- 1Department of Chemical Engineering, College of Chemical and Materials, King Fahd University of Petroleum and Minerals, Dhahran, Saudi Arabia
- 2Bavarian Center for Battery Technology (BayBatt), Department of Chemistry, University of Bayreuth, Bayreuth, Germany
- 3School of Integrative Engineering, Chung-Ang University, Seoul, Republic of Korea
- 4Department of Molecular Science and Technology, Ajou University, Suwon, Republic of Korea
Graphitic carbon nitride (g-C3N4) has emerged as a promising material for various applications, particularly in the field of energy storage systems. Among these systems, lithium-ion batteries (LIBs) have become the cornerstone of portable electronics and are increasingly being adopted for electric vehicles and renewable energy storage. However, the search for alternative electrode materials that can overcome the limitations of traditional graphite anodes and transition metal oxide cathodes remains a significant challenge. In recent years, g-C3N4 has attracted considerable attention due to its unique physicochemical properties, such as high electrochemical stability, tunable bandgap, large specific surface area, and excellent thermal and chemical stability. Also, the low cost, abundance, and environmental sustainability of g-C3N4 contribute to its suitability for next-generation LIBs. However, the successful utilization of g-C3N4 as an electrode material is hindered by several challenges. This paper aims to explore the challenges and future perspectives of utilizing g-C3N4 as a potential electrode material for LIBs, highlighting the potential benefits and drawbacks of integrating this material into the battery system.
1 Introduction
The Earth has an abundance of energy resources in the form of fossil fuels, most of which are being consumed at an exponential rate leading to rapid depletion of them. Despite, the depletion of fossil fuels, the energy requirement of the world will double by 2050 (Organization for Economic Co-operation and Development, 1999; International Energy Agency, 2050). Secondly, fossil fuels release greenhouse gases such as CO2 and contribute to global warming. To mitigate these problems, scientists have been working on renewable and sustainable energy resources, such as solar energy, wind energy, tidal energy, etc., for a long time (Gong et al., 2015; Shamoon et al., 2022; Greening et al., 2023). Researchers developed many ways to utilize and store these energies in the form of electrical energy (Miller et al., 2015; Baig et al., 2022). The most powerful tool to store electrical energy is electrochemical devices such as batteries (Boruah et al., 2020), in which electrical energy can be stored in the chemical form and can be used directly from batteries when needed (Escudero-González & Amparo López-Jiménez, 2014; Fan et al., 2020). Thus, scientists have started to design and build high-performance rechargeable batteries (Yuan et al., 2022). Among the rechargeable batteries, LIBs have many advantages over other batteries. For example, LIBs are—1) more efficient and smaller in size as compared to other batteries, 2) very good in charge and discharge repeating cycle, 3) able to favor cordless charging, and 4) competent to prevent self-discharge (Liu et al., 2015; Zhou et al., 2018). LIBs possess good energy storage systems and have been extensively employed in many electric vehicles, transferable electronic gadgets, and electrical energy storage stations. Consequently, LIBs attracted huge marketable feat requiring more advancement such as high safety with high energy density capacity, low cost, and good reliability (Hu et al., 2017; Lu et al., 2019).
The main components of LIBs are a cathode (like—LiCoO2), an anode (like—graphite), and an electrolyte (Encyclopedia of Sustainability Science and Technology, 2012). Cathode in LIBs behaves as a source of the Li-ions, once these ions pass through the electrolyte and accumulate in between the stacking layers of the anode, this phenomenon is called recharging of LIBs (Figure 1D). The LIBs follow the vice versa phenomenon of charging and discharging. In commercially available LIBs, graphite is widely acceptable as an anode as it has indispensable merits of high numbers of charging and discharging cycles, easy availability, good thermal and chemical stability, short voltage plateau, excellent chemical kinetics, and low cost (Safaei et al., 2018). In contrast, graphite anode has a low energy density rate (372 mAh g−1) which does not equally align with the recently unveiled cathodes’ energy density rate as these cathodes have a comparatively high rate (Fu et al., 2014). Besides, the propagation of lithium dendrite over the graphite anode is also a matter of acute safety concerns, such as catching fire (Lu et al., 2019). These shortcomings collectively restrict its application in high-performance LIBs. Subsequently, immense research has been done to mitigate these problems, and researchers designed and synthesized numerous materials to be used as an anode. However, the search for alternative electrode materials that can overcome the limitations of traditional graphite anodes and transition metal oxide cathodes remains a significant challenge (Liang et al., 2021). Among them, the two-dimensional graphite with nitrogen substitution at its maximum doping level provides a unique material called graphitic carbon nitride (g-C3N4) as a highly potential electrode material. Nitrogen doping of graphite provides a high coulombic cycle storage and reversible capacity. That is why, g-C3N4 has emerged as a promising material for various applications, particularly in the field of energy storage systems (Zhang H. et al., 2020; Wang et al., 2021; Tang et al., 2023). Also, this two-dimensional carbon-based material (g-C3N4) in comparison with graphite exhibits high oxygen-reducing reactivity, remarkable chemical stability, and high thermal stability. Furthermore, g-C3N4 has a tunable bandgap, high surface area, and excellent stability (Luo et al., 2019). These features all together make it an ideal candidate for batteries. In terms of metal-ion batteries (MIBs) like LIBs, g-C3N4 composites have shown superiority to some extent over other electrode materials (like: graphite, silicone, TiO2) as demonstrated by Yadav et al., 2023, Maniyazagan et al., 2023, Nulu et al., 2022; Hankel et al., 2015, because its structure is based on the poly (triazine imide) webs. These webs contain pores to facilitate easy coordination with Li-ions (Zhao et al., 2015).
