campus power generation system, using pruned branches for heating on campus reduced GHG emissions by 70%–180% (Kalkan et al., 2011). Concentrated on the University of Southampton’s Highfield Campus in England to develop a more environmentally friendly method of producing heat and electricity. The proposed designs are technically and financially feasible, thus the results were satisfying in that regard.
At Sulaiman Al-Rajhi University in the Kingdom of Saudi Arabia (Alfaoyzan and Almasri, 2023a), examined the use of PV solar energy. According to the authors, the second scenario can reduce CO2 emissions by 7,170.075 tons per year. Tsinghua University was used as a case study by Li and Jia (2022) to analyze the campus’s ability to emit and absorb CO2 in order to achieve carbon neutrality while still in operation. The authors concluded that carbon neutrality might be attained by placing PV panels on the ground, on the roofs of buildings, and their south sides, while enhancing plant life on the east, west, and north sides of buildings. This came after a quantitative analysis of the CO2 emissions and reductions induced by the installation of solar PV panels and the expansion of plant life. The scientists also suggested the idea of combining PV panel installation and plant growing with other renewable technologies to reduce CO2 emissions even further (Olivieri et al., 2020). evaluated the environmental, economic, and technological viability of utilizing photovoltaic to partially power a building at the Universidad Politécnica de Madrid in Spain. Based on the accounting technique used for the characteristics of the power produced, the expense would allow for a yearly reduction in CO2 emissions of 619–1,400 tCO2eq, or 13%–30% less than the campus’s emissions in 2016.
Ghenai and Bettayeb (2019) investigated the efficacy of a freestanding hybrid power system at the administration building of the University of Sharjah in the United Arab Emirates. This system provides the best feasible balance of low energy costs (92 cents per MWh), high energy security (renewable component between 66.1% and 75.8%), and low GHG emissions (24 kg of CO2 per MWh) (Mokhtara et al., 2021). Developed a grid-connected hybrid RE system in Ouargla, Algeria, to generate power and H2 while maximizing self-sufficiency for a university campus. When H2 demand increases, the authors claim that LCOE, non-RE consumption, and CO2 emissions decrease significantly. More thorough research on hybrid energy systems is needed, according to the researchers, to demonstrate that the optimal technological, financial, and environmental conditions are met.
Baseer et al. (2019) evaluated the potential application of hybrid energy systems for three compounds in Jubail Industrial City, Saudi Arabia. The ideal strategy, according to the authors, will reduce CO2 emissions by 2,800 tons per year. According to Opel et al. (2017), in 2014, Leuphana University in Lueneburg, Germany, transitioned to RE and established the first energy balance. A high-temperature aquifer thermal energy storage project can reduce annual CO2 emissions by 2.424 t. (Şevik, 2022) of Turkey’s Hitit University Vocational College examined the use of RE in the creation of a green campus in Çorum. According to the findings, the campus may reduce its GHG emissions by 1,546–2,272 tons CO2 (Esfilar et al., 2021). Investigated the techno-economic feasibility of hybrid systems and multiuse waste-to-energy technology on the University of Victoria campus. According to the experts, using the hybrid system they offer will save 1,039 tons of CO2 (Husein and Chung, 2018). Designed a RE microgrid for Seoul National University’s Gwanak Campus in South Korea. According to the research, a perfect microgrid could lower energy costs by 42% while also reducing emissions by 15%. With an 18% capacity factor and a benefit-to-cost ratio of 1.2–1.5, the ideal net present value of a 125-kW PV system is $420,727 (Kourgiozou et al., 2021). Conducted an assessment of smart energy systems implemented on UK higher education campuses. They underlined that the structure currently in place in higher education facilities focuses on reducing carbon emissions rather than integrating smart energy technologies. The authors emphasized the importance of a clear, consistent structure in order to accomplish the campus’s main objectives (Tian et al., 2022). Demonstrated the ideal energy system layout for fulfilling seasonal demands for electricity, heat, cooling, and carbon-neutral systems in a sustainable manner. A series of case studies highlight various applications of the optimization process using Cornell University’s main campus in New York as the living lab. According to the authors, emissions in 2020 will range from 8% to 17% of the value.
