- 1Department of Civil, Architectural and Environmental Engineering, Department of Civil, Architectural and Environmental Engineering, University of Padova, Padova, Italy
- 2Department of Environmental Science and Policy, University of Milan, Milano, Italy
- 3Department of Bioengineering and Imperial College Centre for Synthetic Biology, Imperial College London, London, United Kingdom
Introduction: Compost heat recovery systems (CHRS) represent an emerging technology to recover residual woody biomass from agroforestry and forestry activities and use the heat that is naturally produced during aerobic biodegradation (composting). However, a low oxygen concentration in the gas phase and self-drying and compaction of the compost body often limit efficient oxidation by microbial communities. Woodchip-derived biochar has often been proposed as a bulking agent and improver of water retention and of oxygen accessibility in the composting process, but the literature reporting its effects in the CHRS is scarce.
Methods: Here, biochar (average particle size of 10 mm) was added at 10% (on weight basis) to chipped pruning residues into two bench-scale-controlled reactors (0.2 m3), operated in parallel for 57 days.
Results and Discussion: The addition of 10% (w/w) biochar to the composting body increased biodegradation yields by approximately 50% and improved oxidation rates over readily biodegradable organic fractions (addition of cheese whey). Temperatures were on average 1.34°C higher, and heat extraction flux was also improved in the presence of biochar (0.3 kW/m3) versus in its absence (0.1 kW/m3). The organic matter mass balance resulted in approximately 50% higher biodegradation yield and improved oxidation rates over readily biodegradable organic fractions. Microbial analysis highlighted a higher concentration of thermophilic species and a lower concentration of well-known pathogenic and antibiotic-resistant genera in the presence of biochar.
1 Introduction
Although energy recovery from organic waste is one of the pillars of circular economy, waste-to-energy approaches not only protect human health and the environment but can also contribute significantly to the efficient substitution of fossil fuel resources (Zhou and Zhang, 2022). Residual biomass from agriculture and forestry activities can be converted into usable forms of energy (thermal energy, electricity, or biofuels) (Di Fraia et al., 2020) through various biorefinery processes. Compost heat recovery systems (CHRSs) can generate heat for buildings and households by recovering the heat naturally produced during aerobic composting of organic matter (Malesani et al., 2021). Composting is the aerobic biodegradation of organic materials, where heat is released during microbial respiration (Zhao et al., 2017).
In regular composting facilities, the heat generated from composting is typically dispersed into the environment as the preliminary goal of composting is the safe treatment of organic residues and the production of soil amendment. However, with the rapid increase in the worldwide energy demand, the generated heat is gaining increasing interest as it can be a sustainable alternative to fossil fuels, thereby reducing global warming (Fan et al., 2021). According to Smith and Aber (2017), CHRSs can replace significant amounts of fossil fuels commonly used for domestic heating, thereby proving their commercial viability.
However, CHRSs still face some limitations, especially in real-scale implementation. The thermal power generated per unit of the volume of an organic substance remains relatively low, primarily due to inefficiencies in the three key steps of the process: a) limited rate of microbial hydrolysis of the organic matter; b) limited oxygen mass transfer through the biomass body; and c) inefficient heat exchange between the biomass and the heat transfer system. These mechanisms are influenced by key variables such as bulk density and biomass porosity, relative moisture content, and the air–water–solid volume ratios. Limitations to capillary air flow through the bulk biomass body result in limiting both oxygen supply to microbial communities and efficient heat transfer. Simultaneously, moisture water retention within the biomass macroaggregates plays a role in facilitating microbial hydrolysis and cation transfer in water solutes, along with the microbial oxidation of organic compounds.
In the present study, biochar was chosen as the bulking agent and improver of the composting processes. Biochars are carbon-rich materials produced by biomass pyrolysis (Lehmann and Joseph, 2015). Biochars have several chemical, physical, and electrochemical properties, such as high macro- and micro-porosity, high ion-exchange capacity, water sorption and holding capacity, thermal conductivity, surface electrochemical properties, and some electrical conductivity (Yang et al., 2017; Schievano et al., 2019; Bona et al., 2020). Thanks to these properties, numerous studies have demonstrated that biochar addition can enhance composting processes. Biochar has been tested as an amendment in composting processes because it is expected to have several benefits, such as decreased bulk density, increased aeration, improved microbial respiration rates, and enhanced water holding capacity (Wang et al., 2021). The addition of biochar improves the composting process, as evidenced by an increase in temperature during the thermophilic phase and a shortened lag-period before the thermophilic phase (Chen et al., 2010). Wang et al. (2021) reported that biochar addition at 5%, 10%, and 15% resulted in prolonged periods of temperatures over 67°C. Waqas et al. (2018) reported that biochar addition (15%) allows for the rapid achievement of thermophilic conditions. While numerous literature reviews have been conducted to address composting processes, certain aspects, particularly those related to heat recovery and utilization, are inadequately covered in current reviews (Fan et al., 2021). Nowadays, one of the most crucial challenges for the CHRS is to enhance volumetric heat extraction rates to achieve a cost per unit of energy that is comparable to or lower than competitive solutions in decentralized areas, such as solar panels and pellet combustors.
The primary objective of the present experimental research was to assess the impact of adding biochar to the compost pile in terms of (1) biodegradation rates and mass balance and (2) temperatures and volumetric heat extraction rates (in kW/m3). We used pilot-scale CHRS units to better control heat transfer and losses, as well as process parameters.
2 Materials and methods
2.1 Experimental plan
The experimental process was carried out in two bench-scale reactors (Figure 1) operated in parallel for 57 days, where boundary conditions were kept identical (ambient temperature, water refill, and amount of external feeding). Reactor 1, representing the control, contained only woodchip, and Reactor 2 contained woodchip +10% (on weight basis) biochar. To ensure homogeneous distribution through the whole reactor body, the solution was added both from the top and the middle of the reactors using rigid tubes. All feedstock used for each reactor and their characteristics are given in Tables 1, 2. A liquid inoculum was added at 2% (V/V) of the reactor volume, as indicated in previous experiments (Fan et al., 2018). The reactors worked in a semi-closed environment under well-controlled boundary conditions. According to the initial moisture content of the materials involved (measured as the weight loss after heating at 95°C until weight variations were negligible), an appropriate amount of water was periodically added to both reactors in order to restore 60% MC, which is an ideal moisture content for composting processes, according to the literature (Azim et al., 2018; Fan et al., 2021). Each reactor was equipped with a hydraulic circuit for heat extraction and quantification of heat recovery (see Supplementary Material).