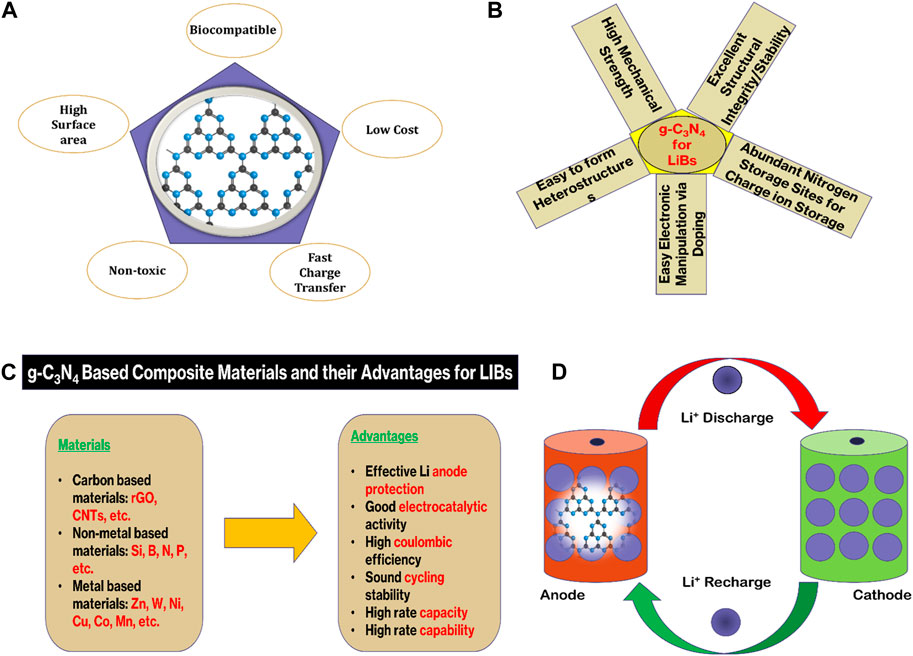
FIGURE 1. (A) g-C3N4 structure and its properties, (B,C) Advantages and physicochemical properties of g-C3N4 composites for LIBs, and (D) Charging and discharging of a g-C3N4-based LIB.
Instead of having of edge over other electrode materials, several challenges need to be addressed to realize the full potential of g-C3N4-based electrodes for LIBs. Among them, some of the major challenges compared to other electrodes are limited electrical conductivity, restricted cycling stability, and poor kinetics of LIBs (Gordon et al., 2023). Besides, large-scale production of g-C3N4 with cost-effective methods is still a quest. There is significant literature on the application of g-C3N4 in energy storage systems in the last decade, however, this manuscript is solely focused on the utilization of g-C3N4 as electrode material for LIBs. Also, this review article aims to explore those challenges and future perspectives of utilizing g-C3N4 as an electrode for LIBs, highlighting the potential benefits and drawbacks of integrating this material into the battery system. By addressing the key challenges and discussing the potential prospects, this analysis will shed light on the feasibility and relevance of g-C3N4-based LIBs in the quest for more efficient and sustainable energy storage solutions.
2 Physicochemical properties of g-C3N4
The physicochemical properties of g-C3N4 make it an attractive alternative to conventional electrode materials as shown in Figure 1. These physicochemical properties are chiefly influenced by the distinctive sheet structure of g-C3N4. The sheets of g-C3N4 are supposed to be made up of two primary unit rings—1) s-triazine (C3N4), and 2) tri-s-triazine (C6N7).
The s-triazine units are not as energetically favorable as the tri-s-triazine units (Cao et al., 2020). It was determined that the density functional theory (DFT) favors the thermodynamic stability of tri-s-triazine units over the s-triazine units (Kroke et al., 2002). Hence, it is broadly admitted that the tri-s-triazine units are the main constructing blocks of g-C3N4 sheets. Here, the key physicochemical properties of g-C3N4 that contribute to its suitability for LIBs have been conferred.
2.1 Thermal stability
The thermal stability of electrode materials is crucial to ensure reliable and safe operation. It is vital to develop such LIBs which can be operated at elevated temperatures for long-lastingEVs operation. For this purpose, g-C3N4 exhibits excellent thermal stability, with a decomposition temperature of around 600 °C (Liang et al., 2021). The high thermal stability of g-C3N4 ensures that it remains structurally intact and minimizes the risk of thermal runaway in LIBs (X. Liu et al., 2023). Thus g-C3N4 exhibits a paramount potential for thermally stable LIBs. However, there is a need of further research on commercialization of stable LIBs employing g-C3N4 as an electrode for elevated temperature environments as in EVs (Chen et al., 2019).
2.2 Electrochemical stability
g-C3N4 shows its structural integrity and performance over repeated charge-discharge cycles, exibhiting electrochemical stability. In, electrode materials undergo significant volume changes during cycling, which leads to mechanical degradation and capacity loss (Luo et al., 2019). However, plasma-induced highly nitrogen-deficient (ND) g-C3N4 electrode possesses a reasonably stable chemical structure resulting in retention of its performance even after 5,000 cycles (Sun et al., 2022). The remarkable chemical stability makes g-C3N4 a suitable candidate for long-lasting and high-capacity LIBs (Niu and Yang, 2018; Zhang J. et al., 2023).
2.3 Electrical conductivity
Pure g-C3N4 is a semiconductor with limited electrical conductivity. However, by incorporating dopants or adopting new synthesis methods, the conductivity of g-C3N4 can be significantly improved (Zhang X. et al., 2020). For instance, doping g-C3N4 with elements such as carbon or sulfur has been shown to enhance its conductivity, resulting in improved Li-ion battery performance (Hong et al., 2020). Likewise, recently developed technologies such as nitrogen-deficient g-C3N4 have a great potential for high-conductivity electrodes (Sun et al., 2022). The superior electrical conductivity will help the rapid and large-scale adoption of g-C3N4 in LIBs.
2.4 Specific surface area
The g-C3N4 exhibits a large surface area due to its unique porous structure (Huang et al., 2020), which consists of stacked layers with interlayer spacing that can accommodate a high density of lithium ions. The high surface area of g-C3N4 enhances the accessibility of lithium ions to the electrode material, facilitating faster charge-discharge processes and higher energy storage capacity (J. Zhang et al., 2015). The high surface areas also favor g-C3N4 as a highly potential electrode candidate for LIBs.
