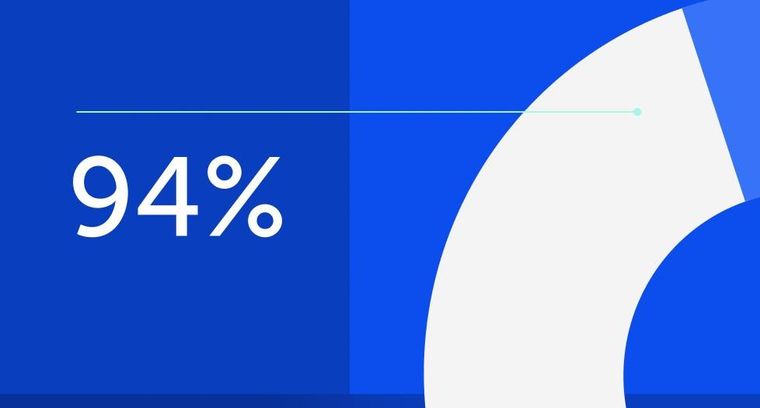
94% of researchers rate our articles as excellent or good
Learn more about the work of our research integrity team to safeguard the quality of each article we publish.
Find out more
ORIGINAL RESEARCH article
Front. Energy Res., 06 October 2023
Sec. Sustainable Energy Systems
Volume 11 - 2023 | https://doi.org/10.3389/fenrg.2023.1291213
This article is part of the Research TopicBuilding Energy System Retrofit and Optimization in Small Energy CommunitiesView all 4 articles
The roof plays a pivotal role as it directly engages with solar radiation absorption and external heat exchange, significantly influencing the building’s overall energy dynamics. To assess the performance of green roofs and cool roofs, a roof performance test facility was established in Nanjing. Time-difference comparison experiments were conducted to measure and analyze the energy-saving effects of cool roofs, green roofs, and conventional roofs during both summer and winter conditions. The study aimed to investigate how their thermal performance impacts building energy consumption. The study’s findings reveal that under summer conditions, the incorporation of a cool roof system leads to a substantial enhancement in energy efficiency, achieving an impressive 13.2% energy savings compared to conventional roofing solutions. In contrast, the implementation of a green roof system results in a more modest energy-saving rate of 4.1%. Transitioning to winter conditions, the adoption of a cool roof system shows a marginal increase of 2.8% in energy consumption compared to conventional roofs. Interestingly, the green roof system stands out as an energy-efficient option during winter, demonstrating a significant 4.9% reduction in energy consumption. This approach ensured reliable and valid results to provide a comprehensive view of how different roof types respond to varying climatic conditions.
Urbanization is closely intertwined with building energy consumption, a factor that has exhibited a persistent upward trajectory. This escalating trend underscores the growing significance of building energy consumption within the broader landscape of societal energy utilization, currently approximating 30% (Xu and Wang, 2020; Olu-Ajayi et al., 2022; Huang et al., 2023). The building roof, often metaphorically termed the “fifth facade,” has transcended its conventional structural role, evolving into a multifaceted platform with pronounced energy-efficient attributes. This transformation is attributed to its capacity to harmonize indoor thermal environments and concomitantly ameliorate the aggregate building energy consumption. Scholarly sources affirm that within urban contexts, building roofs encompass an expansive expanse, constituting approximately 25% of the total urban area (Zhao and Zhang, 2023). Furthermore, roofs emerge as the primary recipient of intense and unfiltered solar radiation, rendering them an optimal canvas for the assimilation of diverse energy-conserving technologies. The astute exploitation of the latent capacities inherent in building roofs, coupled with the strategic deployment of an array of energy-saving methodologies, holds the promise of mitigating urban energy consumption to a certain degree. In doing so, it plays a pivotal role in advancing China’s imperative objectives of realizing “peak carbon emissions” and attaining a state of “carbon neutrality” within specified timelines.
Existing energy-efficient roofing technologies encompass ventilated roofs (Gagliano et al., 2012; Kain et al., 2020; Hou et al., 2021; Ingebretsen et al., 2022), solar roofs (Wang and Zhai, 2010; Chaianong and Pharino, 2015; Ayadi and Al-Dahidi, 2019; Bódis et al., 2019; Evangelisti et al., 2019; Gassar and Cha, 2021), reflective roofs (Jo et al., 2010; Hernández-Pérez et al., 2014; Hernández-Pérez et al., 2018), and green roofs (Shafique et al., 2018; Cascone, 2019; Manso et al., 2021). Amid these strategies, green roofs stand as the predominant passive roofing energy-saving technique, second only to insulation methodologies, and have garnered extensive adoption. Beyond their thermal insulation attributes, green roofs exert a salient influence on localized ecological amelioration. They epitomize an exemplary paradigm of rooftop design for civilian structures, harmonizing ecological considerations, energy conservation imperatives, and economic advantages into a cohesive framework. This amalgamation finds particular resonance in residential edifices situated within regions characterized by pronounced climatic oscillations, featuring scorching summers and frigid winters.
The genesis of cool roofs can be traced back to their precursor, namely, reflective roofs. Conventional reflective roofing entails the application of luminous-hued, high-reflectance coatings onto surfaces, thereby curtailing the absorption of solar radiation. This approach imparts several merits, including the attenuation of surface temperatures, mitigation of diurnal thermal oscillations, and safeguarding against roof fissures.
The concept of cool roofs was initially introduced by Parker and Barkaszi (1997). Differing from conventional reflective roofs, cool roofs emphasize not only reducing the absorption of solar radiation (by increasing solar reflectance), but also maximizing the emissivity of roof materials for longwave atmospheric radiation. In other words, the goal is to minimize absorption and maximize emission, thereby lowering the surface temperature of the roof and reducing the direct heat transfer from the roof into the indoor spaces of the building. Parker’s testing on nine representative buildings in the state of Florida, USA, demonstrated that the use of cool roof materials led to energy savings of 19% for residential buildings and 25% for commercial buildings (Florida Solar Energy Center and Parker, 1997).