Soares et al. (2015) conducted an energy efficiency evaluation for the Teaching Building of the Faculty of Economics at the University of Coimbra in Portugal. According to the authors, implementing lighting system optimization approaches would eliminate 3,704 kg CO2 emissions and reduce annual electricity use by approximately 26,123 kWh (Bellia et al., 2018). Provided a process for developing energy refurbishment plans, following which the Cost-Optimal technique was used to assess alternative retrofit scenarios. The case study’s subject, Edificio Polifunzionale, is located in southern Italy and houses the administrative offices and classrooms of the University of Molise’s law department. The installation of heat recovery systems, HVAC regulation dispositive, and LED lighting with automatic control, among other energy-efficient measures, according to the authors, results in energy savings, a 33% reduction in polluting emissions, and a 35% reduction in overall expenditures (Erasmus and Maritz, 2023). Proposed constructing a campus microgrid with a bedrock thermal storage system capable of supporting a 1,380 kW peak demand in order to recover heat from diesel engines. PV was employed alongside diesel engines at the University of the Free State Qwaqwa campus in South Africa’s Phuthaditjhaba region. The authors claimed that because there is an alternative thermal energy source present, it is possible to utilize the heat produced by the system on the university campus and minimize carbon emissions (Celniker et al., 2021). Estimated the environmental benefits of cool paint on the main campus of the University of California. According to the authors, cool paints could enhance 35% of campus structures. The institution may prevent 86 metric tons of CO2-eq emissions each year.
There is a well-established relationship between environmental management and sustainable practices. Environmental management refers to the process of planning, implementing, and monitoring an organization’s activities to ensure they are environmentally sustainable. Sustainable practices, on the other hand, involve conducting activities in a way that meets the needs of the present without compromising the ability of future generations to meet their own needs. The research provided shows that using RE sources or implementing energy-saving measures results in positive environmental effects. The general environmental impact of the production, installation, and transportation procedures was ignored. To completely elucidate the situation, more research is necessary.
Preserving ecosystems is facilitated by both energy conservation and REproduction. Combatting climate change necessitates the reduction of energy consumption and the implementation of energy efficiency measures, which are crucial initial steps. Multiple studies highlight the technological, economic, and environmental benefits of adopting EERES. The study’s findings resulted in the subsequent conclusions:
❖ The energy consumption on a campus of higher education institutions is subject to various influencing factors, such as the institution’s type, the energy sources employed, the characteristics and structures of its facilities, occupant activities within buildings, prevailing weather conditions, and user behavior.
❖ There is a significant commitment to the United Nations Sustainable Development Agenda by numerous educational institutions worldwide. However, there is an urgent need to further bolster this commitment at a broader scale within high educational institutions, given their influential role in this domain and the heightened expectations placed upon them as leaders in advancing sustainable practices.
❖ High educational institutions investing in energy-efficient technologies experience substantial reductions in utility bills, leading to long-term cost savings. Additionally, the decreased reliance on fossil fuels mitigates GHG emissions, contributing to environmental sustainability.
❖ Beyond economic and environmental gains, EERES in educational facilities offers unique educational opportunities. Integrating these systems into curricula fosters interdisciplinary learning, allowing students to engage with real-world sustainable technologies. Moreover, student-led initiatives, such as campus-wide EE campaigns and RE projects, empower learners as sustainability advocates.
❖ The literature on EERES in high-educational facilities illustrates a growing global awareness of the importance of sustainable energy practices. While challenges persist, the economic, environmental, and educational benefits underscore the need for continued research, policy advocacy, and collaborative efforts among high educational institutions, governments, and industry stakeholders.
❖ The literature anticipates several future trends in EERES implementation, such as in energy storage technologies, artificial intelligence applications in building management, and innovative financing mechanisms. Moreover, there is a growing emphasis on community engagement, wherein educational institutions collaborate with local communities to create RE microgrids, ensuring energy resilience and sustainability.
❖ Many studies focus solely on achieving carbon neutrality during campus operations without considering the development of university buildings or material production. Greater emphasis on these aspects is essential to advance carbon neutrality efforts. Future research in this area is warranted.
❖ It is important to note that specific recommendations can vary based on the educational institution’s goals, the region’s regulatory framework, and the unique needs of the community. The involvement of architects, sustainability experts, and other stakeholders in the planning and design process is crucial for developing effective and sustainable higher education facilities.
❖ We believe that our work provides a knowledge foundation for policymakers and industry stakeholders, facilitating informed decision-making. By highlighting the commitment of educational institutions to environmental stewardship and sustainable practices, our study aims to inspire collaborative efforts between stakeholders to create a positive societal impact. By publishing this review paper, the authors look forward to contributing to the ongoing discourse on sustainable energy practices in higher education and fostering collaborative initiatives for a greener and more sustainable future.
RA: Investigation, Project administration, Resources, Writing–original draft, Methodology. NA-H: Supervision, Validation, Visualization, Writing–review and editing, Investigation. NA-T: Funding acquisition, Investigation, Methodology, Writing–review and editing, Validation.
The author(s) declare that no financial support was received for the research, authorship, and/or publication of this article.
The researchers would like to thank the Deanship of Scientific Research, Qassim University for funding the publication of this project.
The authors declare that the research was conducted in the absence of any commercial or financial relationships that could be construed as a potential conflict of interest.
All claims expressed in this article are solely those of the authors and do not necessarily represent those of their affiliated organizations, or those of the publisher, the editors and the reviewers. Any product that may be evaluated in this article, or claim that may be made by its manufacturer, is not guaranteed or endorsed by the publisher.