2.2 Water-soluble carbon-source feeding
Along the experiment, tests were performed to verify the differential capacity of the two systems to access oxygen as the electron acceptor of microbial respiration. Injections of a readily biodegradable carbon source were provided by the manual addition of liquid cheese whey twice a week, on average. Details of the composition of the liquid solution are given in Table 2.
2.3 Heat recovery
The measurements and calculation of heat extraction were done after day 22 (35 days), during which three different amounts of external feeding were tested. To extract the heat, a pump was manually switched on and off. The thermal power extracted (kW) was calculated using Eq. 1:
where
2.4 Process monitoring and characterization
During the 57 days, the following parameters were monitored:
- Temperature inside and outside the reactors was monitored one time per minute during the whole period through the remote monitoring system including two probes placed inside the reactors’ body (one placed at a 0.4-m depth and one at a 1.2-m depth) plus one probe for the external ambient temperature for each reactor.
- Volatile solid (VS), total solid (TS), moisture content (MC), and pH were alternately monitored every 5 days during the first 22 days through the manual collection of samples from the reactors’ body of approximately 15 g. Then, they were analyzed in the middle and at the end of the 57 days.
- The total organic carbon (TOC) was monitored at the beginning, middle, and end of the experiment.
- The lower heating value (LHV) was measured on the raw biomass (at the beginning of the tests, before filling the reactors) and on the treated biomass (at the end of the 57 days of the experiment). O2, CO2, and CH4 percentages (%V/V) were monitored two times per week using an OPTIMA 7 BIOGAS gas analyzer model by inserting the gas collection pipe directly into the specific valves placed on the columns (one at 0.66 m and the other one at a 1.24-m depth) during the first 22 days of monitoring.
Shotgun metagenomic sequencing was performed (Novogene, United Kingdom) in order to comprehensively define the abundant organisms present in samples taken from reactors 1 and 2 at the end of the experiment and to correlate the reactor performance with the composition of microbial communities. A DNA library was prepared by fragmentation, end repair, and A-tailing, followed by adapter ligation, PCR amplification, size selection, and purification. The sequencing depth was ≥40 million read pairs per sample. The library was checked using Qubit and real-time PCR, and quantified libraries in terms of concentration and data amount were sequenced on an Illumina NovaSeq 6000 platform. Table 2 illustrates the data on the initial physical–chemical characterization of input feedstocks as raw (not already mixed) and in mixtures (to be placed in Reactor 2) before being added to the reactors.
2.5 Data analysis
The experiments in the present study were not run in duplicates. Therefore, a single-trial experiment did not allow proper statistical analysis on replicates to support the robust significance of the observed differences between the treatment and the control condition. However, for the monitored parameters, variance was calculated either as the standard deviation or represented by quartiles (in box plots) by repeated measurements along time and/or sampling in different places of the reactors (top, middle, and bottom) (see Supplementary Material). Analysis of variance (ANOVA) was performed to detect significant differences between repeated measurements (in time and space) in the treatment and the control reactors.
3 Results
3.1 Carbon and energy balance
Ash conservation was applied as the principle for calculating the balance, and detailed calculations on 1-kg TS are given in Supplementary Table S2. Table 3 shows the complete mass balances for reactors 1 and 2 using the VS, TOC, and LHV as parameters. Reactor 1 resulted in 52%, 56%, and 49% biodegradation yields for VS, TOC, and LHV, respectively, while for Reactor 2, the yields were 78%, 82%, and 81%, respectively. The presence of biochar in Reactor 2 induced an increase in biodegradation yields by 50%, 47%, and 67%, respectively, for the three parameters.
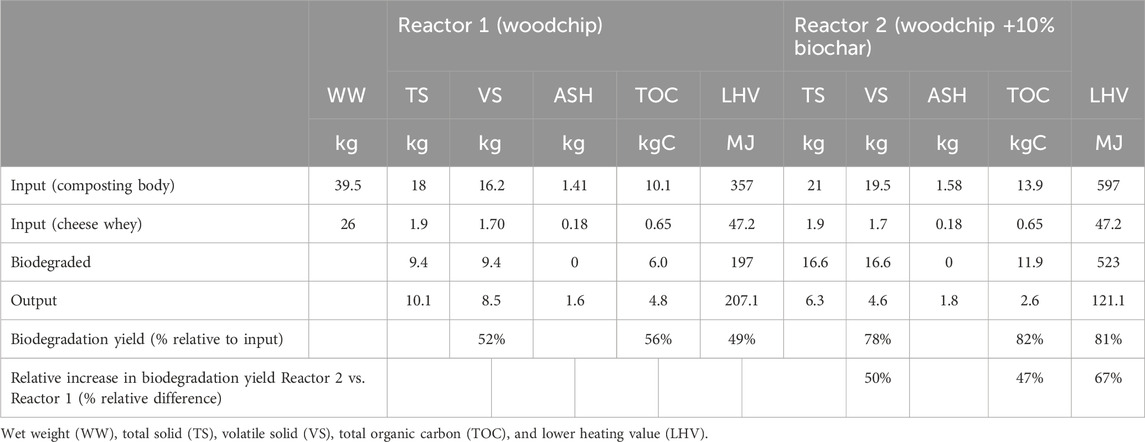
TABLE 3. Mass balance of the biodegradation processes (as VS, TOC, and LHV) as measured in reactors 1 and 2 for 57 days of operation. Biodegradation yield is calculated as the ratio between biodegraded mass and input mass. The relative increase in the biodegradation yield obtained by the addition of biochar (10% w/w) in Reactor 2, as compared to Reactor 1, is calculated as the percentage relative difference: (R2–R1)/R1.
In parallel, there was also a significant difference between reactors 1 and 2 in the (MC along the process (before periodical water re-fill). Supplementary Table S3 shows how MC, despite identical treatment for 53 days, was on average 19% higher (p < 0.05, n = 5) in Reactor 2, confirming that biochar has an effect in preventing over-drying of the material despite the higher temperatures inside the Reactor 2 compared to Reactor 1.
3.2 Heat extraction
Complete energy recovery was not possible due to uncontrolled heat dispersion by the bench-scale reactors. However, the heat exchangers allowed measuring the heat flux during definite observation periods of 1 or few hours. The monitored heat fluxes are given in Table 4. The calculations were performed, according to Eq. 3, using a flowmeter installed in the hydraulic circuit (see Supplementary Material). The higher difference in temperatures outgoing and re-entering the bodies of Reactor 2 is related to the higher temperature reached inside Reactor 2 due to a better degradation efficiency, resulting in higher thermal power outputs for Reactor 2 due to a higher temperature maintained for longer periods.