2.5 Sustainability and chemical stability
The carbon and nitrogen atoms in g-C3N4 form strong covalent bonds, which render the material resistant to chemical degradation. This stability ensures that the g-C3N4 electrode withstands solid-electrolyte-interphase leading to a prolonged lifespan of the electrode material, reducing the need for frequent replacements and lowering the environmental impact (Li et al., 2023). Additionally, g-C3N4 is composed of abundant elements, making it an attractive alternative to the limited and expensive resources used in traditional LIB materials, such as cobalt (Kong et al., 2018).
3 Application of g-C3N4 in LIBs
Especially to be used as an anode or cathode the composite structures of g-C3N4 can also be formed by combining with other materials (Rono et al., 2021), leveraging the advantages of both materials. Here are some specific applications of g-C3N4 in LIBs as an anode and cathode.
3.1 Auxiliary anode material
The pristine form of g-C3N4 has shown a higher theoretical capacity compared to graphite, with a capacity of around 524 mAh g−1 (Adekoya et al., 2021). The g-C3N4 unique electronic properties and porous structure allow for improved lithium-ion diffusion and enhanced cycling stability (Chen et al., 2017; Li et al., 2020). Therefore, as an anode, g-C3N4 composites of graphene (Wang et al., 2018), Nitrogen and Phosphorous (Tao et al., 2017), and salt of Se (Zhou et al., 2018), Zn (Joshi et al., 2018), Mn/Ni/Cu/Co (Zhang et al., 2019), Sn (Maniyazagan et al., 2023), WS2 (Xu et al., 2022) exhibit improved specific capacities. While, 2,749 mAh g−1 is the highest initial discharge capacity so far reported for Sn/g-C3N4 composite anode (Le et al., 2022). So, it is deduced that g-C3N4 can be utilized as a composite with other materials to enhance its properties and performance.
3.2 Auxiliary cathode material
The g-C3N4 can also be employed as a cathode material in LIBs. It can serve as a host matrix for active materials, improving their electrochemical performance (Dutta et al., 2022). By incorporating active materials into the g-C3N4 matrix, the overall capacity and cycling stability of the cathode can be enhanced (Ramar and Wang, 2022). Similarly, the composites of g-C3N4 with graphene (Huang et al., 2016), porous carbon (Hong et al., 2020), nano sulfur copolymer (Yao et al., 2018; Tiwari et al., 2022), and salt of P (Zhang H. et al., 2020), and Cu (Li et al., 2023), show incredibly good cathodic properties. Hence, g-C3N4 also shows the characteristics of it being employed as a single electrode material or as support for other materials rendering them superior performance.
4 Challenges and future directions of g-C3N4 for LIBs
Despite the excellent merits of g-C3N4 (Ahmed and Maraz, 2023), as an electrode, it is facing many challenges to be used as a strong electrode material in LIBs, which are discussed in this section.
4.1 Challenges in utilizing g-C3N4 for LIBs
4.1.1 Limited electrical conductivity
One of the primary challenges associated with g-C3N4 as an electrode material for LIBs is its limited electrical conductivity (Ruby Raj et al., 2023). Compared to conventional carbon-based materials, such as graphite, g-C3N4 exhibits lower electron transport properties. This impedes the efficient movement of charge carriers during charge and discharge processes, leading to reduced battery performance (Luo et al., 2019). There have been efforts to improve the electrical conductivity of g-C3N4 by doping with other materials. However, enhancing the electrical conductivity of g-C3N4 without compromising its unique properties is still a critical challenge that needs to be addressed.
4.1.2 Poor kinetics of Li-ion intercalation
The inherently layered structure of graphite allows for easy intercalation of Li-ions, which enables the reversible charge storage mechanism in LIBs (Zhu et al., 2019). In the case of g-C3N4, however, the intercalation kinetics of Li-ions is slower (Li et al., 2022). This sluggish intercalation limits the battery’s rate capability and affects charge-discharge performance (S. Wang et al., 2018). Developing strategies to improve the kinetics of li-ion intercalation within g-C3N4 is imperative for its successful integration into high-performance LIBs (Pathak et al., 2021).
4.1.3 Limited cycling stability
The g-C3N4-based electrodes demonstrate poor cycling stability, it is because they may experience mechanical stress, leading to structural degradation (Versaci et al., 2020). Li-ion insertion and extraction cause pulverization of the electrode. Further, slow kinetics of li-ion diffusion, unwanted side reactions between the electrode and the electrolyte leading to the formation of solid-electrolyte interface (SEI), and g-C3N4 may trap some lithium ions during cycling, reducing the reversible capacity of the electrode over time. These all phenomena contribute to the loss of cycling stability. Exploring novel approaches to mitigate structural deterioration and enhance the cycling stability of g-C3N4 electrodes is crucial for their industrial implementation as Sun et al., did, they built an anode material for LIBs by refilling of heteroatom in plasma-induced highly ND g-C3N4 (Sun et al., 2022).
4.1.4 Scalability and cost-effectiveness
For any promising material to find widespread application in LIBs, scalability and cost-effectiveness are key considerations (Xia et al., 2022; Liu et al., 2023). Currently, the synthesis of g-C3N4 lacks appropriate methods for large-scale production (Wang et al., 2019). Additionally, the cost of raw materials used for g-C3N4 synthesis is higher compared to conventional carbonaceous materials (Fang et al., 2016; Zou et al., 2016). Addressing these challenges is vital to ensure the viability and commercialization of g-C3N4-based LIBs.
4.2 Future directions
4.2.1 Designing hierarchical structures
The development of hierarchical structures can address the limited electrical conductivity of g-C3N4 (Xu et al., 2022). Introducing conductive additives or forming composite structures with conducting materials can improve the overall conductivity of the electrode. For example, incorporating carbon nanotubes or graphene into g-C3N4 matrices can enhance its electrical properties, leading to improved li-ion diffusion and charge transport within the electrode (Wang S. et al., 2023; Wang Y. et al., 2023).