ASHRAE, American Society of Heating, Refrigerating and Air-Conditioning Engineers; AC, Air Conditioning; CDD, Cooling Degree Days; DHS, District Heating System; ECMs, Energy Conservation Measures; EE, Energy Efficiency; EERES, Energy Efficiency and Renewable Energy Systems; EUI, Energy Use Intensity; GHG, Greenhouse Gas; FC, Fuel Cell; HDD, Heating Degree Days; HOMER, Hybrid Optimization of Multiple Energy Resources; HVAC, Heating, Ventilation, and Air Conditioning; KAU, King Abdulaziz University; KSA, Kingdom of Saudi Arabia; LCOE, Levelized Cost of Energy, NEST, Neighbourhood Evaluation for Sustainable Territories; PPM, parts per million (ppm); PV, Photovoltaics; RE, Renewable Energy; SPT, Simple Payback Time; UCD, University of California, Davis; UK, United Kingdom; UN, United Nations.
Abdo-Allah, A., Iqbal, M. T., and Pope, K. (2019). Energy consumption analysis of a large building at memorial university. J. Energy 2019, 1–21. doi:10.1155/2019/5243737
Abdou, A. A., Abd El Gwad, I. O., and Mahmoud, A. A. (2019). Reducing energy consumption strategies in university buildings in Egypt. Acad. Res. Community Publ. 2, 112–125. doi:10.21625/archive.v2i3.351
Aghamolaei, R., and Fallahpour, M. (2023). Strategies towards reducing carbon emission in university campuses: a comprehensive review of both global and local scales. J. Build. Eng. 76, 107183. doi:10.1016/j.jobe.2023.107183
Alfaoyzan, F. A., and Almasri, R. A. (2023a). Utilizing solar photovoltaic systems in educational facilities to achieve a zero bill campus: the Sulaiman Al-Rajhi University case. Energy Explor. Exploitation 41 (4), 1415–1438. doi:10.1177/01445987231174767
Alfaoyzan, F. A., and Almasri, R. A. (2023b). Benchmarking of energy consumption in higher education buildings in Saudi Arabia to Be sustainable: sulaiman Al-rajhi university case. Energies 16, 1204. doi:10.3390/en16031204
Ali, G., Anbren, S., and Bashir, M. K. (2018). Climate mitigation, low-carbon society, and dynamism of educational institutes in a low-income country. Environ. Sci. Pollut. Res. Int. 25 (4), 3775–3784. doi:10.1007/s11356-017-0607-9
Ali, S. B. M., Hasanuzzaman, M., Rahim, N., Mamun, M., and Obaidellah, U. (2021). Analysis of energy consumption and potential energy savings of an institutional building in Malaysia. Alexandria Eng. J. 60 (1), 805–820. doi:10.1016/j.aej.2020.10.010
AlKassem, A., Draou, A., Alamri, A., and Alharbi, H. (2022). Design analysis of an optimal microgrid system for the integration of renewable energy sources at a university campus. Sustainability 14, 4175. doi:10.3390/su14074175
Almeida, A. P., Sousa, V., and Silva, C. M. (2021). Methodology for estimating energy and water consumption patterns in university buildings: case study, Federal University of Roraima (UFRR). Heliyon 7 (12), e08642. doi:10.1016/j.heliyon.2021.e08642
Al-Tamimi, N. (2017). A state-of-the-art review of the sustainability and energy efficiency of buildings in Saudi Arabia. Energy Effic. 10 (5), 1129–1141. doi:10.1007/s12053-017-9507-6
Al-Tamimi, N. (2022). Building envelope retrofitting strategies for energy-efficient office buildings in Saudi Arabia. Buildings 12, 1900. doi:10.3390/buildings12111900
Amaral, A. R., Rodrigues, E., Gaspar, A. R., and Gomes, Á. (2020). A review of empirical data of sustainability initiatives in university campus operations. J. Clean. Prod. 250, 119558. doi:10.1016/j.jclepro.2019.119558
Amaral, A. R., Rodrigues, E., Gaspar, A. R., and Gomes, Á. (2021). Lessons from unsuccessful energy and buildings sustainability actions in university campus operations. J. Clean. Prod. 297, 126665. doi:10.1016/j.jclepro.2021.126665
Amber, K. P., Aslam, M. W., Mahmood, A., Kousar, A., Younis, M. Y., Akbar, B., et al. (2017). Energy consumption forecasting for university sector buildings. Energies 10 (10), 1579. doi:10.3390/en10101579
Awbi, A. J. (1995). “Ventilation and energy conservation in buildings,” in Second international conference, proceedings indoor air quality (Montreal: Springer).