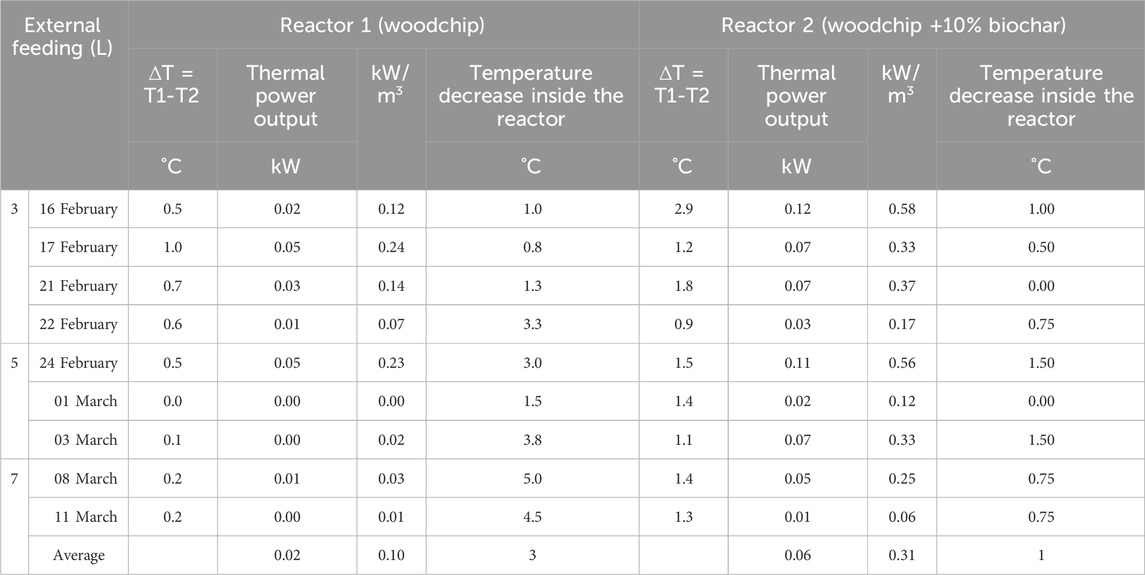
TABLE 4. Temperatures inside the hydraulic circuit during the heat extraction phases, calculated thermal power outputs, and temperature decrease inside the reactors during heat extraction.
Furthermore, the temperatures of the material placed inside the reactors were monitored during heat extraction, and the drops (between the beginning and the end of the heat extraction) were monitored to avoid the excessive cooling of the feedstocks inside the reactor, which could result in the shutdown of the biological process. On average, as shown in Table 4, the heat extraction phase lasted longer for Reactor 2 than for Reactor 1. During each heat extraction process, the temperature inside Reactor 1 decreased too much after 25 min on average (3°C on average), while in Reactor 2, the highest temperature lasted for 42 min on average and then decreased by approximately 1°C.
The thermal power output of the traditional version (Reactor 1) emerged to be 0.10 kW/m3, which was in line with the average literature data reported by Malesani et al. (2021), while for the innovative version (Reactor 2), it was 0.31 kW/m3 significantly higher than that reported in the literature data, confirming the beneficial effect of biochar addition.
3.3 Temperatures within the composting body
The average temperatures inside the reactors’ bodies suggest beneficial effects of biochar addition, as shown in Figure 2A. The ambient temperature was maintained between 25 and 35° for the whole period and monitored by the two probes placed close to the reactors. The ambient temperature probes, together with those placed inside the reactors, recorded the data every minute during the whole period and sent the data to an online graphical interface. The data were then processed (24 data points per day for the probes placed inside the reactors and 6 data points for the probes monitoring the external ambient temperature). In Figure 2A, the red curve representing the temperatures inside Reactor 2 (containing woodchip +10% biochar) remained higher for the whole period compared to the green line representing the temperatures inside Reactor 1.
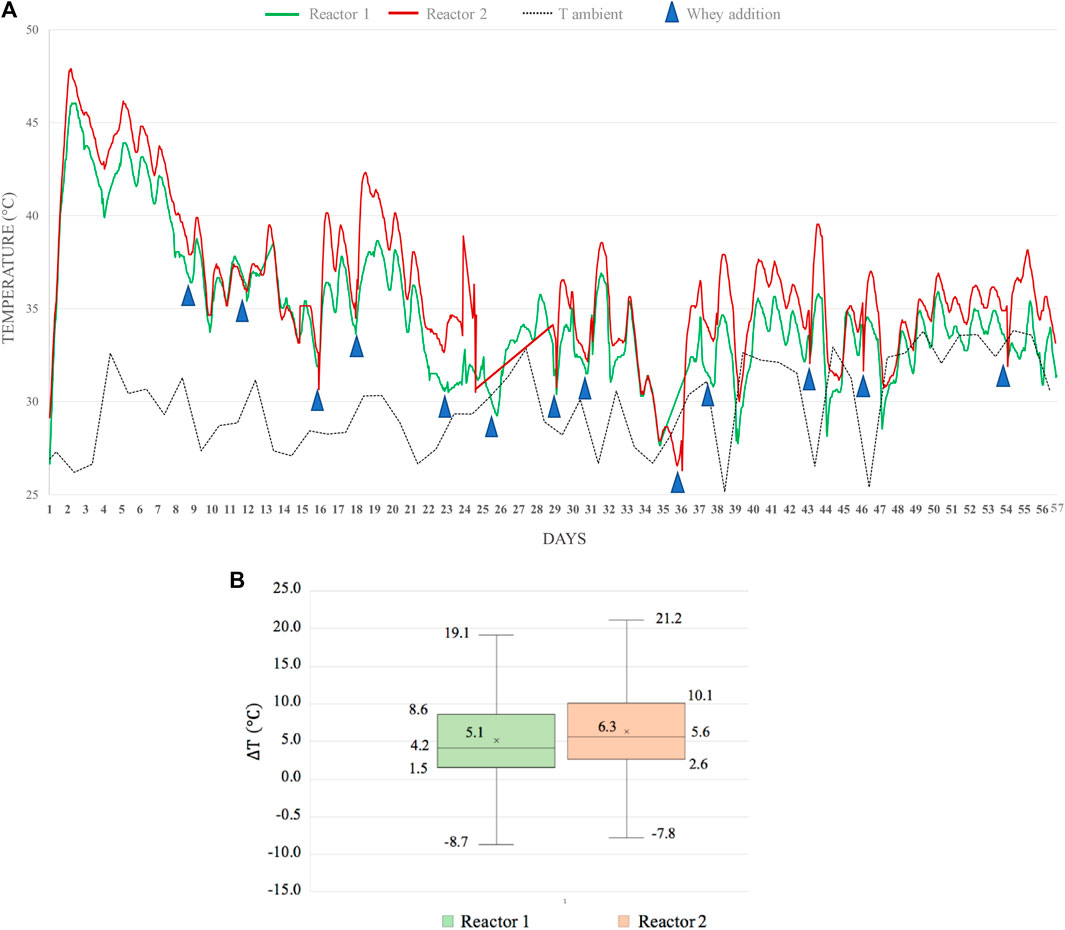
FIGURE 2. (A) Comparison of temperatures trend inside the two reactors and ambient temperature. Curves represent the average values of 24 data points per day collected at two depths for Reactor 1 (woodchip) and Reactor 2 (woodchip + 10% biochar). Blue triangles represent the external feeding addition of whey. (B) Boxplot graph showing the distribution of the hourly-average differences of temperatures (ΔT) between inside and outside Reactor 1 (woodchip) and Reactor 2 (woodchip + 10% biochar). The line within the box shows the median value, the box denotes the range of 50% of data, X stands for the average value.