4.2.2 Surface modification of g-C3N4
Surface modification strategies can be employed to enhance the kinetics of li-ion intercalation in g-C3N4-based electrodes. Functionalizing the g-C3N4 surface with active groups can facilitate li-ion diffusion and enhance the interaction between the electrode and electrolyte (J. Li et al., 2023). By careful selection and design of functional groups, improved intercalation kinetics and enhanced battery performance can be achieved (Choudhury et al., 2023).
4.2.3 Structural stability improvement
To improve the cycling stability of g-C3N4 electrodes, efforts must focus on stabilizing their structure during repeated lithiation and delithiation cycles (Li et al., 2017; Veith et al., 2013). Incorporating strengthening agents, such as carbon fibers or polymers, can provide mechanical support and alleviate structural distortion. Furthermore, exploring surface coatings and protective layers can prevent unwanted side reactions, reducing capacity fade, and improving long-term stability as reported by Maniyazagan et al.
4.2.4 Scalable synthesis methods
Developing scalable synthesis methods for g-C3N4 electrodes will facilitate their large-scale production. Innovative approaches like solvothermal, aerosol-assisted, or direct carbonization of nitrogen-containing precursors (Wang et al., 2020) can be explored (Villalobos et al., 2020). These techniques can promote efficient mass production of g-C3N4 electrodes, making them more commercially viable (Ramar and Wang, 2022).
4.2.5 Cost reduction
To enhance the cost-effectiveness of g-C3N4-based LIBs, efforts should be directed toward finding alternative and cheaper precursors for g-C3N4 synthesis (Han et al., 2019), which can be sustainably recycled or disposed off from used LIBs (Y. Liu et al., 2021). Moreover, innovative manufacturing techniques and optimization of fabrication processes can contribute to cost reduction for commercial production of LIBs with g-C3N4 (Zhang Y. et al., 2023; Degen and Krätzig, 2023).
5 Conclusion
In conclusion, the g-C3N4 exhibits various physicochemical properties that make it a promising material for LIBs. Its high thermal stability, large specific surface area, chemical stability, and environmental sustainability contribute to its suitability for LIBs, as discovered recently. These exceptional properties of g-C3N4 make it a favorable material for diverse functions in LIBs, including anodes, cathodes, composites, electrolyte additives, and separator coatings. Also, its incorporation in LIBs can lead to improved energy storage performance, enhanced cycling stability, and increased safety. Despite the challenges faced by g-C3N4 for LIBs, concerted efforts in addressing the limited electrical conductivity, poor intercalation kinetics, scalability, and cost-effectiveness can pave the way for its successful integration. Additionally, continued research and development in synthesizing and optimizing g-C3N4-based electrodes for high-performance and environment-friendly LIBs can contribute to a more sustainable and efficient energy storage future. g-C3N4 can emerge as a competitive material for next-generation LIBs, by designing hierarchical structures, surface modification, improving structural stability, developing scalable synthesis methods, and reducing production costs.
Data availability statement
The original contributions presented in the study are included in the article/Supplementary material, further inquiries can be directed to the corresponding authors.
Author contributions
MS: Investigation, Writing–original draft, Writing–review and editing, Data curation, Validation. ZC: Investigation, Validation, Writing–review and editing. BH: Investigation, Writing–review and editing, Project administration, Supervision. TY: Investigation, Project administration, Supervision, Writing–review and editing. JC: Investigation, Project administration, Supervision, Writing–review and editing, Conceptualization, Funding acquisition, Resources, Visualization, Writing–original draft.
Funding
The author(s) declare financial support was received for the research, authorship, and/or publication of this article. This work was supported by funding from Bavarian Center for Battery Technology (BayBatt), Bayerisch-Tschechische Hochschulagentur (BTHA) (BTHA-AP-2022-45, BTHA-AP-2023-5, BTHA-AP-2023-12, and BTHA-AP-2023-38). This work was also supported by the University of Bayreuth-Deakin University Joint Ph.D. Program, Bayerische Forschungallianz (BayFOR) (BayIntAn_UBT_2023_84), BK21 program from National Research Foundation of Korea, Erasmus+ program from the European Union, Ministry of Education, Science and Technology as part of the Higher Education for Economic Transformation (HEET) Project (World Bank), and collaboration project funding from Kangwon National University and LINC 3.0 Research Center.
Acknowledgments
MS is highly obliged to JC (University of Bayreuth, Germany) for his supervision from the conceptualization to the completion of this article. MS is also profoundly thankful to Dr. Wasim Ullah Khan (KFUPM, Saudi Arabia) for his mentorship throughout the drafting of this article. In addition, services provided by the King Fahd University of Petroleum and Minerals, Kingdom of Saudi Arabia, and the University of Bayreuth are greatly regarded.
Conflict of interest
The authors declare that the research was conducted in the absence of any commercial or financial relationships that could be construed as a potential conflict of interest.
Publisher’s note
All claims expressed in this article are solely those of the authors and do not necessarily represent those of their affiliated organizations, or those of the publisher, the editors and the reviewers. Any product that may be evaluated in this article, or claim that may be made by its manufacturer, is not guaranteed or endorsed by the publisher.