Pisello and Cotana (2014) conducted a thermal reflection retrofit on a traditional building in Italy. By comparing the conditions before and after the retrofit, they found that the average indoor temperature decreased by 4.7°C during the summer and by 1.2°C during the winter. This outcome indicates a significant reduction in building cooling load during the summer and a slight increase in building heating load during the winter.
Rawat and Singh (2022) summarized the thermal performance of cool roofs with different types of surface coatings in various climatic regions. Their analysis revealed that the average energy savings achieved by cool roofs in different climatic zones (temperate, tropical, and mixed zones) ranged from 15% to 35.7%. Furthermore, by employing cool roof technology, the average roof surface temperature could be lowered by 1.4°C–4.7°C.
Levinson, affiliated with the Lawrence Berkeley National Laboratory in the United States, conducted a comprehensive inquiry encompassing both theoretical and empirical dimensions concerning cool roofs. This comprehensive investigation encompassed a meticulous examination of diverse materials and colors employed for cool roof construction. It involved a detailed analysis of their attributes pertaining to solar spectrum absorption and radiation properties, culminating in the establishment of an extensive repository cataloging the material properties of cool roofs. Employing an expansive repertoire of engineering field tests, this research offered empirical validation. Notably, it ascertained that the adoption of cool roofs could engender appreciable energy savings, ranging from 7% to 21.5%, in the context of air conditioning cooling energy consumption during California’s summer months. The study also ventured into the realm of mitigating the urban heat island phenomenon, revealing the substantial impact of cool roofs in this regard. Moreover, it explored their viability across multifarious domains, including automobiles, roads, walls, and bridges, underscoring the far-reaching applicability of this technology (Levinson et al., 2005a; Levinson et al., 2005b).
In recent years, the exploration of radiative cooling mechanisms, leveraging the vast expanse of the sky as a heat dissipation reservoir, has garnered escalating scholarly interest. Researchers across the global landscape, as well as within domestic contexts, have devoted substantial efforts to intricate theoretical investigations and empirical explorations pertaining to the fundamental underpinnings, operational methodologies, and material engineering associated with radiative cooling (Zhang et al., 2018; Zhao et al., 2019; Wu et al., 2022; Tian et al., 2023). At present, investigations into radiative cooling are predominantly centered around two principal domains: coating reflectivity and infrared emission.
The implementation of radiative cooling strategies, wherein the expansive sky serves as a heat dissipation reservoir, commonly involves the utilization of silver coatings or substrates enriched with micro or nano-particles, functioning as reflective layers. By selecting tailored materials endowed with selective emission attributes, electromagnetic waves are emitted via thermal radiation within the spectral range of the atmospheric window (8–13 μm). This facilitates the efficacious cooling of surfaces to notably low temperatures when interacting with the frigid conditions of outer space. It is important to acknowledge, however, that the realm of radiative cooling materials presents certain hurdles that warrant attention. Notably, challenges encompassing elevated costs and the vulnerability of materials to degradation within exposed environments, precipitating a decline in performance, necessitate concerted efforts to be surmounted.
The review of existing literature underscores that prevailing international research on cool roofs primarily centers around geographical locales marked by intense solar radiation and substantial cooling demands. It is evident that the climatic nuances abroad deviate from the domestic environment, particularly in regions characterized by contrasting climatic patterns of scorching summers and frigid winters. Consequently, the theoretical constructs and methodologies conceived abroad may not offer seamless transference to domestic scenarios. In recent times, the scope of cool roof research within China has predominantly revolved around two pivotal dimensions. Firstly, there is a pronounced emphasis on the investigation of heat-reflective coating materials. Secondly, extensive theoretical and empirical inquiries have been undertaken to unravel the energy-conserving attributes of heat-reflective roofs within air conditioning systems. It becomes evident that the focal point of domestic research revolves primarily around the heat-reflective properties intrinsic to cool roofs. However, it is discernible that the exploration of their equally significant high emissivity attributes, instrumental for radiative cooling, remains relatively incipient in the domestic context.
The current study is focused on validating the energy-saving benefits of cool roofs when compared to alternative roofing systems in a climate characterized by hot summers and cold winters. To accomplish this objective, the investigation involved the following key tasks.
1) Adjustment of Laboratory Conditions: The initial laboratory conditions were adapted and refined to establish an experimental framework suitable for studying energy-efficient roofing technologies.
2) Comparative Analysis using Time-Differential Contrasts: A methodological framework was developed to comprehensively examine and empirically evaluate the energy-saving capabilities of both green roofs and cool roofs. This involved a meticulous comparison of the energy-saving effects and differences inherent in green roofs and cool roofs across various operational conditions, including cooling needs in summer and heating requirements in winter.
3) Comprehensive Overview of Seasonal Cool Roof Performance: The unique operational characteristics exhibited by cool roofs in both winter and summer seasons were systematically summarized. This synthesis provides valuable guidance and serves as a reference point for the practical implementation of cool roofs in various engineering applications.
Through these efforts, this study aims to deepen our understanding of the energy-saving potential offered by cool roofs in climates marked by hot summers and cold winters. Additionally, it seeks to provide practical insights relevant to the real-world adoption of cool roofs, bridging the gap between theoretical advancements and practical applications.
At present, on the global stage, three predominant experimental methodologies are recognized for investigating building energy consumption. The first approach entails the selection of two congruent rooms characterized by uniform thermal attributes, orientation, energy utilization patterns, and HVAC systems. Monitoring encompasses energy consumption patterns as well as temperature and humidity fluctuations within both chambers. Subsequent to data collection, disparities between the two spaces are scrutinized. However, a notable challenge associated with this method lies in its endeavor to mitigate the impact of heat transfer through the walls, which could potentially emanate from adjoining spaces and consequently impede the accuracy of results.