Moreover, data analysis was performed on the temperature delta that represents the difference between the temperature inside each reactor and the ambient temperature. The distribution of the delta temperature values is shown in Figure 2B. The average difference for Reactor 2 was higher than that for Reactor 1. Negative values are related to the fact that sometimes the ambient temperature increased until 35° because of weather, and it happened that the temperature inside the reactors was lower than the ambient temperature, resulting in negative delta values. On average, Reactor 2 was 1.34°C warmer than Reactor 1, as shown in Figure 2B.
3.4 Composition of the gas phase
Moreover, CO2, O2, and CH4 concentrations in the headspace were monitored at two different depths for the two reactors, and their distributions are shown as box plots (Figure 3). Since methane has always remained under the detection limit of the instrument (<0.1% v/v), no results regarding CH4 are reported here. As it can be observed, O2 tended to be higher for Reactor 2 (18.3% (V/V) on average, ranging from 17.5% to 19.0%), with respect to Reactor 1 (17.4% (V/V) as an average value between 16.1% and 19.0%). In accordance, CO2 tended to be higher for Reactor 1 (2.1% (V/V) as an average value between 0.7% and 3.4%) than for Reactor 2 (1.8% (V/V) as an average value between 0.8% and 2.8%).
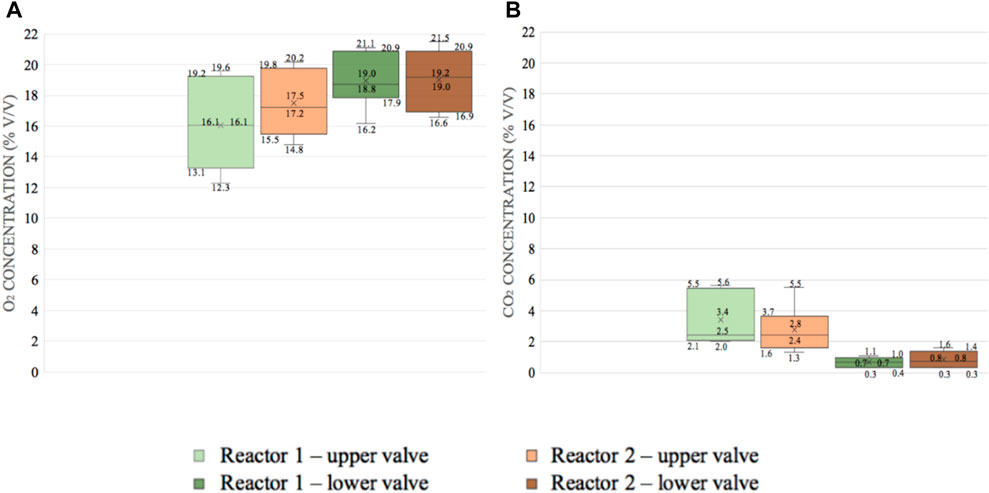
FIGURE 3. (A) Box plot of the O2 values monitored for Reactor 1 (woodchip) and Reactor 2 (woodchip +10% biochar). The line within the box shows the median value, the box denotes the range of 50% of data, and X stands for the average value. (B) Box plot of the CO2 values in % monitored for Reactor 1 (woodchip) and Reactor 2 (woodchip +10% biochar). The line within the box shows the median value, the box denotes the range of 50% of data, and X stands for the average value.
3.5 Response to shock tests using readily biodegradable carbon
External feeding was performed twice a week during the whole experimental period. The volume of whey added increased from 1.5 L to 7 L during the whole period, as shown in Table 4. On average, the amount of whey added was increased after 1 week, except for a 3-L addition that lasted 2 weeks. It was interesting to evaluate a possible correlation with an increase in the total organic carbon content (due to an increase in the whey volume addition) and the temperature peaks. The average temperature peaks monitored were 44.8°C for Reactor 1 and 45.1°C for Reactor 2, with no evidence in better performances with the increase in whey addition.
The TOC content of whey was monitored after collection from the dairy farms (Cadoneghe and Piove di Sacco), and it has always remained constant, at approximately 25,000 ± 299.9 mg C/L. Table 5 shows the temperatures inside the reactors before and after whey addition, the difference in temperatures before whey addition and after whey addition when the temperature peak was reached, and the time needed for each reactor to reach the temperature peaks.