References
Adekoya, D., Qian, S., Gu, X., Wen, W., Li, D., Ma, J., et al. (2021). DFT-guided design and fabrication of carbon-nitride-based materials for energy storage devices: a review. Nano-Micro Lett. 13 (1), 13. doi:10.1007/s40820-020-00522-1
Ahmed, Md. D., and Maraz, K. M. (2023). Revolutionizing energy storage: overcoming challenges and unleashing the potential of next generation Lithium-ion battery technology. Mater. Eng. Res. 5 (1), 265–278. doi:10.25082/MER.2023.01.003
Baig, U., Gondal, M. A., Dastageer, M. A., and Sajid, M. (2022). Maghemite nanoparticles decorated semiconducting graphitic carbon nitride hetero-structured nanocomposite: facile synthesis, characterizations and its visible light active photocatalytic system for removal of hazardous organic pollutants from aqueous solutions. Colloids Surfaces A Physicochem. Eng. Aspects 641, 128427. doi:10.1016/j.colsurfa.2022.128427
Boruah, B. D., Mathieson, A., Wen, B., Jo, C., Deschler, F., and De Volder, M. (2020). Photo-rechargeable zinc-ion capacitor using 2D graphitic carbon nitride. Nano Lett. 20 (8), 5967–5974. doi:10.1021/acs.nanolett.0c01958
Cao, Q., Kumru, B., Antonietti, M., and Schmidt, B. V. K. J. (2020). Graphitic carbon nitride and polymers: a mutual combination for advanced properties. Mater. Horizons 7 (Issue 3), 762–786. doi:10.1039/c9mh01497g
Chen, J., Mao, Z., Zhang, L., Wang, D., Xu, R., Bie, L., et al. (2017). Nitrogen-deficient graphitic carbon nitride with enhanced performance for lithium ion battery anodes. ACS Nano 11 (12), 12650–12657. doi:10.1021/acsnano.7b07116
Chen, W., Liang, J., Yang, Z., and Li, G. (2019). A review of lithium-ion battery for electric vehicle applications and beyond. Energy Procedia 158, 4363–4368. doi:10.1016/j.egypro.2019.01.783
Choudhury, R., Kurra, N., and Meduri, P. (2023). Doped micro-silicon and vanadium carbide MXene composite as anode for high stability and high capacity Li-ion batteries. Results Eng. 19, 101338. doi:10.1016/j.rineng.2023.101338
Degen, F., and Krätzig, O. (2023). Modeling large-scale manufacturing of lithium-ion battery cells: impact of new technologies on production economics. IEEE Trans. Eng. Manag. 2023, 1–17. doi:10.1109/TEM.2023.3264294
Dutta, D. P., Pathak, D. D., Abraham, S., and Ravuri, B. R. (2022). An insight into the sodium-ion and lithium-ion storage properties of CuS/graphitic carbon nitride nanocomposite. RSC Adv. 12 (20), 12383–12395. doi:10.1039/d2ra02014a
Encyclopedia of Sustainability Science and Technology (2012). Encyclopedia of sustainability science and Technology. New York: Springer. doi:10.1007/978-1-4419-0851-3
Escudero-González, J., and Amparo López-Jiménez, P. (2014). Iron redox battery as electrical energy storage system in the Spanish energetic framework. Int. J. Electr. Power Energy Syst. 61, 421–428. doi:10.1016/j.ijepes.2014.03.067
Fan, X., Liu, B., Liu, J., Ding, J., Han, X., Deng, Y., et al. (2020). Battery technologies for grid-level large-scale electrical energy storage. Trans. Tianjin Univ. 26 (Issue 2), 92–103. doi:10.1007/s12209-019-00231-w
Fang, H.-B., Luo, Y., Zheng, Y.-Z., Ma, W., and Tao, X. (2016). Facile large-scale synthesis of urea-derived porous graphitic carbon nitride with extraordinary visible-light spectrum photodegradation. Industrial Eng. Chem. Res. 55 (16), 4506–4514. doi:10.1021/acs.iecr.6b00041
Fu, Y., Zhu, J., Hu, C., Wu, X., and Wang, X. (2014). Covalently coupled hybrid of graphitic carbon nitride with reduced graphene oxide as a superior performance lithium-ion battery anode. Nanoscale 6 (21), 12555–12564. doi:10.1039/c4nr03145h
Gong, Y., Li, M., and Wang, Y. (2015). Carbon nitride in energy conversion and storage: recent advances and future prospects. ChemSusChem 8 (Issue 6), 931–946. doi:10.1002/cssc.201403287
Gordon, I. P., Xu, W., Randak, S., Jow, T. R., and Stadie, N. P. (2023). Stabilizing effects of phosphorus-doped silicon nanoparticle anodes in lithium-ion batteries. Chem. Mater. 35 (2), 549–557. doi:10.1021/acs.chemmater.2c02983
Greening, B., Braunholtz-Speight, T., Wood, R., and Freer, M. (2023). Batteries and beyond: multi-vector energy storage as a tool to decarbonise energy services. Front. Energy Res. 10, 1109997. doi:10.3389/fenrg.2022.1109997
Han, D., Liu, J., Cai, H., Zhou, X., Kong, L., Wang, J., et al. (2019). High-yield and low-cost method to synthesize large-area porous g-C3N4 nanosheets with improved photocatalytic activity for gaseous nitric oxide and 2-propanol photodegradation. Appl. Surf. Sci. 464, 577–585. doi:10.1016/j.apsusc.2018.09.108
Hankel, M., Ye, D., Wang, L., and Searles, D. J. (2015). Lithium and sodium storage on graphitic carbon nitride. J. Phys. Chem. C 119 (38), 21921–21927. doi:10.1021/acs.jpcc.5b07572
Hong, X., Liu, Y., Fu, J., Wang, X., Zhang, T., Wang, S., et al. (2020). A wheat flour derived hierarchical porous carbon/graphitic carbon nitride composite for high-performance lithium–sulfur batteries. Carbon 170, 119–126. doi:10.1016/j.carbon.2020.08.032
Hu, J., Tian, J., and Li, C. (2017). Nanostructured carbon nitride polymer-reinforced electrolyte to enable dendrite-suppressed lithium metal batteries. ACS Appl. Mater. Interfaces 9 (13), 11615–11625. doi:10.1021/acsami.7b00478
Huang, Y., Chen, B., Duan, J., Yang, F., Wang, T., Wang, Z., et al. (2020). Graphitic carbon nitride (g-C3N4): an interface enabler for solid-state lithium metal batteries. Angew. Chem. - Int. Ed. 59 (9), 3699–3704. doi:10.1002/anie.201914417
Huang, Y., Tang, Y., Mai, Y., Wang, X., Wang, C., Han, S., et al. (2016). Three-dimensional carbon nitride/graphene framework as a high-performance cathode for lithium-ion batteries. Chem. Asian J. 11 (8), 1194–1198. doi:10.1002/asia.201501140
International Energy Agency (2050). Net zero by 2050 - a roadmap for the global energy sector. Avaliable at: www.iea.org/t&c/.