Baseer, M. A., Alqahtani, A., and Rehman, S. (2019). Techno-economic design and evaluation of hybrid energy systems for residential communities: case study of Jubail industrial city. J. Clean. Prod. 237, 117806. doi:10.1016/j.jclepro.2019.117806
Batlle, E. A. O., Escobar Palacio, J. C., Silva Lora, E. E., Martínez Reyes, A. M., Melian Moreno, M., and Morejón, M. B. (2020). A methodology to estimate baseline energy use and quantify savings in electrical energy consumption in higher education institution buildings: case study, Federal University of Itajubá (UNIFEI). J. Clean. Prod. 244, 118551. doi:10.1016/j.jclepro.2019.118551
Bayoumi, M. (2018). Potential of integrating power generation with solar thermal cooling to improve the energy efficiency in a university campus in Saudi Arabia. Energy and Environ. 31 (1), 130–154. doi:10.1177/0958305x18787271
Bellia, L., Borrelli, M., De Masi, R. F., Ruggiero, S., and Vanoli, G. P. (2018). University building: energy diagnosis and refurbishment design with cost-optimal approach. Discussion about the effect of numerical modelling assumptions. J. Build. Eng. 18, 1–18. doi:10.1016/j.jobe.2018.02.017
Birch, C., Edwards, R., Mander, S., and Sheppard, A. (2019). Assessing unregulated electricity consumption in a case study university. Build. Serv. Eng. Res. Technol. 41 (3), 305–314. doi:10.1177/0143624419891554
Bonnet, J.-F., Devel, C., Faucher, P., and Roturier, J. (2002). Analysis of electricity and water end-uses in university campuses: case-study of the University of Bordeaux in the framework of the Ecocampus European Collaboration. J. Clean. Prod. 10 (1), 13–24. doi:10.1016/s0959-6526(01)00018-x
Bourdeau, M., Guo, X., and Nefzaoui, E. (2018). Buildings energy consumption generation gap: a post-occupancy assessment in a case study of three higher education buildings. Energy Build. 159, 600–611. doi:10.1016/j.enbuild.2017.11.062
Castrillón-Mendoza, R., Manrique-Castillo, P. A., Rey-Hernández, J. M., Rey-Martínez, F. J., and González-Palomino, G. (2020). PV energy performance in a sustainable campus. Electronics 9, 1874. doi:10.3390/electronics9111874
Celniker, C., Chen, S., Meier, A., and Levinson, R. (2021). Targeting buildings for energy-saving cool-wall retrofits: a case study at the University of California, Davis. Energy Build. 249, 111014. doi:10.1016/j.enbuild.2021.111014
Chalfoun, N. (2014). Greening university campus buildings to reduce consumption and emission while fostering hands-on inquiry-based education. Procedia Environ. Sci. 20, 288–297. doi:10.1016/j.proenv.2014.03.036
Chang, S., Saha, N., Castro-Lacouture, D., and Yang, P. P. J. (2019). Multivariate relationships between campus design parameters and energy performance using reinforcement learning and parametric modeling. Appl. Energy 249, 253–264. doi:10.1016/j.apenergy.2019.04.109
Chedid, R., Sawwas, A., and Fares, D. (2020). Optimal design of a university campus micro-grid operating under unreliable grid considering PV and battery storage. Energy 200, 117510. doi:10.1016/j.energy.2020.117510
Chen, S., Ren, Y., Friedrich, D., Yu, Z., and Yu, J. (2021). Prediction of office building electricity demand using artificial neural network by splitting the time horizon for different occupancy rates. Energy AI 5, 100093. doi:10.1016/j.egyai.2021.100093
Chihib, M., Salmerón-Manzano, E., and Manzano-Agugliaro, F. (2020). Benchmarking energy use at university of Almeria (Spain). Sustainability 12 (4), 1336. doi:10.3390/su12041336
Chung, M. H., and Rhee, E. K. (2014). Potential opportunities for energy conservation in existing buildings on university campus: a field survey in Korea. Energy Build. 78, 176–182. doi:10.1016/j.enbuild.2014.04.018
Costa, M. L. d.S., Freire, M. R., Silva, K. F. d., and Freires, F. G. M. (2022). Analysis of energy consumption related to the use of air conditioning in university buildings in a tropical climate. Rev. Gestão Soc. Ambient. 16 (2), e03025. doi:10.24857/rgsa.v16n2-019
Damiati, S. A., Zaki, S. A., Rijal, H. B., and Razak, A. A. (2017). “Field study of thermal comfort in university buildings in Malaysia,” in Proceedings of the 33rd PLEA International Conference: Design to Thrive - PLEA 2017, Edinburgh, January 2017, 1029–1036.