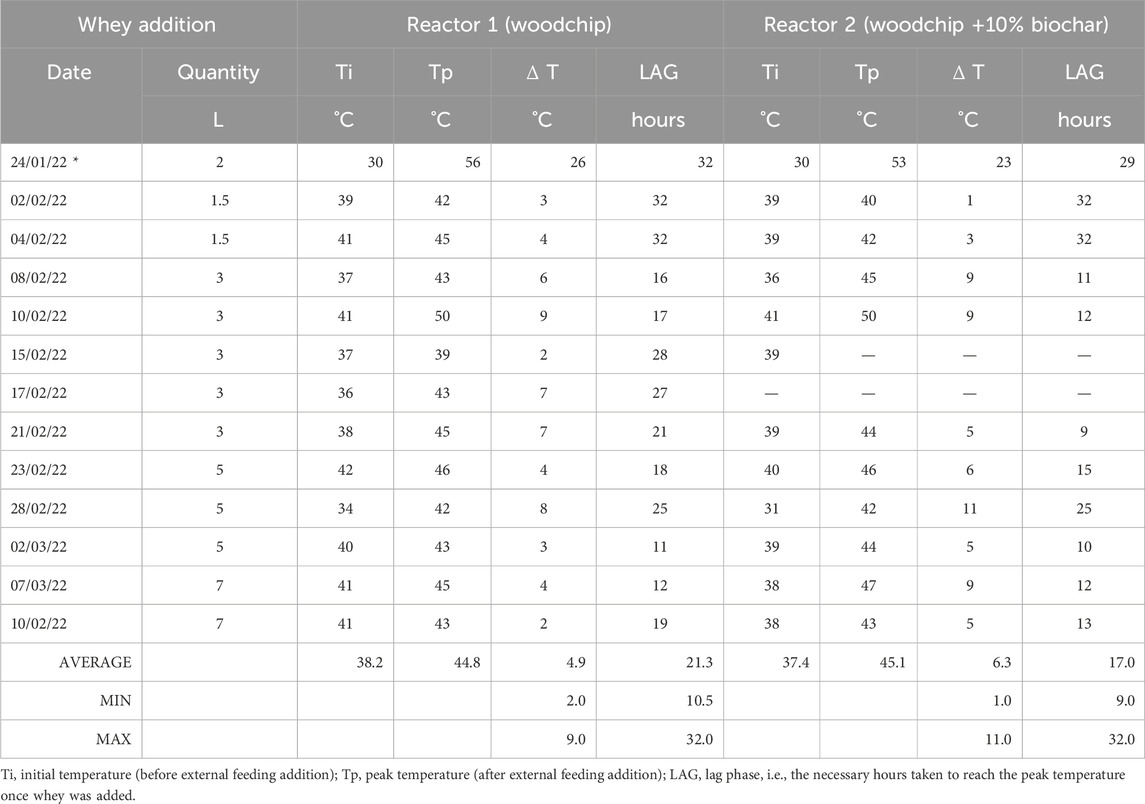
TABLE 5. Temperatures and lag phases monitored inside reactors 1 and 2 before and after external feeding addition with whey.
As shown in Table 4, the difference in temperature
3.6 Microbial community analysis
Figure 4 shows the microbial community analysis results at phylum, class, and genus levels in Reactor 1 (woodchip) and Reactor 2 (woodchip +10% biochar).
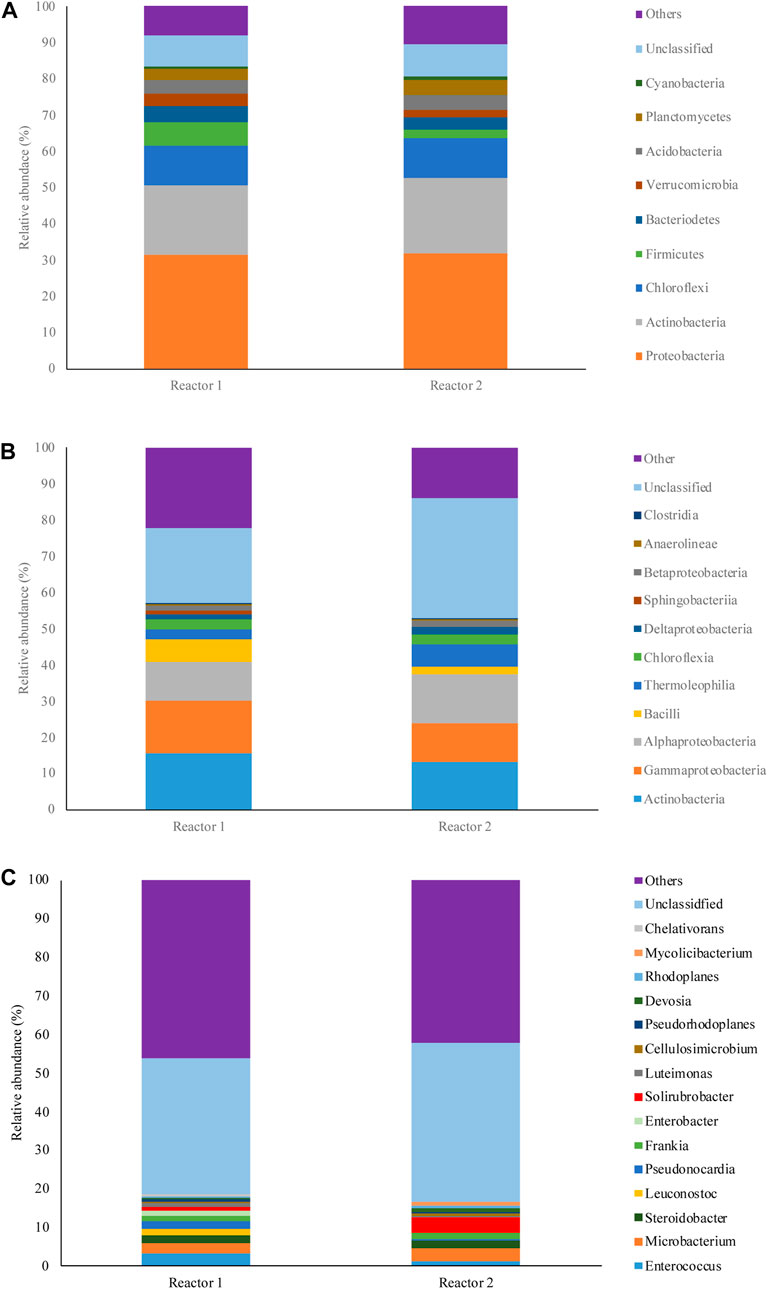
FIGURE 4. Composition of microbial communities in the reactors at (A) phylum, (B) class, and (C) genus levels.
In both reactors, approximately 60% of the community at the phylum level was dominated by three phyla, Proteobacteria, Actinobacteria, and Chloroflexi. The abundance of two other phyla, Firmicutes and Verrucomicrobia, in Reactor 1 was 64% and 41% higher than in Reactor 2. These phyla are commonly found in the literature as the most abundant species in organic materials that underwent composting processes (G. Wang et al., 2022a; S; Wang et al., 2022b).
