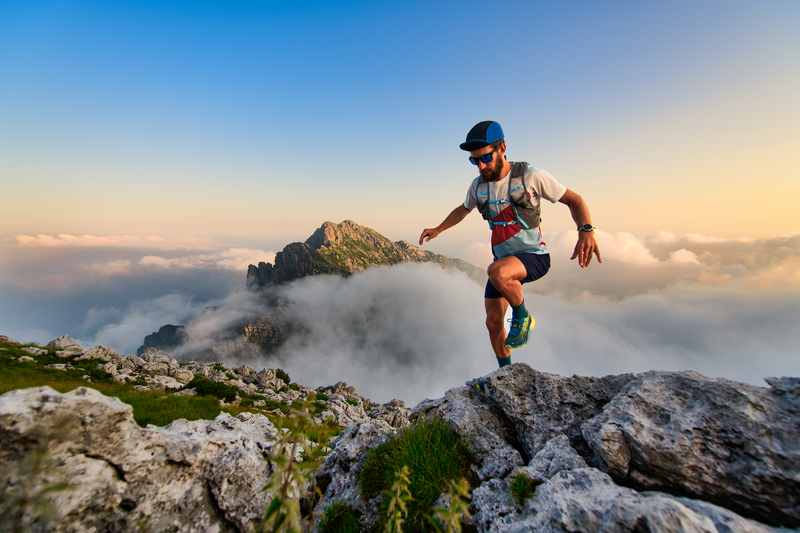
95% of researchers rate our articles as excellent or good
Learn more about the work of our research integrity team to safeguard the quality of each article we publish.
Find out more
MINI REVIEW article
Front. Energy Res. , 07 September 2023
Sec. Energy Storage
Volume 11 - 2023 | https://doi.org/10.3389/fenrg.2023.1246327
This article is part of the Research Topic Advances in Thermal and Electrochemical Energy Storage View all 5 articles
The energy storage mechanism and manufacturing equipment of sodium-ion batteries (SIBs) and lithium-ion batteries (LIBs) are similar. However, SIBs offer several advantages, such as low cost, abundant resources, environmental friendliness, and high safety. Consequently, they have garnered significant attention. SIBs are poised to be potential replacements for LIBs and represent ideal candidates in the field of large-scale energy storage. Layered transition-metal oxides (TMOs) are considered highly promising cathode materials due to their high average voltage, high specific capacity, and ease of synthesis. This paper provides a review of recent advances in layered TMOs for SIBs, including NaxCoO2, NaxMnO2, NaxFeO2, and their derivatives. Furthermore, the challenges and prospects in the development of layered TMOs are also discussed. It is hoped that this review will assist in the design and preparation of SIBs with superior electrochemical performance and further facilitate their practical application.
Energy is the most pressing issue of the 21st century. The unregulated extraction and widespread utilization of traditional fossil fuels, such as coal, petroleum, and natural gas, have resulted in severe environmental pollution. With the increasing demand for electricity and energy, it is imperative to explore new and environmentally-friendly energy storage systems (Wang T. et al., 2018; Niu et al., 2023).
Currently, the most efficient electric energy storage devices, namely, secondary batteries, have gained widespread utilization (González et al., 2016). These batteries are not only employed in mobile devices but also in high-capacity batteries for automobiles and wind power generation equipment. They significantly contribute to enhancing societal energy efficiency. Therefore, there is a pressing need to discover low-cost energy storage devices that can utilize abundant natural resources. Rechargeable batteries offer a viable solution for large-scale energy storage (Gao et al., 2020; Guo et al., 2021; Wei et al., 2021).
Since their commercialization in 1991, rechargeable lithium-ion batteries (LIBs) have earned recognition as the most successful and advanced energy storage devices due to their remarkable energy density and long lifespan. Currently, LIBs hold a prominent position in the field of energy storage (Liu et al., 2020). Indeed, the increasing demand for LIBs will inevitably result in a scarcity of lithium resources and a rise in lithium prices. Calculations have indicated that the limited availability of lithium resources poses a challenge for LIBs to support the growth of electric vehicles and large-scale energy storage industries. Additionally, the recovery and utilization rates of lithium are currently suboptimal, leading to the gradual depletion of lithium resources for battery production. The high cost and scarcity of lithium ore (0.006 wt%) further restrict their widespread application. Consequently, it becomes crucial to explore alternative energy storage systems that offer abundant resources and low costs (Wang Q. et al., 2020).