Joshi, B., Samuel, E., Kim, T.-G., Park, C.-W., Kim, Y.-I., Swihart, M. T., et al. (2018). Supersonically spray-coated zinc ferrite/graphitic-carbon nitride composite as a stable high-capacity anode material for lithium-ion batteries. J. Alloys Compd. 768, 525–534. doi:10.1016/j.jallcom.2018.07.027
Kong, L., Chen, Q., Shen, X., Xu, Z., Xu, C., Ji, Z., et al. (2018). MOF derived nitrogen-doped carbon polyhedrons decorated on graphitic carbon nitride sheets with enhanced electrochemical capacitive energy storage performance. Electrochimica Acta 265, 651–661. doi:10.1016/j.electacta.2018.01.146
Kroke, E., Schwarz, M., Horath-Bordon, E., Kroll, P., Noll, B., and Norman, A. D. (2002). Tri-s-triazine derivatives. Part I. From trichloro-tri-s-triazine to graphitic C3N4 structuresPart II: alkalicyamelurates M3[C6N7O3], M = Li, Na, K, Rb, Cs, manuscript in preparation. New J. Chem. 26 (5), 508–512. doi:10.1039/b111062b
Le, Q. D., Ngoc, P. N., Huu, H. T., Nguyen, T. H. T., Van, T. N., Thi, L. N., et al. (2022). A novel anode Sn/g-C3N4 composite for lithium-ion batteries. Chem. Phys. Lett. 796, 139550. doi:10.1016/j.cplett.2022.139550
Li, J., Ma, D., Lu, G., Fu, D., de León, C. P., and Pan, J. H. (2023). Correlations of precursor and carbon coating with electrochemical property of 2D graphitic carbon nitride (g-C 3 N 4) nanosheets as high-reversibility cathode of nonaqueous Al-ion batteries. J. Phys. Chem. C 127 (10), 4862–4871. doi:10.1021/acs.jpcc.3c00546
Li, P., Shen, Y., Li, X., Huang, W., and Lu, X. (2022). Fullerene-intercalated graphitic carbon nitride as a high-performance anode material for sodium-ion batteries. ENERGY & Environ. Mater. 5 (2), 608–616. doi:10.1002/eem2.12200
Li, X., Tan, T., Zhang, J., Zhang, N., He, J., Han, W., et al. (2020). Nitrogen deficient graphitic carbon nitride as anodes for lithium-ion batteries. J. Wuhan Univ. Technol. Mater. Sci. Ed. 35 (2), 263–271. doi:10.1007/s11595-020-2252-2
Li, Z., Wu, L., Wang, L., Gu, A., and Zhou, Q. (2017). Nickel cobalt sulfide nanosheets uniformly anchored on porous graphitic carbon nitride for supercapacitors with high cycling performance. Electrochimica Acta 231, 617–625. doi:10.1016/j.electacta.2017.02.087
Liang, Q., Shao, B., Tong, S., Liu, Z., Tang, L., Liu, Y., et al. (2021). Recent advances of melamine self-assembled graphitic carbon nitride-based materials: design, synthesis and application in energy and environment. Chem. Eng. J. 405, 126951. doi:10.1016/j.cej.2020.126951
Liu, J., Li, W., Duan, L., Li, X., Ji, L., Geng, Z., et al. (2015). A graphene-like oxygenated carbon nitride material for improved cycle-life lithium/sulfur batteries. Nano Lett. 15 (8), 5137–5142. doi:10.1021/acs.nanolett.5b01919
Liu, X., Xu, X., Gan, H., Yu, M., and Huang, Y. (2023). The effect of different g-C3N4 precursor nature on its structural control and photocatalytic degradation activity. Catalysts 13 (5), 848. doi:10.3390/catal13050848
Liu, Y., Zhang, R., Wang, J., and Wang, Y. (2021). Current and future lithium-ion battery manufacturing. IScience 24 (4), 102332. doi:10.1016/j.isci.2021.102332
Lu, Z., Liang, Q., Wang, B., Tao, Y., Zhao, Y., Lv, W., et al. (2019). Graphitic carbon nitride induced micro-electric field for dendrite-free lithium metal anodes. Adv. Energy Mater. 9 (7). doi:10.1002/aenm.201803186
Luo, Y., Yan, Y., Zheng, S., Xue, H., and Pang, H. (2019). Graphitic carbon nitride based materials for electrochemical energy storage. J. Mater. Chem. A 7 (Issue 3), 901–924. doi:10.1039/c8ta08464e
Maniyazagan, M., Zuhaib, H., Naveenkumar, P., Yang, H.-W., Kang, W. S., and Kim, S.-J. (2023). Flower-like SnS2/honeycomb-like g-C3N4 composite as an anode material for high-rate, long-term lithium-ion batteries. J. Energy Storage 68, 107894. doi:10.1016/j.est.2023.107894
Miller, T. S., Jorge, A. B., Sella, A., Corà, F., Shearing, P. R., Brett, D. J. L., et al. (2015). The use of graphitic carbon nitride based composite anodes for lithium-ion battery applications. Electroanalysis 27 (11), 2614–2619. doi:10.1002/elan.201500205
Niu, W., and Yang, Y. (2018). Graphitic carbon nitride for electrochemical energy conversion and storage. ACS Energy Lett. 3 (Issue 11), 2796–2815. doi:10.1021/acsenergylett.8b01594
Nulu, A., Hwang, Y. G., Nulu, V., and Sohn, K. Y. (2022). Metal (Cu/Fe/Mn)-Doped silicon/graphite composite as a cost-effective anode for Li-ion batteries. Nanomaterials 12 (17), 3004. doi:10.3390/nano12173004
Organisation for Economic Co-operation and Development (1999). Energy, the next fifty years. Paris, France: Organisation for Economic Co-operation and Development.