The second testing methodology involves the utilization of a chamber model strategy, wherein two entirely identical chamber models are meticulously fabricated. A critical prerequisite is the establishment of an adequately voluminous model to ensure the maintenance of natural airflow patterns within. Following the calibration of thermal performance to guarantee parity, encompassing uniform thermal attributes, orientation, energy consumption profiles, and HVAC systems, the monitoring of energy consumption and temperature/humidity transpires in both chambers. This method effectively circumvents the interference attributed to heat transfer from adjacent spaces. However, it necessitates the careful consideration of chamber size, which must be sufficiently substantial to curtail significant discrepancies vis-à-vis actual building energy utilization patterns.
The third testing approach entails an examination of a singular room before and after modifications, thereby facilitating a comparative analysis of alterations in energy consumption and temperature/humidity profiles. This technique guarantees the preservation of identical thermal attributes within the room and attains heightened accuracy through the elimination of external interferences. However, a limitation of this method is its inability to ensure consistent outdoor weather parameters between the pre-modification and post-modification phases. Consequently, the overall building energy consumption values may carry diminished significance as reference points. Nevertheless, this approach remains pertinent for the evaluation of energy-saving enhancements and interventions, wherein the proportion of energy conservation can be presumed to remain relatively consistent under similar weather conditions.
Taking into consideration factors like experimental site suitability and the effectiveness of the experiments, this study employs a combination of the second and third methods. In other words, a time-differential comparative experimental design is used. For cool roofs, green roofs, and conventional roofs, two identical chamber models of the same scale are constructed. The energy-saving effects of cool roofs and green roofs are determined by comparing them against the conventional roof. The specific procedures are as follows.
1) Select 2 days with weather conditions as close as possible. On the first day, measure the energy consumption of the conventional roof and the green roof to determine the energy-saving rate of the green roof.
2) Apply cool roof coating to the conventional roof chamber for the second day’s test.
3) On the second day, measure the energy consumption of the cool roof and the green roof with the cool coating applied. Calculate the energy-saving rate of the cool roof relative to the green roof, using the previously obtained energy-saving rate of the green roof relative to the conventional roof.
1) Select 2 days with similar weather conditions. Test the energy consumption of the cool roof and the green roof with the cool coating applied to determine the energy usage difference between the two.
2) Apply conventional coating to the cool roof chamber for the second day’s test.
3) Measure the energy consumption of the conventional roof and the green roof with the conventional coating applied. Calculate the energy usage difference between the conventional roof and the green roof using the previously obtained data for the cool roof and green roof comparison.
This experimental approach appears to be well-designed for comparing the energy-saving characteristics of cool roofs and green roofs under summer cooling and winter heating conditions. It accounts for weather variations and uses a time-differential comparison to yield valuable insights into the performance of these roofing systems.
The experimental configuration is situated atop the Cooling Science Laboratory at Southeast University’s Sipailou Campus. This building boasts an approximate height of 8 m. Notably, tall edifices of varying orientations are situated at a significant remove from the testing locale, thus effectively curtailing their capacity to influence experimental readings. The rooftop enjoys abundant natural illumination and is devoid of extraneous heat sources, as vividly illustrated in Figure 1.
In order to facilitate the smooth progress of the experiment, the following idealized assumptions were made.
1) Only external heat gains of the building were considered, while internal heat sources such as lighting, outlets, and human activities were disregarded.
2) To align with the working hours of typical office buildings, measurements and calculations were limited to the period from 9:00 a.m. to 5:00 p.m.
3) Heat transfer through the floor, both upward and downward, was not taken into account.
4) Given the impracticality of identical weather conditions on two consecutive days, the experiment selected 2 days with weather parameters as close as possible to typical meteorological conditions to ensure the experiment’s accuracy.
In order to mitigate heat dissipation from the enclosure structure and augment the precision of the experiments, both chambers are fabricated employing high-performance 50 mm sandwiched polystyrene panels. The exterior dimensions of each chamber are delineated as follows: Length × Width × Height = 2,200 mm × 1,980 mm × 1,900 mm. These chambers are further fortified by a 600 mm high angle steel base support positioned underneath, ensuring structural stability and elevation.
In the context of this experiment, a proprietary green roof comprising Manila grass sod is implemented. The choice of Manila grass is underpinned by its recognized attributes encompassing resilience against drought, capacity to withstand high temperatures, tolerance to shaded environments, resistance to foot traffic, rapid growth, and straightforward maintenance regimen. The specific configuration of the green roof for this experiment entails a soil layer thickness of approximately 2 cm, with the grass attaining an approximate height of 8 cm.
In the case of the cool roof, an application of ZS-222 type cool roof coating is administered via a spraying technique. The salient attributes characterizing this coating are comprehensively summarized in Table 1. As for the conventional roof, a standard gray-colored coating is brushed onto the surface, boasting a quantified reflectance value of approximately 0.45.
Both thermal testing chambers, along with their enveloping protective enclosures, are skillfully assembled utilizing high-performance 50 mm sandwiched polystyrene panels. It is projected that the cooling load for each chamber approximates 300 W, while the corresponding heating load is estimated at approximately 450 W.
Given the complexities entailed in acquiring a 200 W small refrigeration unit and with a strategic intent to streamline expenses while leveraging available resources, the cooling unit enlisted for this configuration capitalizes on an extant laboratory asset. Specifically, a compact vortex-type air-cooled heat pump unit with a maximum cooling capacity of 8.2 kW is employed. Furthermore, a 0.8 L capacity energy storage water tank is seamlessly integrated into the setup. Its principal function pertains to ensuring a sustained water supply temperature, thus effectively curtailing unwarranted fluctuations in indoor temperatures.