Recently, SIBs have garnered significant attention as a viable alternative to LIBs due to their similar electrochemical mechanism and cost-effectiveness (Armand, 1980; Ding J. et al., 2013; Xiang et al., 2015; Fang et al., 2017). Moreover, SIBs demonstrate higher stability and safety during the cyclic process due to the higher standard electrode potential of sodium (−2.71 V) compared to lithium (−3.04 V) (Ellis et al., 2012; Slater et al., 2013; Kundu et al., 2015; Zhu et al., 2015; Wang et al., 2016). The structure and principles of SIBs closely resemble those of LIBs. They consist of a positive electrode, negative electrode, and electrolyte, allowing for the storage and release of energy through the continuous insertion and extraction of sodium ions in the electrode materials. The concept of SIBs, often referred to as the “rocking chair battery,” was initially proposed by the Armand team in 1980 (Armand, 1980). During the charging process, sodium ions are dissociated from the cathode and intercalated into the anode through the electrolyte. Simultaneously, electrons flow through an external circuit to the anode, maintaining charge balance. The discharge process follows the reverse mechanism (Qian et al., 2013; Wen et al., 2014).
Cathode material of SIBs plays a key role in demonstrating the electrochemical performance of SIBs. At present, the mainstream cathode materials mainly include layered transition-metal oxides (TMOs) (Jiang et al., 2014; Yang and Wei, 2020; Zhao X.-D. et al., 2021), polyanionic compounds (Lv et al., 2020; Essehli et al., 2021), organic cathode materials (Wang et al., 2014; Huang et al., 2017) and prussian blue analogues (Zhu et al., 2019; Du and Pang, 2021). All of them have appropriate spaces and channels for Na+ to transfer and store, the corresponding crystal structure diagrams are exhibited in Figures 1A–E.
FIGURE 1. Crystal structure diagrams of (A) layered NaMO2, (B) olivine NaMPO4, (C) NASICON-type (D) fluorophosphates, and (E) Prussian blue analogues (Wang T. et al., 2018). (F) The classification of layered structure with the sheets of edge-sharing MeO6 octahedra and phase transition processes caused by Na+ extraction (Su et al., 2013).
In the battery system, the electrode material plays a critical role in the performance of battery systems. With the growing demand for new energy storage systems and the expanding market for SIBs, the research on sodium-ion electrode materials with excellent electrochemical performance has become increasingly significant. The development of such materials is crucial for determining and advancing the industrial application of SIBs. Layered TMOs have attracted considerable attention in this regard due to their high average voltage and ease of synthesis. Various conventional methods for positive electrode material modification, such as doping, surface coating, and preparation of materials with different phase structures, have been explored (Jamil et al., 2023).
This paper aims to summarize and analyze the current research status of layered TMOs, potentially offering insights for selecting practical layered cathode materials for the industrial application of SIBs.
Historically, the development of cathode materials for SIBs could actually be traced back to the 1970s, almost parallel to the research of lithium storage materials, due to their similar atomic structure and electrochemical characteristics. The early research on cathode materials mainly focused on TMOs. In 1970 and 1972, Fouassier (Delmas et al., 1981) published papers on crystal phase diagrams of NaxMnO2 and NaxCoO2 materials. Subsequently, Delams also began to study NaxMO2 layered oxides in 1978, describing the internal structure and mechanism. Since then, the theory of layered oxide phase structure has been completely proposed (Jean et al., 1971; Mendiboure et al., 1985; Schulze et al., 2008). Layered positive NaxMO2 had been studied by many researchers and the crystal structure was classified into tunnel type and layered structure according to sodium content. The layered structure consists of a shared MO6 and sodium layer. According to Demals et al., layered cathode oxides could be mainly classified into P2 type, O3 type and P3 type. O or P represent different meanings of coordination environments, n refers to the number of transition metal layers.
The sodium content directly determines the structural phases. The x value of O3 phase is in the range of 0.83–1.0, and the P2 phase is between 0.67 and 0.80 (Xu et al., 2014; Li et al., 2016). The above kinds of crystal structure are shown in Figure 1F (Su et al., 2013). Table 1 summarizes electrochemical properties of layered transition metal cathodes in SIBs which was reported recently.