At the class level, both reactors 1 and 2 were dominated by Actinobacteria (15.62% and 13.19%), Gammaproteobacteria (14.35% and 10.71%), and Alphaproteobacteria (10.71% and 13.57%), respectively. Actinobacteria are important compost microbes; they possess a wide array of enzymes that are involved in delignification, and they increase through the compost process (Kirby, 2005; Bhatti et al., 2017; Duran et al., 2022). Furthermore, Gammaproteobacteria are known as lignin degraders (Bugg et al., 2020), and Alphaproteobacteria are related to delignification processes (Duran et al., 2022). The relative abundance of Bacilli in Reactor 1 (6.2%) was almost three times of that in Reactor 2 (1.97%). The higher relative abundance of Thermoleophilia in Reactor 2 (6.34%) compared to Reactor 1 (2.91%) could be correlated with the higher temperatures obtained in Reactor 2. Species belonging to Thermoleophilia are known as heat lovers (Hu et al., 2019). Other classes including Chloroflexia, Deltaproteobacteria, Betaproteobacteria, Anaerolineae, and Clostridia had similar abundances in both reactors.
Genus-level analysis indicated that microbial communities in both reactors 1 and 2 were very diverse, and no genera accounted for more than 4% of the community in the reactors, while a high number of taxa was present at very low abundance (<0.01%). Due to the high diversity, only genera with abundances higher than 0.5% are shown in Figure 4. The other genus contributions to diversity that were <0.5% are defined as Other. The variation in microbial communities between the two reactors was more obvious at the genus level as some genera experienced abundances up to 12-fold higher than that of the other reactor. In Reactor 1, Enterococcus (a large genus of lactic acid bacteria) was the dominant genus at the end of the experiment, with 3.43% abundance. The other abundant genera in Reactor 1 comprised Microbacterium (2.54%), Steroidobacter (2.10%), Leuconostoc (1.69%), Pseudonocardia (1.89%), Frankia (1.48%), and Enterobacter (1.19%). Reactor 2 was dominated by Solirubrobacter (3.93%), a genus belonging to the class Thermoleophilia. Microbacterium (3.32%), Steroidobacter (1.9%), Frankia (1.37%), and Enterococcus (1.32%) were the other abundant genera defined in Reactor 2.
Interestingly, our data showed that in Reactor 2, which contained 10% biochar, the abundance of some well-known pathogenic and antibiotic-resistant genera were lower than that in Reactor 1 at the end of the experiment. Leuconostoc, which is known to be resistant to several antibiotics (e.g., streptomycin, vancomycin and teicoplanin, trimethoprim, sulfamethoxazole, cefuroxime, tetracycline, and clindamycin (Casado Muñoz et al., 2014; Jeong and Lee, 2015; Kumar et al., 2022), contributed to 0.06% of the community in Reactor 2, which was equal to a 12-fold decrease in relative abundance compared to Reactor 1. Similarly, lower abundances of 3.96-, 2.65-, and 2.59-fold were observed for Enterobacter, Luteimonas, and Enterococcus, respectively. The observed decrease in antibiotic resistance and pathogenic genera has a positive impact on the stability and functionality of local ecological communities. This is achieved through the reduction of potential risks associated with the spread of antibiotic-resistance genes and harmful pathogens, and it is essential for both the ecosystem and public health (Ohore et al., 2022; Bagra et al., 2023). The promising results highlight the practical application of our proposed technology as an ecofriendly process that integrates waste management and heat recovery while promoting a healthier and more balanced ecosystem.
4 Discussion
The use of biochar was proposed to enhance the composting process conditions, according to the data found in the literature. Some of the positive effects of biochar include improvement of aeration (see higher average O2 concentrations in the headspace of Reactor 2, Figure 3) due to the porous structure and low density of biochar (Malinowski et al., 2019), prevention of over-drying of the material (see significantly higher moisture content found in Reactor 2, Supplementary Table S3) due to evaporation resulting from its water-storage capacity (Akdeniz, 2019), and shortening of microbial lag-phases needed to enter the thermophilic phase (Chen et al., 2010). Moreover, the microbial community can be influenced in various ways by the addition of biochar (Akdeniz, 2019) since it can serve as a habitat for microorganisms by protecting them from desiccation and serving as a source of nutrients (Jindo et al., 2012) besides fostering aeration that is essential for microorganism health. Biochar also has electro-active properties (Schievano et al., 2019). Electro-activity can play a fundamental role in facilitating electron flow toward terminal electron acceptors (such as O2, NO3-, and SO42-), especially in oxygen-deficient environments. These properties, combined with the observed higher O2 headspace concentrations and higher moisture contents, may have been decisive in speeding up oxidation rates and biodegradation yields, as observed in the presence of biochar (Reactor 2), compared to Reactor 1. Much deeper insights into such mechanisms should be investigated to better understand and express their potentials for improving CHRS processes.
In general, all data demonstrate that the characteristics of biochar increased aeration and moisture content, and provided an overall better condition for degradation that had increased efficiency with a subsequent higher heat production, as confirmed by the higher temperatures registered inside Reactor 2. This was a significance of the present research since a higher temperature is a key parameter for real-scale CHRS implementation. Moreover, Reactor 2 proved a faster reactivity after external feeding, indicating that the highest temperatures were reached in a shorter time compared to Reactor 1. These results are in line with the findings of Mao et al. (2018), López-Cano et al. (2016), and Li et al. (2015), who reported that biochar addition accelerates the rate of temperature increase (6–7 days earlier). Moreover, Wei et al. (2014) reported that the addition of biochar shortened the time needed to start the thermophilic phase (3 days instead of 5–7 days). Several authors reported that compost material containing added biochar reached higher temperatures during the composting process (Chen et al., 2010; Steiner et al., 2010; Wei et al., 2014; Czekała et al., 2016).
The beneficial effects of biochar addition for Reactor 2 reflected in higher VS and TOC variations from the initial phase to the end of the process. This can be observed for the values given in Table 3 and Supplementary Table S3, where the TOC in reactors 1 and 2 decreased by 10.47% and 22.86%, respectively. It was concluded that such results resulted from the biochar effects on enhanced aerobic degradation due to better aeration, over-drying prevention and providing a better environment for microorganisms, and finally, supporting higher degradation rates and faster reactivities.
5 Conclusion
The addition of 10% (w/w) biochar to the composting body increased biodegradation yields by approximately 50% (calculated either using VS, TOC, or LHV balance) and improved oxidation rates over readily biodegradable organic fractions (addition of cheese whey). On average, temperature peaks lasted 4 h less in Reactor 2 than in Reactor 1. The temperature was, on average, 1.34°C higher in the presence of biochar. The heat extraction flux was also improved by the presence of biochar (0.3 kW/m3) versus the absence of biochar (0.1 kW/m3). Microbial analysis highlighted a higher concentration of thermophilic species (Thermoleophilia) and a lower concentration of well-known pathogenic and antibiotic-resistant genera (Leuconostoc, Enterobacter, Luteimonas, and Enterococcus) in the presence of biochar.