Pathak, D. D., Dutta, D. P., Ravuri, B. R., Ballal, A., Joshi, A. C., and Tyagi, A. K. (2021). An insight into the effect of g-C3N4 support on the enhanced performance of ZnS nanoparticles as anode material for lithium-ion and sodium-ion batteries. Electrochimica Acta 370, 137715. doi:10.1016/j.electacta.2021.137715
Ramar, A., and Wang, F.-M. (2022). “Graphitic carbon nitride for batteries,” in Nanoscale graphitic carbon nitride (Amsterdam, Netherlands: Elsevier), 367–392. doi:10.1016/B978-0-12-823034-3.00011-X
Rono, N., Kibet, J. K., Martincigh, B. S., and Nyamori, V. O. (2021). A review of the current status of graphitic carbon nitride. Crit. Rev. Solid State Mater. Sci. 46 (Issue 3), 189–217. doi:10.1080/10408436.2019.1709414
Ruby Raj, M., Yun, J., Son, D., and Lee, G. (2023). Extraordinary ultrahigh-capacity and long cycle life lithium-ion batteries enabled by graphitic carbon nitride-perylene polyimide composites. ENERGY & Environ. Mater. doi:10.1002/eem2.12553
Safaei, J., Mohamed, N. A., Mohamad Noh, M. F., Soh, M. F., Ludin, N. A., Ibrahim, M. A., et al. (2018). Graphitic carbon nitride (g-C3N4) electrodes for energy conversion and storage: a review on photoelectrochemical water splitting, solar cells and supercapacitors. J. Mater. Chem. A 6 (45), 22346–22380. doi:10.1039/c8ta08001a
Shamoon, A., Haleem, A., Bahl, S., Javaid, M., and Bala Garg, S. (2022). Role of energy technologies in response to climate change. Mater. Today Proc. 62, 63–69. doi:10.1016/j.matpr.2022.02.025
Sun, S., Wu, Y., Zhu, J., Lu, C., Sun, Y., Wang, Z., et al. (2022). Stabilizing plasma-induced highly nitrogen-deficient g-C3N4 by heteroatom-refilling for excellent lithium-ion battery anodes. Chem. Eng. J. 427, 131032. doi:10.1016/j.cej.2021.131032
Tang, Y., Chen, J., Mao, Z., Roth, C., and Wang, D. (2023). Highly N-doped carbon with low graphitic-N content as anode material for enhanced initial Coulombic efficiency of lithium-ion batteries. Carbon Energy 5 (2). doi:10.1002/cey2.257
Tao, H., Xiong, L., Du, S., Zhang, Y., Yang, X., and Zhang, L. (2017). Interwoven N and P dual-doped hollow carbon fibers/graphitic carbon nitride: an ultrahigh capacity and rate anode for Li and Na ion batteries. Carbon 122, 54–63. doi:10.1016/j.carbon.2017.06.040
Tiwari, R. K., Singh, S. K., Srivastava, N., Mishra, R., Meghnani, D., Patel, A., et al. (2022). Conducting carbon rich graphitic carbon nitride nanosheets with attached nano sulfur copolymer as high capacity cathode for long-lifespan lithium-sulfur battery. Batter. Supercaps 5 (12). doi:10.1002/batt.202200282
Veith, G. M., Baggetto, L., Adamczyk, L. A., Guo, B., Brown, S. S., Sun, X.-G., et al. (2013). Electrochemical and solid-state lithiation of graphitic C 3 N 4. Chem. Mater. 25 (3), 503–508. doi:10.1021/cm303870x
Versaci, D., Amici, J., Francia, C., and Bodoardo, S. (2020). Simple approach using g-C3N4 to enable SnO2 anode high rate performance for Li ion battery. Solid State Ionics 346, 115210. doi:10.1016/j.ssi.2019.115210
Villalobos, L. F., Vahdat, M. T., Dakhchoune, M., Nadizadeh, Z., Mensi, M., Oveisi, E., et al. (2020). Large-scale synthesis of crystalline g-C3N4 nanosheets and high-temperature H2 sieving from assembled films. Sci. Adv. 6 (4), eaay9851. doi:10.1126/sciadv.aay9851
Wang, L., Hou, Y., Xiao, S., Bi, F., Zhao, L., Li, Y., et al. (2019). One-step, high-yield synthesis of g-C 3 N 4 nanosheets for enhanced visible light photocatalytic activity. RSC Adv. 9 (67), 39304–39314. doi:10.1039/C9RA08922E
Wang, S., Liu, X., and Deng, Y. (2023a). Ultrafine Co-species interspersed g-C3N4 nanosheets and graphene as an efficient polysulfide barrier to enable high performance Li-S batteries. Molecules 28 (2), 588. doi:10.3390/molecules28020588
Wang, S., Shi, Y., Fan, C., Liu, J., Li, Y., Wu, X.-L., et al. (2018). Layered g-C 3 N 4 @reduced graphene oxide composites as anodes with improved rate performance for lithium-ion batteries. ACS Appl. Mater. Interfaces 10 (36), 30330–30336. doi:10.1021/acsami.8b09219
Wang, X., Han, D., Ding, Y., Liu, J., Cai, H., Jia, L., et al. (2020). A low-cost and high-yield approach for preparing g-C3N4 with a large specific surface area and enhanced photocatalytic activity by using formaldehyde-treated melamine. J. Alloys Compd. 845, 156293. doi:10.1016/j.jallcom.2020.156293
Wang, Y., Ai, R., Wang, F., Hu, X., Zeng, Y., Hou, J., et al. (2023b). Research progress on multifunctional modified separator for lithium–sulfur batteries. Polymers 15 (4), 993. doi:10.3390/polym15040993
Wang, Y., Liu, L., Ma, T., Zhang, Y., and Huang, H. (2021). 2D graphitic carbon nitride for energy conversion and storage. Adv. Funct. Mater. 31 (Issue 34). doi:10.1002/adfm.202102540