As early as 1981, Delmas et al. (1981) pioneered NaxCoO2 system by solid state reaction between 500°C and 800°C under oxygen atmosphere, four different phases were obtained, including P′3 phase (0.55 ≤ x ≤ 0.6), P2 phase (0.64 ≤ x ≤ 0.74), O′3 phase (x = 0.77) and O3 phase (x = 1) and they were all indexed into layered structure. Unlike P3 phase and O3 phase, P2 phase not only has lamellar displacement, but also involves rotation of (CoO6) octahedron and Co-O bond rupture when the phase transfers to P3 or O3. Thus, the P2 structure remains unchanged throughout the electrochemical process. However, for O3 type NaCoO2, a reversible phase transition O3−P3−P′3 in the electrochemical process can be observed in Figure 2B. Jean et al. (1971) respectively synthesized Na0.7CoO2 intercalation compound by using different preparation techniques such as solid-stated reaction, sol-gel and hydrothermal method. The results showed that the cathode material with uniform ball-shaped morphology synthesized by sol-gel method demonstrated the best performance. The contribution of Na+ ions to the conductivity was also confirmed, but the corresponding electrochemical performance was not mentioned. The electrochemical properties of Na0.71CoO2 were systematically investigated. In the relatively narrow voltage range of 2–3.5 V, it delivered 70.4 mAh g−1 reversible capacity at 0.08 C in the first week with an initial coulomb efficiency of 90% (Figure 2C). At the magnification of 0.08 C, the cyclic life was long and stable, almost reaching 100% (Figure 2D). In-situ XRD (Figure 2E) results showed that the diffraction peaks of (002), (004) and (100) shifted with the insertion/extraction of Na+, indicating the composition range of sodium has changed slightly. Carlier et al. (2011) successfully prepared P2-type Na2/3Co2/3Mn1/3O2, the discharge profile shown in Figure 2F presents nine different platforms in the x range 0.5–0.9. When x = 1/2 and 2/3, a large voltage step could be observed, indicating that Na+/vacancy was arranged in the structure. When Mn4+ partly replaced Co4+, Na1/2Co2/3Mn1/3O2 with well-ordered structure was formed. Moreover, P2-phase Na0.74CoO2 prepared by Ding J.-J. et al. (2013) exhibited 107 mAh g−1 initial discharge capacity at 0.1 C with 94% reversible capacity remained after 40 weeks under 2–3.8 V, showing good cycling performance.
FIGURE 2. Electrochemical performance of Na0.71CoO2 (A) Charge/discharge profiles. (B) Cyclic performance at 0.08 C. (C) In-situ XRD results of the cathode in the initial cycle. (D) Discharge profiles of Na0.71CoO2 in the first two cycles (E) Galvanostatic charge/discharge profiles of Na//Na2/3Co1/2Ti1/2O2.
In recent years, Na0.7CoO2 microspheres using CoO3 microspheres as precursor self-template has been investigated (Zhu et al., 2005). Due to its unique structure, the material exhibited high reversible specific capacity (125 mAh g−1), excellent rate property (64 mAh g−1 reversible specific capacity at 16 C rate). Sabi et al. (2017) prepared P2-Na2/3Co1/2Ti1/2O2 in air, and compared the electrochemical behavior of the obtained material with NaxCoO2. The constant current charge-discharge cycle showed that the nine potential steps of NaxCoO2 were effectively reduced by the substitution of Co by Ti (Figure 2E). In addition, the substitution of Co by Ti has promoted the improvement of electrochemical performance. The P2-Na2/3Co1/2Ti1/2O2 delivered 100 mAh g−1 initial specific discharge capacity in Na half cell between 2.0 and 4.2 V with 98% capacity retention after 50 rounds. Moreover, Huang et al. (2021) proposed a Mg/Zn co-doping strategy to increase the interfacial spacing thus providing a broad ion diffusion channel for rapid Na + insertion/extraction. The modified material possessed a 67.2 mAh g−1 at 10 C. Zhu et al. (2016) synthesized P2-Na0.67Co0.5Mn0.5O2 cathode, which was easy to quickly diffuse Na+ and establish stable structure.