Overall, the addition of biochar promotes and biologically stabilizes the aerobic composting process, allowing for better heat recovery from tree-pruning materials. The expected impact is that of an inexpensive optimization solution, such as the one proposed, to encourage the application of the CHRS as a solution for households and small farms in off-grid areas.
Data availability statement
The raw data supporting the conclusion of this article will be made available by the authors, without undue reservation.
Author contributions
AP: conceptualization, data curation, formal analysis, investigation, methodology, project administration, supervision, validation, visualization, and writing–original draft. RM: data curation, writing–original draft, and writing–review and editing. SB: writing–review and editing. RR: investigation, methodology, and writing–review and editing. AS: conceptualization, data curation, formal analysis, investigation, methodology, project administration, supervision, validation, visualization, writing–original draft, and writing–review and editing.
Funding
The author(s) declare that financial support was received for the research, authorship, and/or publication of this article. This project was partially funded by the Cariplo Foundation, Italy, on the call “Beni Aperti”—Project 2018-1426—“CasciNet & Cascina Sant’Ambrogio Reinvenzione contemporanea di un monastero del 1100.”
Acknowledgments
The authors thank BiokW Srl of Trento (TN, Italy) and the CNR-IVALSA Institute of BioEconomy of Trento (TN) for providing the biochar needed to carry out the experiment.
Conflict of interest
The authors declare that the research was conducted in the absence of any commercial or financial relationships that could be construed as a potential conflict of interest.
Publisher’s note
All claims expressed in this article are solely those of the authors and do not necessarily represent those of their affiliated organizations, or those of the publisher, the editors, and the reviewers. Any product that may be evaluated in this article, or claim that may be made by its manufacturer, is not guaranteed or endorsed by the publisher.
Supplementary material
The Supplementary Material for this article can be found online at: https://www.frontiersin.org/articles/10.3389/fenrg.2023.1327136/full#supplementary-material
References
Akdeniz, N. (2019). A systematic review of biochar use in animal waste composting. Waste Manag. 88, 291–300. doi:10.1016/j.wasman.2019.03.054
Azim, K., Soudi, B., Boukhari, S., Perissol, C., Roussos, S., and Thami Alami, I. (2018). Composting parameters and compost quality: a literature review. Org. Agric. 8 (2), 141–158. doi:10.1007/s13165-017-0180-z
Bagra, K., Bellanger, X., Merlin, C., Singh, G., Berendonk, T. U., and Klümper, U. (2023). Environmental stress increases the invasion success of antimicrobial resistant bacteria in river microbial communities. Sci. Total Environ. 904, 166661. doi:10.1016/j.scitotenv.2023.166661
Bhatti, A. A., Haq, S., and Bhat, R. A. (2017). Actinomycetes benefaction role in soil and plant health. Microb. Pathog. 111, 458–467. doi:10.1016/j.micpath.2017.09.036
Bona, D., Beggio, G., Weil, T., Scholz, M., Bertolini, S., Grandi, L., et al. (2020). Effects of woody biochar on dry thermophilic anaerobic digestion of organic fraction of municipal solid waste. J. Environ. Manag. 267, 110633. doi:10.1016/j.jenvman.2020.110633
Bugg, T. D. H., Williamson, J. J., and Rashid, G. M. M. (2020). Bacterial enzymes for lignin depolymerisation: new biocatalysts for generation of renewable chemicals from biomass. Curr. Opin. Chem. Biol. 55, 26–33. doi:10.1016/j.cbpa.2019.11.007
Casado Muñoz, M. del C., Benomar, N., Lerma, L. L., Gálvez, A., and Abriouel, H. (2014). Antibiotic resistance of Lactobacillus pentosus and Leuconostoc pseudomesenteroides isolated from naturally-fermented Aloreña table olives throughout fermentation process. Int. J. Food Microbiol. 172, 110–118. doi:10.1016/j.ijfoodmicro.2013.11.025
Chen, Y. X., Huang, X. D., Han, Z. Y., Huang, X., Hu, B., Shi, D. Z., et al. (2010). Effects of bamboo charcoal and bamboo vinegar on nitrogen conservation and heavy metals immobility during pig manure composting. Chemosphere 78, 1177–1181. doi:10.1016/j.chemosphere.2009.12.029
Czekała, W., Malińska, K., Cáceres, R., Janczak, D., Dach, J., and Lewicki, A. (2016). Co-composting of poultry manure mixtures amended with biochar - the effect of biochar on temperature and C-CO2 emission. Bioresour. Technol. 200, 921–927. doi:10.1016/j.biortech.2015.11.019
Di Fraia, S., Fabozzi, S., Macaluso, A., and Vanoli, L. (2020). Energy potential of residual biomass from agro-industry in a Mediterranean region of southern Italy (Campania). J. Clean. Prod. 277, 124085. doi:10.1016/j.jclepro.2020.124085
Duran, K., van den Dikkenberg, M., van Erven, G., Baars, J. J. P., Comans, R. N. J., Kuyper, T. W., et al. (2022). Microbial lignin degradation in an industrial composting environment. Bioresour. Technol. Rep. 17, 100911. doi:10.1016/j.biteb.2021.100911
Fan, S., Li, A., ter Heijne, A., Buisman, C. J. N., and Chen, W.-S. (2021). Heat potential, generation, recovery and utilization from composting: a review. Resour. Conservation Recycl. 175, 105850. doi:10.1016/j.resconrec.2021.105850
Fan, Y. V., Klemeš, J. J., Lee, C. T., and Ho, C. S. (2018). Efficiency of microbial inoculation for a cleaner composting technology. Clean Technol. Environ. Policy 20, 517–527. doi:10.1007/s10098-017-1439-5
Hu, D., Zang, Y., Mao, Y., and Gao, B. (2019). Identification of molecular markers that are specific to the class thermoleophilia. Front. Microbiol. 10, 1185–1213. doi:10.3389/fmicb.2019.01185
Jeong, D. W., and Lee, J. H. (2015). Antibiotic resistance, hemolysis and biogenic amine production assessments of Leuconostoc and Weissella isolates for kimchi starter development. Lwt 64, 1078–1084. doi:10.1016/j.lwt.2015.07.031
Jindo, K., Sánchez-Monedero, M. A., Hernández, T., García, C., Furukawa, T., Matsumoto, K., et al. (2012). Biochar influences the microbial community structure during manure composting with agricultural wastes. Sci. Total Environ. 416, 476–481. doi:10.1016/j.scitotenv.2011.12.009
Kirby, R. (2005). Actinomycetes and lignin degradation. Adv. Appl. Microbiol. 58, 125–168. doi:10.1016/S0065-2164(05)58004-3
Kumar, S., Bansal, K., and Sethi, S. K. (2022). Comparative genomics analysis of genus Leuconostoc resolves its taxonomy and elucidates its biotechnological importance. Food Microbiol. 106, 104039. doi:10.1016/j.fm.2022.104039
Lehmann, J., and Joseph, S. (2015). Biochar for environmental management: science, technology and implementation. Routledge.