In order to establish a regimen of consistent and reliable operation for the air-cooled heat pump unit, a judicious selection has been made of an FSFP-34-LA fan coil unit, bolstered by an electric heater component. This electric heater assumes a pivotal role in counterbalancing any surplus cooling capacity generated during operation, thereby ensuring the maintenance of the indoor temperature at the designated set point. This strategy is predicated on guaranteeing both the stability and dependability of the system’s functioning while concurrently optimizing costs through the judicious utilization of extant laboratory assets. An additional advantage intrinsic to this approach is the dynamic control it affords. By modulating the heating power of the electric heater, the capacity for adjustment in heating and cooling capabilities is realized. This stands in contrast to traditional refrigeration system modifications and confers a distinct advantage in terms of operational flexibility and precision.
The testing chambers are outfitted with a reheat-type fan coil system, incorporating a temperature controller that functions in consonance with the designated temperature set point and the temperature differential perceived by the embedded temperature sensor. The orchestration of the electric heater’s operation is governed by this controller, as illustrated in Figure 2. Following the attainment of system stabilization, the ensuing temperature oscillations within the testing chambers are adeptly contained within a narrow range of ±0.3°C.
The crux of the experiment revolves around the comprehensive testing and real-time monitoring of a gamut of parameters.
1) Roof inner surface temperature (at two distinct measurement points)
2) Internal chamber temperature (at two distinct measurement points)
3) Inlet and outlet water temperatures (captured at four distinct measurement points)
4) Flow rates (evaluated at two distinct measurement points)
5) Vertical surface solar radiation (monitored at a singular measurement point)
6) Fan power consumption (measured at two separate points)
7) Electric heater power consumption (assessed at two discrete points)
This meticulous assessment endeavors to provide a holistic perspective on the experiment’s dynamics and outcomes.
Outdoor Environmental Parameter Testing System: The efficacy evaluation of the chamber’s energy-saving performance hinges upon the interplay with outdoor environmental factors, encompassing solar radiation, outdoor dry bulb temperature, relative humidity, wind speed, and wind direction. To this end, the outdoor environmental testing system is designed to systematically gather and archive hourly data concerning the following parameters.
1) Total solar radiation on the horizontal plane
2) Total solar radiation on the vertical surface of the chamber
3) Outdoor dry bulb temperature
4) Relative humidity
5) Wind speed
6) Wind direction
Nanjing is located in a region characterized by hot summers and cold winters, belonging to the subtropical monsoon climate zone, with a Köppen climate classification of “Cfa.” Specifically, Nanjing’s climate features four distinct seasons, with hot and humid summers, cold and dry winters, and pleasant temperatures during the transitional periods of spring and autumn. It is also influenced by monsoons, resulting in rainy summers and relatively dry winters.
The schematic representation of this system is vividly illustrated in Figure 3, accentuating its pivotal role in affording a comprehensive understanding of the experiment’s contextual dynamics.
In order to facilitate a comparative evaluation of the energy-saving efficacy across distinct roofing types, the real-time internal surface temperatures of both categories necessitate meticulous monitoring. To achieve this objective, T-type copper-constantan thermocouples have been chosen as the sensing apparatus. These thermocouples are strategically deployed to capture real-time temperature measurements at four designated points. The orchestration of data acquisition is facilitated through the utilization of the Agilent-34972A data acquisition instrument, which records the data at intervals of 10 s. This data collection regimen ensures a comprehensive insight into the temporal temperature variations and their interplay with the different roofing configurations.
To ascertain the cooling load exerted on the two testing chambers throughout the experiment, a comprehensive methodology is adopted. The procedure encompasses real-time monitoring of the power consumption pertaining to the cooling systems within each chamber, culminating in the amalgamation of the acquired data. Through the meticulous assessment of inlet and outlet water temperatures in conjunction with the flow rates of the fan coil units, the cooling load imparted to each chamber is ascertainable over a defined temporal span. An analogous approach is employed to delineate the heating load sustained by each chamber over the same duration. This entails the measurement of power consumption attributed to the fan motors and electric heaters. The differential between these two distinct loads encapsulates the requisite energy for air conditioning within each respective chamber. This analysis offers a nuanced insight into the dynamic interplay of energy dynamics within the experimental setup.
The Yokogawa WT230 real-time monitoring system serves as the instrumental conduit for capturing the power consumption metrics affiliated with the motors of the fan coil units and the electric heaters domiciled within Testing Chamber A and Testing Chamber B. This deployment substantially streamlines the process of energy consumption calculations, ensuring accuracy and efficiency in the assessment of power utilization dynamics.
In accordance with prevailing weather patterns, the dates of July 24th and July 25th have been earmarked as the designated testing days for evaluating summer operating conditions. The duration of testing will span from 09:00 a.m. to 06:00 p.m. on these selected days. The intricacies of temperature variations and solar radiation intensities pertinent to these dates are graphically represented in Figure 4, rendering a comprehensive visual depiction of the atmospheric dynamics during the experimentation period.
FIGURE 4. (A) weather parameters for July 24th, summer season (B) Weather parameters for July 25th, summer season.
The visual representation in Figure 4 distinctly elucidates that, encompassing both July 24th and July 25th, a discernible constancy characterizes the temperature trends spanning from 09:00 a.m. to 06:00 PM. It becomes evident, however, that the solar irradiance intensity encounters pronounced fluctuations, a phenomenon attributed to variables such as cloud coverage and prevailing meteorological conditions. Notably, the intensity reaches its zenith around the noon hour, attaining an approximate value of 1,000 W/m2. This observation underscores the dynamic and intricate interplay of solar radiation dynamics within the designated time frame.