Davis, J. A., and Nutter, D. W. (2010). Occupancy diversity factors for common university building types. Energy Build. 42 (9), 1543–1551. doi:10.1016/j.enbuild.2010.03.025
Deng, Y., Gou, Z., Gui, X., and Cheng, B. (2021). Energy consumption characteristics and influential use behaviors in university dormitory buildings in China's hot summer-cold winter climate region. J. Build. Eng. 33, 101870. doi:10.1016/j.jobe.2020.101870
Droutsa, K. G., Kontoyiannidis, S., Dascalaki, E., and Balaras, C. (2017). Benchmarking energy use of existing hellenic non-residential buildings. Procedia Environ. Sci. 38, 713–720. doi:10.1016/j.proenv.2017.03.153
Eisapour, A. H., Jafarpur, K., and Farjah, E. (2021). Feasibility study of a smart hybrid renewable energy system to supply the electricity and heat demand of Eram Campus, Shiraz University; simulation, optimization, and sensitivity analysis. Energy Convers. Manag. 248, 114779. doi:10.1016/j.enconman.2021.114779
Emil, F., and Diab, A. (2021). Energy rationalization for an educational building in Egypt: towards a zero energy building. J. Build. Eng. 44, 103247. doi:10.1016/j.jobe.2021.103247
Energy Star (2023). What is energy use intensity (EUI). Available at: https://www.energystar.gov/buildings/benchmark/understand_metrics/what_eui (Accessed April 11, 2023).
Erasmus, S., and Maritz, J. (2023). A carbon reduction and waste heat utilization strategy for generators in scalable PV—diesel generator campus microgrids. Energies 16, 6749. doi:10.3390/en16186749
Esfilar, R., Bagheri, M., and Golestani, B. (2021). Technoeconomic feasibility review of hybrid waste to energy system in the campus: a case study for the University of Victoria. Renew. Sustain. Energy Rev. 146, 111190. doi:10.1016/j.rser.2021.111190
Esmaeil, K. K., Alshitawi, M. S., and Almasri, R. A. (2019). Analysis of energy consumption pattern in Saudi Arabia’s residential buildings with specific reference to Qassim region. Energy Effic. 12 (8), 2123–2145. doi:10.1007/s12053-019-09806-x
Fabozzi, M., and Dama, A. (2019). Field study on thermal comfort in naturally ventilated and air-conditioned university classrooms. Indoor Built Environ. 29 (6), 851–859. doi:10.1177/1420326x19887481
Fernandez-Antolin, M.-M., del Río, J. M., and Gonzalez-Lezcano, R.-A. (2021). The use of gamification in higher technical education: perception of university students on innovative teaching materials. Int. J. Technol. Des. Educ. 31 (5), 1019–1038. doi:10.1007/s10798-020-09583-0
Gaspar, K., Gangolells, M., Casals, M., Pujadas, P., Forcada, N., Macarulla, M., et al. (2022). Assessing the impact of the COVID-19 lockdown on the energy consumption of university buildings. Energy Build. 257, 111783. doi:10.1016/j.enbuild.2021.111783
Ghenai, C., and Bettayeb, M. (2019). Modelling and performance analysis of a stand-alone hybrid solar PV/Fuel Cell/Diesel Generator power system for university building. Energy 171, 180–189. doi:10.1016/j.energy.2019.01.019
Guan, J., Nord, N., and Chen, S. (2016). Energy planning of university campus building complex: energy usage and coincidental analysis of individual buildings with a case study. Energy Build. 124, 99–111. doi:10.1016/j.enbuild.2016.04.051
Gui, X., Gou, Z., and Lu, Y. (2021). Reducing university energy use beyond energy retrofitting: the academic calendar impacts. Energy Build. 231, 110647. doi:10.1016/j.enbuild.2020.110647
Hamida, M. B., Ahmed, W., Asif, M., and Almaziad, F. A. (2021). Techno-economic assessment of energy retrofitting educational buildings: a case study in Saudi Arabia. Sustainability 13 (1), 179. doi:10.3390/su13010179
Hasapis, D., Savvakis, N., Tsoutsos, T., Kalaitzakis, K., Psychis, S., and Nikolaidis, N. P. (2017). Design of large scale prosuming in Universities: the solar energy vision of the TUC campus. Energy Build. 141, 39–55. doi:10.1016/j.enbuild.2017.01.074
Heravi, G., Aryanpour, D., and Rostami, M. (2021). Developing a green university framework using statistical techniques: case study of the University of Tehran. J. Build. Eng. 42, 102798. doi:10.1016/j.jobe.2021.102798
Husein, M., and Chung, I.-Y. (2018). Optimal design and financial feasibility of a university campus microgrid considering renewable energy incentives. Appl. Energy 225, 273–289. doi:10.1016/j.apenergy.2018.05.036