Li, R., Wang, Q., Zhang, Z., Zhang, G., Li, Z., Wang, L., et al. (2015). Nutrient transformation during aerobic composting of pig manure with biochar prepared at different temperatures. Environ. Technol. (United Kingdom) 36, 815–826. doi:10.1080/09593330.2014.963692
López-Cano, I., Roig, A., Cayuela, M. L., Alburquerque, J. A., and Sánchez-Monedero, M. A. (2016). Biochar improves N cycling during composting of olive mill wastes and sheep manure. Waste Manag. 49, 553–559. doi:10.1016/j.wasman.2015.12.031
Malesani, R., Pivato, A., Bocchi, S., Lavagnolo, M. C., Muraro, S., and Schievano, A. (2021). Compost Heat Recovery Systems: an alternative to produce renewable heat and promoting ecosystem services. Environ. Challenges 4, 100131. doi:10.1016/j.envc.2021.100131
Malinowski, M., Wolny-Koładka, K., and Vaverková, M. D. (2019). Effect of biochar addition on the OFMSW composting process under real conditions. Waste Manag. 84, 364–372. doi:10.1016/j.wasman.2018.12.011
Mao, H., Lv, Z., Sun, H., Li, R., Zhai, B., Wang, Z., et al. (2018). Improvement of biochar and bacterial powder addition on gaseous emission and bacterial community in pig manure compost. Bioresour. Technol. 258, 195–202. doi:10.1016/j.biortech.2018.02.082
Ohore, O. E., Wei, Y., Wang, Y., Nwankwegu, A. S., and Wang, Z. (2022). Tracking the influence of antibiotics, antibiotic resistomes, and salinity gradient in modulating microbial community assemblage of surface water and the ecological consequences. Chemosphere 305, 135428. doi:10.1016/j.chemosphere.2022.135428
Schievano, A., Berenguer, R., Goglio, A., Bocchi, S., Marzorati, S., Rago, L., et al. (2019). Electroactive biochar for large-scale environmental applications of microbial electrochemistry. ACS Sustain. Chem. Eng. 7, 18198–18212. doi:10.1021/acssuschemeng.9b04229
Smith, M. M., and Aber, J. D. (2017). Heat Recovery from Composting: a step-by-step guide to building an aerated static pile heat recovery composting facility.
Steiner, C., Das, K. C., Melear, N., and Lakly, D. (2010). Reducing nitrogen loss during poultry litter composting using biochar. J. Environ. Qual. 39, 1236–1242. doi:10.2134/jeq2009.0337
Wang, G., Kong, Y., Yang, Y., Ma, R., Li, L., Li, G., et al. (2022a). Composting temperature directly affects the removal of antibiotic resistance genes and mobile genetic elements in livestock manure. Environ. Pollut. 303, 119174. doi:10.1016/j.envpol.2022.119174
Wang, S., Gao, Y., Sun, Z., Peng, X., Xie, C., and Tang, Y. (2022b). Thermophilic semi-continuous composting of kitchen waste: performance evaluation and microbial community characteristics. Bioresour. Technol. 363, 127952. doi:10.1016/j.biortech.2022.127952
Wang, Y., Akdeniz, N., and Yi, S. (2021). Biochar-amended poultry mortality composting to increase compost temperatures, reduce ammonia emissions, and decrease leachate’s chemical oxygen demand. Agric. Ecosyst. Environ. 315, 107451. doi:10.1016/j.agee.2021.107451
Waqas, M., Nizami, A. S., Aburiazaiza, A. S., Barakat, M. A., Ismail, I. M. I., and Rashid, M. I. (2018). Optimization of food waste compost with the use of biochar. J. Environ. Manag. 216, 70–81. doi:10.1016/j.jenvman.2017.06.015
Wei, L., Shutao, W., Jin, Z., and Tong, X. (2014). Biochar influences the microbial community structure during tomato stalk composting with chicken manure. Bioresour. Technol. 154, 148–154. doi:10.1016/j.biortech.2013.12.022
Yang, X., Wang, H., Strong, P. J., Xu, S., Liu, S., Lu, K., et al. (2017). Thermal properties of biochars derived from waste biomass generated by agricultural and forestry sectors. Energies 10, 469. doi:10.3390/en10040469
Zhao, R. F., Gao, W., and Guo, H. Q. (2017). Comprehensive review of models and methods used for heat recovery from composting process. Int. J. Agric. Biol. Eng. 10, 1–12. doi:10.25165/j.ijabe.20171004.2292
Keywords: compost heat recovery system, woodchips, sustainable heat, waste to energy, agroforestry
Citation: Pivato A, Malesani R, Bocchi S, Rafieenia R and Schievano A (2024) Biochar addition to compost heat recovery systems improves heat conversion yields. Front. Energy Res. 11:1327136. doi: 10.3389/fenrg.2023.1327136
Received: 03 November 2023; Accepted: 31 December 2023;
Published: 18 January 2024.
Edited by:
Surendra Sarsaiya, Zunyi Medical University, ChinaReviewed by:
Liwen Luo, Hong Kong Baptist University, Hong Kong SAR, ChinaRanjan Singh, Dr. Rammanohar Lohia Avadh University, India
Copyright © 2024 Pivato, Malesani, Bocchi, Rafieenia and Schievano. This is an open-access article distributed under the terms of the Creative Commons Attribution License (CC BY). The use, distribution or reproduction in other forums is permitted, provided the original author(s) and the copyright owner(s) are credited and that the original publication in this journal is cited, in accordance with accepted academic practice. No use, distribution or reproduction is permitted which does not comply with these terms.
*Correspondence: Alberto Pivato, YWxiZXJ0by5waXZhdG9AdW5pcGQuaXQ=