Test Results for July 24th:
Adhering to the prescribed experimental regimen tailored for summer conditions, the primary focus pivoted towards scrutinizing the energy consumption attributes distinctive to the conventional roof and the green roof configurations. Over the duration encompassing 09:00 a.m. to 06:00 p.m., the testing chambers underwent comprehensive evaluations. The ingress of cooling load into the indoor environment was gauged by computing the temperature differential in conjunction with the flow rate of the chilled water. This value, juxtaposed against the electric heater’s power consumption, yields an accurate reflection of the authentic cooling load borne within the chamber. The outcomes of these calculations are vividly portrayed in Figure 5A, furnishing a visual representation of the derived results.
FIGURE 5. (A) Roof cooling load for July 24th, summer season. (B) Roof cooling load for July 25th, summer season.
From Figure 5A, it is evident that starting from 09:00 a.m., the energy consumption of the green roof remains consistently lower than that of the conventional roof. As solar radiation intensity increases and temperatures rise, the maximum cooling load of the conventional roof can exceed 260 W, whereas the cooling load for the same period on the green roof is only 230 W, resulting in a difference of 11.5% in peak loads. Calculations show that during the time span from 09:00 a.m. to 06:00 p.m., the total energy savings for the green roof, compared to the conventional roof, amount to 11.7%.
Test Results for July 25th:
Persisting in adherence to the outlined experimental design tailored for summer conditions, the subsequent phase encompassed the comprehensive examination of energy consumption traits associated with both the heat-reflective cool roof and the green roof configurations. The assessment activities mirrored those of the prior day, occurring within the temporal ambit spanning from 09:00 a.m. to 06:00 PM. Akin to the preceding day, the cooling load infiltrating the indoor realm was deduced through the computation of temperature disparities alongside the flow rate of chilled water. Subsequently, the disparity between this calculated load and the electric heater’s power consumption unveils the genuine cooling load intrinsic to the chamber. The outcomes of these meticulous calculations find visual articulation in Figure 5B, vividly encapsulating the resultant findings.
As elucidated by Figures 5A, B clear pattern emerges wherein the energy consumption attributed to the heat-reflective cool roof consistently trails that of the green roof configuration, commencing from 09:00 a.m. onward. Parallel to previous observations, an incremental surge in solar irradiance intensity coupled with escalating temperatures accentuates this trend. Notably, the zenith of cooling load experienced by the green roof culminates around 210 W, whereas the analogous cooling load of the cool roof merely registers at 200 W. This delineates a discernible 4.8% divergence in peak loads. Broadening the purview to encapsulate the entirety of the time frame spanning from 09:00 a.m. to 06:00 p.m., calculated results concretely affirm that the total energy savings rendered by the cool roof, relative to the green roof configuration, stands at a noteworthy 4.1%. This observation attests to the efficacy of the heat-reflective cool roof in effecting discernible energy conservation amidst the specific summer conditions under examination.
Cool roofs primarily achieve energy savings through high reflectivity and emissivity, whereas green roofs achieve energy savings through the transpiration of plants and the heat storage capacity of the soil. At the end of a summer day, as solar radiation gradually diminishes, the energy savings from the high reflectivity of cool roofs gradually diminish as well. In contrast, green roofs maintain a stable energy-saving benefit due to the transpiration of plants and the heat storage capacity of the soil. Therefore, at the end of a summer day, the energy savings deviation between green roofs and cool roofs tends to converge.
With due consideration to the discerned energy conservation proportions between the green roof and the conventional roof configurations, a salient conclusion can be drawn. Specifically, the cool roof configuration achieves a commendable total energy savings ratio of 13.2% during the operational hours characteristic of the summer season. This finding underscores the significant strides made in energy efficiency through the adoption of the cool roof solution within the ambit of this study.
In alignment with prevailing weather dynamics, the dates of January 3rd and January 4th in the year 2017 were judiciously earmarked for conducting testing during winter conditions. The evaluative phase was conducted within the temporal span spanning from 09:00 a.m. to 18:00 PM. This 2-day interval was thoughtfully selected to facilitate a comprehensive assessment of performance within the context of winter climatic conditions. The intricate interplay of temperature trends and solar radiation intensity levels for these specific dates are distinctly illustrated in Figure 6, offering a graphical representation that encapsulates the atmospheric nuances during the experimentation period.
FIGURE 6. (A) Weather parameters for January 3rd during the winter season. (B) Weather parameters for January 4th during the winter season.
The graphical representation in Figure 6 effectively encapsulates the temperature dynamics during the winter season for January 3rd and January 4th. Spanning from 09:00 a.m. to 06:00 p.m., a noticeable congruence characterizes the temperature trajectories on these days. On January 3rd, the temperature range spanned from a minimum of −2.7°C to a maximum of −0.1°C. Conversely, January 4th witnessed slightly milder conditions, with temperatures oscillating between a low of −0.9°C and a high of 2.1°C. Evidently, the latter day was marked by comparatively elevated temperatures vis-à-vis January 3rd. Furthermore, the solar irradiance intensity conspicuously oscillates, a phenomenon attributed to variables such as cloud coverage. Notably, the intensity crescendos around midday, attaining an approximate zenith of 260 W/m2. This observation distinctly underscores the intricate dynamics governing solar radiation patterns during the specified winter conditions.
January 3rd Test Results:
Adhering to the outlined experimental protocol tailored for the winter season, the focal point of scrutiny shifted towards the energy consumption attributes of both the cool roof and the green roof configurations. Over the temporal span encompassing 09:00 a.m. to 06:00 p.m., a noteworthy facet becomes apparent: owing to the absence of cooling load, the initiation of the chiller unit for cold water was superfluous. Instead, the sole parameter necessitating measurement pertained to the power consumption of the electric heater, which effectively mirrors the genuine indoor heating load. This discerning approach steers the experimental assessment toward an intricate examination of the heating dynamics within the testing chambers. The cumulative outcome of these calculations is graphically depicted in Figure 7A, serving to illustrate the findings rendered through meticulous analysis of the available data.