Jami, S., Forouzandeh, N., Zomorodian, Z. S., Tahsildoost, M., and Khoshbakht, M. (2021). The effect of occupant behaviors on energy retrofit: a case study of student dormitories in Tehran. J. Clean. Prod. 278, 123556. doi:10.1016/j.jclepro.2020.123556
Jradi, M., Sangogboye, F. C., Mattera, C. G., Kjærgaard, M. B., Veje, C., and Jørgensen, B. N. (2017). A world class energy efficient university building by Danish 2020 standards. Energy Procedia 132, 21–26. doi:10.1016/j.egypro.2017.09.625
Kalkan, N., Bercin, K., Cangul, O., Morales, M. G., Saleem, M. M. K. M., Marji, I., et al. (2011). A renewable energy solution for Highfield campus of university of Southampton. Renew. Sustain. Energy Rev. 15 (6), 2940–2959. doi:10.1016/j.rser.2011.02.040
Khoshbakht, M., Gou, Z., and Dupre, K. (2018). Energy use characteristics and benchmarking for higher education buildings. Energy Build. 164, 61–76. doi:10.1016/j.enbuild.2018.01.001
Kourgiozou, V., Commin, A., Dowson, M., Rovas, D., and Mumovic, D. (2021). Scalable pathways to net zero carbon in the UK higher education sector: a systematic review of smart energy systems in university campuses. Renew. Sustain. Energy Rev. 147, 111234. doi:10.1016/j.rser.2021.111234
Lauder, A., Sari, R. F., Suwartha, N., and Tjahjono, G. (2015). Critical review of a global campus sustainability ranking: greenMetric. J. Clean. Prod. 108, 852–863. doi:10.1016/j.jclepro.2015.02.080
Leal Filho, W., Salvia, A. L., Paço, A. d., Anholon, R., Gonçalves Quelhas, O. L., Rampasso, I. S., et al. (2019). A comparative study of approaches towards energy efficiency and renewable energy use at higher education institutions. J. Clean. Prod. 237, 117728. doi:10.1016/j.jclepro.2019.117728
Leon, I., Oregi, X., and Marieta, C. (2018). Environmental assessment of four Basque University campuses using the NEST tool. Sustain. Cities Soc. 42, 396–406. doi:10.1016/j.scs.2018.08.007
Levy, J. M., Ohadi, M. M., and Kyosung, C. (2015). Energy analysis of cleanrooms in an academic research building. ASHRAE Trans. 121, 71+.
Li, K., Sun, Y., Robinson, D., Ma, J., and Ma, Z. (2020). A new strategy to benchmark and evaluate building electricity usage using multiple data mining technologies. Sustain. Energy Technol. Assessments 40, 100770. doi:10.1016/j.seta.2020.100770
Li, S., and Chen, Y. (2021). Internal benchmarking of higher education buildings using the floor-area percentages of different space usages. Energy Build. 231, 110574. doi:10.1016/j.enbuild.2020.110574
Li, Y., and Jia, Q.-S. (2022). On the feasibility to achieve carbon neutrality in university campus: a case study. IFAC-PapersOnLine 55 (5), 78–83. doi:10.1016/j.ifacol.2022.07.643
Ligade, J., and Razban, A. (2019). Investigation of energy efficient retrofit HVAC systems for a university: case study. Sustainability 11 (20), 5593. doi:10.3390/su11205593
Litardo, J., Hidalgo-Leon, R., and Soriano, G. (2021). Energy performance and benchmarking for university classrooms in hot and humid climates. Energies 14 (21), 7013. doi:10.3390/en14217013
Liu, Q., and Ren, J. (2020). Research on the building energy efficiency design strategy of Chinese universities based on green performance analysis. Energy Build. 224, 110242. doi:10.1016/j.enbuild.2020.110242
Ma, Y. T., Lu, M. Y., and Weng, J. T. (2015). “Energy consumption status and characteristics analysis of university campus buildings,” in Proceedings of the 5th International Conference on Civil Engineering and Transportation, Guangzhou, China, January 2015.
Mahyuddin, N., Awbi, H. B., and Alshitawi, M. (2013). The spatial distribution of carbon dioxide in rooms with particular application to classrooms. Indoor Built Environ. 23 (3), 433–448. doi:10.1177/1420326x13512142
Makino, S., Onishi, T., Itoh, A., Sato, I., Huzita, T., and Kayo, C. (2021). Sustainable campus: reducing environmental and financial burdens by using pruned branches for on-campus energy. Sustainability 13, 7480. doi:10.3390/su13137480
Mohamed, N. H., Noor, Z. Z., and Sing, C. L. I. (2020). “Environmental sustainability of universities: critical review of best initiatives and operational practices,” in Green engineering for campus sustainability. Editor A. Z. Yaser (Singapore: Springer Singapore), 5–17.