Xia, P., Li, G., Li, X., Yuan, S., Wang, K., Huang, D., et al. (2022). Synthesis of g-C3N4 from various precursors for photocatalytic H2 evolution under the visible light. Crystals 12 (12), 1719. doi:10.3390/cryst12121719
Xu, H., Sun, L., Li, W., Gao, M., Zhou, Q., Li, P., et al. (2022). Facile synthesis of hierarchical g-C3N4@WS2 composite as Lithium-ion battery anode. Chem. Eng. J. 435, 135129. doi:10.1016/j.cej.2022.135129
Yadav, J. K., Rani, B., and Dixit, A. (2023). 2D graphitic carbon nitride as the efficient cathode material for the non-aqueous rechargeable iron-ion battery under an ambient environment. J. Power Sources 567, 232943. doi:10.1016/j.jpowsour.2023.232943
Yao, S., Xue, S., Peng, S., Guo, R., Wu, Z., Shen, X., et al. (2018). Synthesis of graphitic carbon nitride via direct polymerization using different precursors and its application in lithium–sulfur batteries. Appl. Phys. A 124 (11), 758. doi:10.1007/s00339-018-2189-x
Yuan, Z., Hu, Z., Gao, P., Zhang, W., Tang, Y., Li, L., et al. (2022). Graphitic carbon nitride-derived high lithium storage capacity graphite material with regular layer structure and the structural evolution mechanism. Electrochimica Acta 409, 139985. doi:10.1016/j.electacta.2022.139985
Zhang, H., Wu, X., and Li, R. (2020a). Nitrogen doped multi-channel graphite for high rate and high capacity Li ion battery. J. Wuhan Univ. Technology-Mater. Sci. Ed. 35 (1), 65–70. doi:10.1007/s11595-020-2228-2
Zhang, J., Chen, Y., and Wang, X. (2015). Two-dimensional covalent carbon nitride nanosheets: synthesis, functionalization, and applications. Energy Environ. Sci. 8 (11), 3092–3108. doi:10.1039/c5ee01895a
Zhang, J., Tian, Y.-F., Xin, S., and Guo, Y.-G. (2023a). Space-confined electrochemical reactions and materials for high-energy-density batteries. Accounts Mater. Res. 4 (7), 580–590. doi:10.1021/accountsmr.3c00017
Zhang, W., Fu, Y., Liu, W., Lim, L., Wang, X., and Yu, A. (2019). A general approach for fabricating 3D MFe2O4 (M=Mn, Ni, Cu, Co)/graphitic carbon nitride covalently functionalized nitrogen-doped graphene nanocomposites as advanced anodes for lithium-ion batteries. Nano Energy 57, 48–56. doi:10.1016/j.nanoen.2018.12.005
Zhang, X., Yang, S., Chen, Y., Li, S., Tang, S., Shen, D., et al. (2020b). Effect of phosphorous-doped graphitic carbon nitride on electrochemical properties of lithium-sulfur battery. Ionics 26 (11), 5491–5501. doi:10.1007/s11581-020-03728-w
Zhang, Y., Lu, S., Wang, Z., Volkov, V., Lou, F., and Yu, Z. (2023b). Recent technology development in solvent-free electrode fabrication for lithium-ion batteries. Renew. Sustain. Energy Rev. 183, 113515. doi:10.1016/j.rser.2023.113515
Zhao, Y., Zhang, J., and Qu, L. (2015). Graphitic carbon nitride/graphene hybrids as new active materials for energy conversion and storage. ChemNanoMat 1 (Issue 5), 298–318. doi:10.1002/cnma.201500060
Zhou, Y., Tian, R., Duan, H., Wang, K., Guo, Y., Li, H., et al. (2018). CoSe/Co nanoparticles wrapped by in situ grown N-doped graphitic carbon nanosheets as anode material for advanced lithium ion batteries. J. Power Sources 399, 223–230. doi:10.1016/j.jpowsour.2018.07.110
Zhu, Y.-P., Lei, Y., Ming, F., Abou-Hamad, E., Emwas, A.-H., Hedhili, M. N., et al. (2019). Heterostructured MXene and g-C3N4 for high-rate lithium intercalation. Nano Energy 65, 104030. doi:10.1016/j.nanoen.2019.104030
Keywords: graphitic, carbon nitride, lithium, rechargeable, electrode
Citation: Sajid M, Chandio ZA, Hwang B, Yun TG and Cheong JY (2023) Graphitic carbon nitrides as electrode supporting materials for lithium-ion batteries: what lies ahead in view of the current challenges?. Front. Energy Res. 11:1285044. doi: 10.3389/fenrg.2023.1285044
Received: 29 August 2023; Accepted: 17 November 2023;
Published: 22 December 2023.
Edited by:
Nithyadharseni Palaniyandy, University of South Africa, South AfricaReviewed by:
Yang Chen, Shanghai Normal University, ChinaCopyright © 2023 Sajid, Chandio, Hwang, Yun and Cheong. This is an open-access article distributed under the terms of the Creative Commons Attribution License (CC BY). The use, distribution or reproduction in other forums is permitted, provided the original author(s) and the copyright owner(s) are credited and that the original publication in this journal is cited, in accordance with accepted academic practice. No use, distribution or reproduction is permitted which does not comply with these terms.
*Correspondence: Jun Young Cheong, anVuLmNoZW9uZ0B1bmktYmF5cmV1dGguZGU=; Tae Gwang Yun, eXRrMDQwMkBham91LmFjLmty; Byungil Hwang, Ymlod2FuZ0BjYXUuYWMua3I=