Initiating from 09:00 a.m., a discernible pattern emerges wherein the heating load gradually recedes in tandem with the escalating solar radiation intensity and ascending temperatures. Around 5:00 p.m., a pivotal shift becomes perceptible: the radiation intensity diminishes, initiating a decline in temperature and an attendant increase in the heating load. Notably, during this period, the minimal heating load attributable to the green roof configuration approximates 300 W. Conversely, the corresponding heating load for the cool roof configuration registers at approximately 330 W. Cumulative computations undertaken across the temporal span of 09:00 a.m. to 6:00 p.m. coalesce to yield a definitive outcome. Specifically, the total energy savings facilitated by the green roof, relative to the cool roof configuration, amount to a substantial 7.8%. This revelation accentuates the noteworthy potential of the green roof solution in orchestrating energy conservation during the specified winter conditions under examination.
January 4th Test Conditions:
Remaining in accordance with the stipulated experimental protocol tailored for the winter season, the ensuing phase revolves around assessing the energy performance characteristics of both the conventional roof and the green roof configurations. Spanning from 09:00 a.m. to 6:00 p.m., the test chambers are engaged within this time frame. It is pertinent to note that in the absence of any discernible cooling load, the chiller unit for cold water remains dormant throughout the evaluation. Consequently, the exclusive parameter that demands scrutiny pertains to the power consumption of the electric heater–a parameter that faithfully mirrors the authentic indoor heating load. The culmination of these meticulous measurements is artfully illustrated in Figure 7B, furnishing a visual representation that effectively encapsulates the outcomes of the comprehensive analysis of data.
The insights drawn from Figure 7B resonate with compelling clarity. Commencing at 09:00 a.m., a discernible trend becomes evident: the heating load progressively diminishes in tandem with the mounting solar radiation intensity and concurrent temperature elevation. The turning point manifests around 5:00 p.m., as the solar radiation intensity wanes, instigating a temperature decrease and consequently triggering an uptick in the heating load. During this interval, the minimal heating loads attributable to both the green roof and the regular roof configurations are strikingly similar, approximating 275 W.
During winter, the transpiration activity of plants on green roofs decreases, and this reduced transpiration can have adverse effects on heating inside buildings. Therefore, the primary factors influencing the energy-saving performance of green roofs during winter are the reflectivity of the vegetation layer and the heat storage capacity of the soil. At the end of a winter day, as solar radiation gradually diminishes, the reflectivity benefits of the vegetation layer gradually diminish as well. Simultaneously, the soil layer reaches a saturation point in terms of heat storage, and as temperatures gradually rise (as shown in Figure 6), the energy-saving effects of green roofs and regular roofs gradually become more consistent.
The cumulative reckoning spans from 09:00 a.m. to 18:00 p.m., culminating in a definitive inference. Specifically, the green roof showcases a commendable energy reduction rate of 4.9% when juxtaposed with the conventional roof. Notably, upon extrapolating the energy savings attributable to the green roof and the conventional roof, a nuanced insight emerges. The cool roof configuration, operating during the winter operational hours, begets a marginally detrimental outcome: an approximate 2.8% increment in energy consumption compared to the conventional roof. A pivotal factor underlying this outcome pertains to the cool roof’s pronounced capacity to reflect solar radiation, which inhibits the absorption of heat from the natural solar source during winter. Concurrently, the cool roof’s elevated emissivity incessantly releases heat outward, amplifying the building’s demand for heating loads. This symbiotic interplay contributes to the observed increase in energy consumption, establishing the cool roof’s suboptimal performance during winter heating conditions.
The study’s implications transcend mere energy savings, underscoring the imperative of encompassing broader environmental and economic considerations while navigating the realm of roofing choices. While the energy-saving feats of green roofs during summer are undoubtedly remarkable, their value proposition transcends the confines of energy efficiency. Green roofs manifest a multidimensional impact, encompassing air quality amelioration, stormwater management, and urban aesthetics. On a divergent trajectory, the season-dependent performance variations exhibited by cool roofs serve as an unequivocal testament to the intricacy of their thermal attributes. This complexity underscores the necessity for meticulous evaluation and optimization, particularly within locales characterized by harsh winter conditions.
In the realm of pragmatic applications, the study’s outcomes bear the potential to guide architects and building proprietors in adopting judicious roofing strategies, aligned with localized climatic attributes. For regions replete with sweltering summers, the strategic implementation of green roofs could yield remarkable cooling dividends. Conversely, the suitability of cool roofs assumes prominence within domains characterized by unrelenting winter conditions. A noteworthy proposition worth exploring pertains to hybrid solutions that artfully amalgamate the strengths inherent to both green and cool roofs. Such an approach could find profound relevance within climates punctuated by dramatic temperature oscillations.
However, the study does acknowledge certain limitations that merit acknowledgment. Primarily, the scope of the research is tethered to a specific building and a particular set of weather conditions. Extrapolation to dissimilar building typologies and climates necessitates prudence. Furthermore, the study predominantly orbits around short-term energy implications, warranting the inclusion of long-term assessments encompassing roof durability, maintenance outlays, and holistic building performance within subsequent investigations.
In summation, this empirical exploration deftly probes into the energy efficacy of disparate roof typologies across an array of meteorological milieus. The outcomes distinctly underscore the intricate dance between roof characteristics and climatic dynamics, underscoring the indispensability of bespoke roofing solutions that harmonize with regional climatic vagaries. Embedded within the contours of this study is a seminal contribution to the compendium of knowledge tethered to building energy efficiency. The insights gleaned reverberate with implications, embellishing sustainable building design and enriching the landscape of urban planning.