Mohamed, O., Fakhoury, S., Aldalou, G., and Almasri, G. (2022). Energy auditing and conservation for educational buildings: a case study on princess Sumaya university for technology. Process Integration Optim. Sustain. 6 (4), 901–920. doi:10.1007/s41660-022-00273-z
Mohammadalizadehkorde, M., and Weaver, R. (2018). Universities as models of sustainable energy-consuming communities? Review of selected literature. Sustainability 10, 3250. doi:10.3390/su10093250
Mohd Shukri, M. A., Jailani, J., and Hauashdh, A. (2022). Benchmarking the energy efficiency of higher educational buildings: a case study approach. Int. J. Energy Econ. Policy 12 (2), 491–496. doi:10.32479/ijeep.11941
Mokhtara, C., Negrou, B., Settou, N., Bouferrouk, A., and Yao, Y. (2021). Design optimization of grid-connected PV-Hydrogen for energy prosumers considering sector-coupling paradigm: case study of a university building in Algeria. Int. J. Hydrogen Energy 46 (75), 37564–37582. doi:10.1016/j.ijhydene.2020.10.069
MSPI (2022). More than 4 million riyals the rate of waste of electricity at the university annually!. Available at: https://rs.ksu.edu.sa/issue-1365/16391 (Accessed June 29, 2022).
Muqeet, H. A., Munir, H. M., Javed, H., Shahzad, M., Jamil, M., and Guerrero, J. M. (2021). An energy management system of campus microgrids: state-of-the-art and future challenges. Energies 14, 6525. doi:10.3390/en14206525
Mytafides, C. K., Dimoudi, A., and Zoras, S. (2017). Transformation of a university building into a zero energy building in Mediterranean climate. Energy Build. 155, 98–114. doi:10.1016/j.enbuild.2017.07.083
Nasir, S. N. S., Ludin, N. A., Radzi, A. A. S. M., Junedi, M. M., Ramli, N., Marsan, A., et al. (2022). Lockdown impact on energy consumption in university building. Environ. Dev. Sustain 25, 12051–12070. doi:10.1007/s10668-022-02507-z
Niemelä, T., Kosonen, R., and Jokisalo, J. (2016). Cost-optimal energy performance renovation measures of educational buildings in cold climate. Appl. Energy 183, 1005–1020. doi:10.1016/j.apenergy.2016.09.044
Ntsaluba, S. B. K., and Mukadi, P. (2019). “Comparative campus energy usage study for a South African university,” in Proceedings of the 2019 International Conference on the Domestic Use of Energy (DUE), Wellington, South Africa, March 2019.
Olivieri, L., Caamaño-Martín, E., Sassenou, L. N., and Olivieri, F. (2020). Contribution of photovoltaic distributed generation to the transition towards an emission-free supply to university campus: technical, economic feasibility and carbon emission reduction at the Universidad Politécnica de Madrid. Renew. Energy 162, 1703–1714. doi:10.1016/j.renene.2020.09.120
Opel, O., Strodel, N., Werner, K., Geffken, J., Tribel, A., and Ruck, W. (2017). Climate-neutral and sustainable campus Leuphana university of Lueneburg. Energy 141, 2628–2639. doi:10.1016/j.energy.2017.08.039
Quevedo, T. C., Geraldi, M., Melo, A., and Lamberts, R. (2024). Benchmarking energy consumption in universities: a review. J. Build. Eng. 82, 108185. doi:10.1016/j.jobe.2023.108185
Quevedo, T. C., Geraldi, M. S., and Melo, A. P. (2023). Applying machine learning to develop energy benchmarking for university buildings in Brazil. J. Build. Eng. 63, 105468. doi:10.1016/j.jobe.2022.105468
Renedo, C. J., Ortiz, A., Pérez, S., Delgado, F., Fernández, I., and Carcedo, J. (2014). Improving the efficiency of an air conditioning system using a fire water tank as thermal accumulator. Build. Serv. Eng. Res. Technol. 36 (3), 386–405. doi:10.1177/0143624414555812
Rey-Hernández, J. M., Rey-Martínez, F. J., Yousif, C., and Krawczyk, D. (2023). Assessing the performance of a renewable District Heating System to achieve nearly zero-energy status in renovated university campuses: a case study for Spain. Energy Convers. Manag. 292, 117439. doi:10.1016/j.enconman.2023.117439
Saeed, S. A. R. (1993). Thermal comfort requirements in hot dry regions with special reference to Riyadh, Part 1: for university students. Int. J. Ambient Energy 14 (3), 147–154. doi:10.1080/01430750.1993.9675608
Sait, H. H. (2013). Auditing and analysis of energy consumption of an educational building in hot and humid area. Energy Convers. Manag. 66, 143–152. doi:10.1016/j.enconman.2012.10.005
Samira, A., and Nurmammad, M. (2018). Multi-disciplinary energy auditing of educational buildings in Azerbaijan: case study at a university campus. IFAC-PapersOnLine 51 (30), 311–315. doi:10.1016/j.ifacol.2018.11.308
Saudi Electric Company (2021). Annual statistical booklet for electricity and seawater desalination industries. Available at: https://www.ecra.gov.sa/en-us/MediaCenter/doclib2/Pages/SubCategoryList.aspx?categoryID=5.
Şevik, S. (2022). Techno-economic evaluation of a grid-connected PV-trigeneration-hydrogen production hybrid system on a university campus. Int. J. Hydrogen Energy 47 (57), 23935–23956. doi:10.1016/j.ijhydene.2022.05.193
Soares, N., Dias Pereira, L., Ferreira, J., Conceição, P., and Pereira da Silva, P. (2015). Energy efficiency of higher education buildings: a case study. Int. J. Sustain. High. Educ. 16 (5), 669–691. doi:10.1108/ijshe-11-2013-0147
Soliman, A. M. A., and Mehanna, M. A. (2023). Sustainable and green academic buildings in Al-azhar university: case study. Int. J. Renew. Energy Res. 13 (1), 87–103. doi:10.20508/ijrer.v13i1.13431.g8664
Stavropoulos, T. G., Koutitas, G., Vrakas, D., Kontopoulos, E., and Vlahavas, I. (2016). A smart university platform for building energy monitoring and savings. J. Ambient Intell. Smart Environ. 8 (3), 301–323. doi:10.3233/ais-160375
Sufian Hasim, M., Wan Azam, W. F. H., Hashim, A. E., and Muhamad Ariff, N. R. (2019). The implementation of sustainable energy initiatives in campus buildings. Asian J. Qual. Life 4 (17), 63–77. doi:10.21834/ajqol.v4i17.201
Sun, Y., Luo, X., and Liu, X. (2021). Optimization of a university timetable considering building energy efficiency: an approach based on the building controls virtual test bed platform using a genetic algorithm. J. Build. Eng. 35, 102095. doi:10.1016/j.jobe.2020.102095
Tazay, A. (2021). Techno-economic feasibility analysis of a hybrid renewable energy supply options for university buildings in Saudi Arabia. Open Eng. 11 (1), 39–55. doi:10.1515/eng-2021-0005
Tian, X., Zhou, Y., Morris, B., and You, F. (2022). Sustainable design of Cornell University campus energy systems toward climate neutrality and 100% renewables. Renew. Sustain. Energy Rev. 161, 112383. doi:10.1016/j.rser.2022.112383
University of Central Florida (2021). Open energy information system. Available at: https://oeis.ucf.edu/(Accessed April 11, 2023).
Wiryadinata, S., Morejohn, J., and Kornbluth, K. (2019). Pathways to carbon neutral energy systems at the University of California, Davis. Renew. Energy 130, 853–866. doi:10.1016/j.renene.2018.06.100
Worldwide (2022). Degree days calculated accurately for locations worldwide. Available at: https://www.degreedays.net/(Accessed September 12, 2023).
Yıldırım, N., Toksoy, M., and Gökçen, G. (2006). District heating system design for a university campus. Energy Build. 38 (9), 1111–1119. doi:10.1016/j.enbuild.2006.01.004
Yildirim, N., Toksoy, M., and Gokcen, G. (2010). Piping network design of geothermal district heating systems: case study for a university campus. Energy 35 (8), 3256–3262. doi:10.1016/j.energy.2010.04.009
Yildiz, Y., and Kocyigit, M. (2021). Energy consumption analysis of education buildings: the case study of balikesir university. Gazi Univ. J. Sci. 34 (3), 665–677. doi:10.35378/gujs.722746
Keywords: education facilities, energy efficiency, photovoltaics, renewable energy, sustainability
Citation: Almasri RA, Abu-Hamdeh NH and Al-Tamimi N (2024) A state-of-the-art review of energy-efficient and renewable energy systems in higher education facilities. Front. Energy Res. 11:1344216. doi: 10.3389/fenrg.2023.1344216
Received: 25 November 2023; Accepted: 27 December 2023;
Published: 23 January 2024.
Edited by:
Hegazy Rezk, Prince Sattam Bin Abdulaziz University, Saudi ArabiaReviewed by:
Elisa Marrasso, University of Sannio, ItalyCopyright © 2024 Almasri, Abu-Hamdeh and Al-Tamimi. This is an open-access article distributed under the terms of the Creative Commons Attribution License (CC BY). The use, distribution or reproduction in other forums is permitted, provided the original author(s) and the copyright owner(s) are credited and that the original publication in this journal is cited, in accordance with accepted academic practice. No use, distribution or reproduction is permitted which does not comply with these terms.
*Correspondence: Radwan A. Almasri, cmEuYWxtYXNyaUBxdS5lZHUuc2E=, bWFzcmkucmFkd2FuQHFlYy5lZHUuc2E=, bWFzcmkucmFkd2FuQGdtYWlsLmNvbQ==
Disclaimer: All claims expressed in this article are solely those of the authors and do not necessarily represent those of their affiliated organizations, or those of the publisher, the editors and the reviewers. Any product that may be evaluated in this article or claim that may be made by its manufacturer is not guaranteed or endorsed by the publisher.
Research integrity at Frontiers
Learn more about the work of our research integrity team to safeguard the quality of each article we publish.