The building envelope plays a critical role in determining energy consumption in buildings, and the roof, due to its direct interaction with solar radiation and heat exchange with the environment, holds a prominent position. This study focused on cool roofs, green roofs, and their heat transfer mechanisms, conducted using a roof performance testing infrastructure in Nanjing. The primary objective was to assess the energy-saving characteristics of these roof types in both summer and winter conditions, leading to the following key findings.
1) In summer, the cool roof exhibited the lowest energy consumption, followed by the green roof, while the conventional roof had the highest energy consumption. As solar radiation increased, the energy-saving effect of the cool roof became more pronounced. Between 9:00 a.m. and 6:00 p.m. in summer, the cool roof saved 11.7% more energy than the green roof and 4.1% more energy than the conventional roof. After calculations, the cool roof saved 13.2% more energy than the conventional roof.
2) In winter, the green roof had the lowest energy consumption, followed by the conventional roof, with the cool roof having the highest energy consumption. Between 9:00 a.m. and 6:00 p.m. in winter, the green roof saved 7.8% more energy than the cool roof and 4.9% more energy than the conventional roof. After adjustments, the cool roof increased energy consumption by 2.8% compared to the conventional roof.
3) The study revealed that while cool roofs provided clear energy savings during summer cooling, they could lead to increased heating loads during winter heating, especially in regions where cooling loads significantly outweigh heating loads in summer. This suggests the potential for substantial energy savings with cool roofs. Future research should explore their impact on overall building energy consumption throughout the year, considering both their positive effects in summer and negative effects in winter, to better assess the practical application of cool roofs and green roof technologies.
Furthermore, the methodological rigor of this study was evident, as it combined indoor experimentation with real-time monitoring of outdoor meteorological conditions. This approach ensured reliable and valid results by measuring parameters including surface temperatures, energy consumption, solar radiation, and ambient temperatures, providing a comprehensive view of how different roof types respond to varying climatic conditions.
The raw data supporting the conclusions of this article will be made available by the authors, without undue reservation.
SZ: Writing–original draft, Data curation, Investigation. GH. HM, Formal Analysis, Supervision, Writing–review and editing. XZ: Writing–review and editing.
This work is supported by the Jiangsu Maritime Institute Doctoral Research Initiation Fund (2023BSKY04) and the Natural Science Foundation of the Jiangsu Higher Education Institu-tions of China (23KJB470005).
The authors declare that the research was conducted in the absence of any commercial or financial relationships that could be construed as a potential conflict of interest.
The review editor XJ declared a shared affiliation with author XZ at the time of the review.
All claims expressed in this article are solely those of the authors and do not necessarily represent those of their affiliated organizations, or those of the publisher, the editors and the reviewers. Any product that may be evaluated in this article, or claim that may be made by its manufacturer, is not guaranteed or endorsed by the publisher.
Ayadi, O., and Al-Dahidi, S. (2019). Comparison of solar thermal and solar electric space heating and cooling systems for buildings in different climatic regions. Sol. Energy 188, 545–560. doi:10.1016/j.solener.2019.06.033
Bódis, K., Kougias, I., Jäger-Waldau, A., Taylor, N., and Szabó, S. (2019). A high-resolution geospatial assessment of the rooftop solar photovoltaic potential in the European Union. Renew. Sustain. Energy Rev. 114, 109309. doi:10.1016/j.rser.2019.109309
Cascone, S. (2019). Green roof design: state of the art on technology and materials. Sustainability 11, 3020. doi:10.3390/su11113020
Chaianong, A., and Pharino, C. (2015). Outlook and challenges for promoting solar photovoltaic rooftops in Thailand. Renew. Sustain. Energy Rev. 48, 356–372. doi:10.1016/j.rser.2015.04.042
Evangelisti, L., De Lieto Vollaro, R., and Asdrubali, F. (2019). Latest advances on solar thermal collectors: A comprehensive review. Renew. Sustain. Energy Rev. 114, 109318. doi:10.1016/j.rser.2019.109318
Gagliano, A., Patania, F., Nocera, F., Ferlito, A., and Galesi, A. (2012). Thermal performance of ventilated roofs during summer period. Energy Build. 49, 611–618. doi:10.1016/j.enbuild.2012.03.007
Gassar, A. A. A., and Cha, S. H. (2021). Review of geographic information systems-based rooftop solar photovoltaic potential estimation approaches at urban scales. Appl. Energy 291, 116817. doi:10.1016/j.apenergy.2021.116817
Hernández-Pérez, I., Álvarez, G., Xamán, J., Zavala-Guillén, I., Arce, J., and Simá, E. (2014). Thermal performance of reflective materials applied to exterior building components—a review. Energy Build. 80, 81–105. doi:10.1016/j.enbuild.2014.05.008
Hernández-Pérez, I., Xamán, J., Macías-Melo, E. V., Aguilar-Castro, K. M., Zavala-Guillén, I., Hernández-López, I., et al. (2018). Experimental thermal evaluation of building roofs with conventional and reflective coatings. Energy Build. 158, 569–579. doi:10.1016/j.enbuild.2017.09.085
Hou, M., Kong, X., Li, H., Yang, H., and Chen, W. (2021). Experimental study on the thermal performance of composite phase change ventilated roof. J. Energy Storage 33, 102060. doi:10.1016/j.est.2020.102060
Huang, S., Yu, H., Zhang, M., Qu, H., Wang, L., Zhang, C., et al. (2023). Advances, challenges and outlooks in frost-free air-source heat pumps: A comprehensive review from materials, components to systems. Appl. Therm. Eng. 234, 121163. doi:10.1016/j.applthermaleng.2023.121163
Ingebretsen, S. B., Andenæs, E., and Kvande, T. (2022). Microclimate of air cavities in ventilated roof and façade systems in nordic climates. Buildings 12, 683. doi:10.3390/buildings12050683
Jo, J. H., Carlson, J. D., Golden, J. S., and Bryan, H. (2010). An integrated empirical and modeling methodology for analyzing solar reflective roof technologies on commercial buildings. Build. Environ. 45, 453–460. doi:10.1016/j.buildenv.2009.07.001
Kain, G., Idam, F., Federspiel, F., Réh, R., and Krišťák, L. (2020). Suitability of wooden shingles for ventilated roofs: an evaluation of ventilation efficiency. Appl. Sci. 10, 6499. doi:10.3390/app10186499
Levinson, R., Berdahl, P., and Akbari, H. (2005a). Solar spectral optical properties of pigments—Part I: model for deriving scattering and absorption coefficients from transmittance and reflectance measurements. Sol. Energy Mater. Sol. Cells 89, 319–349. doi:10.1016/j.solmat.2004.11.012
Levinson, R., Berdahl, P., and Akbari, H. (2005b). Solar spectral optical properties of pigments—Part II: survey of common colorants. Sol. Energy Mater. Sol. Cells 89, 351–389. doi:10.1016/j.solmat.2004.11.013
Manso, M., Teotónio, I., Silva, C. M., and Cruz, C. O. (2021). Green roof and green wall benefits and costs: A review of the quantitative evidence. Renew. Sustain. Energy Rev. 135, 110111. doi:10.1016/j.rser.2020.110111
Olu-Ajayi, R., Alaka, H., Sulaimon, I., Sunmola, F., and Ajayi, S. (2022). Building energy consumption prediction for residential buildings using deep learning and other machine learning techniques. J. Build. Eng. 45, 103406. doi:10.1016/j.jobe.2021.103406
Florida Solar Energy Center Parker, D. (1997). Demonstration of cooling savings of light colored roof surfacing in Florida commercial buildings: Retail strip mall. FSEC Energy Research Center®.
Parker, D. S., and Barkaszi, S. F. (1997). Roof solar reflectance and cooling energy use: field research results from Florida. Energy Build. 25, 105–115. doi:10.1016/S0378-7788(96)01000-6
Pisello, A. L., and Cotana, F. (2014). The thermal effect of an innovative cool roof on residential buildings in Italy: results from two years of continuous monitoring. Energy Build. 69, 154–164. doi:10.1016/j.enbuild.2013.10.031
Rawat, M., and Singh, R. N. (2022). A study on the comparative review of cool roof thermal performance in various regions. Energy Built Environ. 3, 327–347. doi:10.1016/j.enbenv.2021.03.001
Shafique, M., Kim, R., and Rafiq, M. (2018). Green roof benefits, opportunities and challenges – a review. Renew. Sustain. Energy Rev. 90, 757–773. doi:10.1016/j.rser.2018.04.006
Tian, D., Zhang, J., and Gao, Z. (2023). The advancement of research in cool roof: super cool roof, temperature-adaptive roof and crucial issues of application in cities. Energy Build. 291, 113131. doi:10.1016/j.enbuild.2023.113131
Wang, R. Z., and Zhai, X. Q. (2010). Development of solar thermal technologies in China. Energy, Energy Its Sustain. Dev. China 35, 4407–4416. doi:10.1016/j.energy.2009.04.005
Wu, Y., Zhao, H., Sun, H., Duan, M., Lin, B., and Wu, S. (2022). A review of the application of radiative sky cooling in buildings: challenges and optimization. Energy Convers. Manag. 265, 115768. doi:10.1016/j.enconman.2022.115768
Xu, G., and Wang, W. (2020). China’s energy consumption in construction and building sectors: an outlook to 2100. Energy 195, 117045. doi:10.1016/j.energy.2020.117045
Zhang, K., Zhao, D., Yin, X., Yang, R., and Tan, G. (2018). Energy saving and economic analysis of a new hybrid radiative cooling system for single-family houses in the USA. Appl. Energy 224, 371–381. doi:10.1016/j.apenergy.2018.04.115
Zhao, D., Aili, A., Zhai, Y., Xu, S., Tan, G., Yin, X., et al. (2019). Radiative sky cooling: fundamental principles, materials, and applications. Appl. Phys. Rev. 6, 021306. doi:10.1063/1.5087281
Keywords: building energy conservation, green roof, cool roof, time difference comparison, experimental analysis
Citation: Zhao S, Hai G, Ma H and Zhang X (2023) Experimental analysis of energy consumption of building roof energy-saving technologies based on time difference comparison test. Front. Energy Res. 11:1291213. doi: 10.3389/fenrg.2023.1291213
Received: 08 September 2023; Accepted: 25 September 2023;
Published: 06 October 2023.
Edited by:
Xiaolei Yuan, Aalto University, FinlandReviewed by:
Xing Jin, Southeast University, ChinaCopyright © 2023 Zhao, Hai, Ma and Zhang. This is an open-access article distributed under the terms of the Creative Commons Attribution License (CC BY). The use, distribution or reproduction in other forums is permitted, provided the original author(s) and the copyright owner(s) are credited and that the original publication in this journal is cited, in accordance with accepted academic practice. No use, distribution or reproduction is permitted which does not comply with these terms.
*Correspondence: Shanguo Zhao, YnJvemhhb0AxMjYuY29t
Disclaimer: All claims expressed in this article are solely those of the authors and do not necessarily represent those of their affiliated organizations, or those of the publisher, the editors and the reviewers. Any product that may be evaluated in this article or claim that may be made by its manufacturer is not guaranteed or endorsed by the publisher.
Research integrity at Frontiers
Learn more about the work of our research integrity team to safeguard the quality of each article we publish.