Recently, in order to further optimize the Na + storage in P2 type Na-Mn-Co-O cathode, cationic doping/substitution has been studied deeply to regulate the layer spacing, suppressing active Mn3+ ions and stabilizing the lattice structure. Wang X. et al. (2021) synthesized Na0.67Co0.20Mn0.79Ce0.01O2 using solid-state reaction, where Ce occupied the Mn-site. The capacity retention was 92.3% after 100 cycles at 0.1 C and 91.7% after 400 rounds at 1 C. The cathode demonstrated excellent cyclic performances. The work proved that small amount of Ce could effectively enhance the stability, which alleviated the Jahn-teller effect and guarantee the phase transition to be highly reversible. Chen Y. et al. (2020) synthesized micron sized Na0.65[Ni0.17Co0.11Mn0.72]O2 by coprecipitation, benefitting from the spherical morphology, the material provided sufficient interface between electrode and electrolyte solution, so the transmission path of sodium ions and electrons was shortened, the as-prepared cathode delivered 187 mAh g-1 initial capacity at 12 mA g-1. After 500 cycles, its abnormal capacity retention rate reached 74.7% at a current density as high as 600 mA g−1.
Chen T. et al. (2020) designed and synthesized multilayer directional lamination Na0.67Mn0.6Ni0.2Co0.1Cu0.1O2 with P2 structure by a simple sol-gel method, a large three-dimensional framework was gained, which enabled sodium ions to diffuse. A reversible capacity of 131.3 mAh g-1 was acquired at 0.1 C, the outstanding retention rate of 80.0% at 1 A g−1 after 500 cycles was obtained. Such remarkable electrochemical performance was attributed to high Na + mobility and low Na + diffusion resistance. Pang et al. (2019) prepared P2 type layered oxide Na2/3Mn1/2Co1/3Cu1/6O2. Due to the collaborative improvement of polymetal ions, the electrode showed good cyclic stability and excellent sodium ion transport performance. And the capacity retention was still achieved at 83.5% after 100 cycles at 100 mAh g−1.
Besides the above efforts, some other transition metal elements have been employed to modify the layered structures. Wang et al. (2019) prepared Na0.67Co0.25Mn0.75-xNbxO2 with different x value ranging from 0 to 0.045 through simple solid-stated method. Because of Nb5+, the structural stability was greatly enhanced and the charge transfer resistance was reduced. The corresponding Nb-doped cathode exhibited 126.7 mAh g−1 initial discharge capacity and the cycling stability was obviously developed than that of Na0.67Co0.25Mn0.75O2. Furthermore, Wang Y. et al. (2018) prepared P2-type Na0.70Mn0.80Co0.15Zr0.05O2 and suggested that Zr could significantly increase the rate and cycling properties of P2 type material, after 50 cycles, the capacity remained 88% of the initial cycle.
The layered NaxMnO2 cathodes were firstly explored by Parant et al. (1971) and Mendiboure et al. (1985) reproduced the crystal chemistry of the NaxMnO2 system in 1985. In the x range of 0–0.44, the structure was found to be three-dimensional, however, when x > 0.5 the structure turned to be two-dimensional. Due to different synthesis conditions and stoichiometric ratios, the layered oxides of Mn group are mainly divided into P2-Na0.7MnO2+y, monocline O′3 type α-NaMnO2 and orthogonal P2 type β-NaMnO2 (Figure 3A). The sodium storage properties of these three compounds were systematically studied in this paper. Shibata et al. (2014) studied the diffusion of lamellar NaxMnO2 at (0.49 < x < 0.75) and compared it with NaxCoO2. In 1994, Doeff et al. (2002) prepared NaxMnO2. When x is less than 0.45, the cathode material had a tunnel structure, such as Na0.44MnO2. When x was greater than 0.45, the crystal structure of the cathode material was generally layered. β-NaMnO2 has a layered structure which was different from the traditional NaMO2 structure. The crystal shape of the traditional layered structure (O3, P2, P3, etc.) depends on the stacking order of the planar layers of the MnO6 octahedron. β-NaMnO2 was consist of a serrated layered edge shared MnO6 octahedron, in which sodium ions was located at the octahedral position as shown in Figure 3B. Figure 3C shows the symbiotic illustration between α and β-NaMnO2 (Billaud et al., 2015). In Figure 3D, when the 2θ value between 12o and 15o, the intensity of (001) peak diminished, while the new peak at lower angles increased. However, from the in-situ XRD data, it could be concluded that the long-range structure collapsed significantly and many peaks disappeared at low sodium levels. In most cases, the remaining peaks showed significant broadening as many peaks disappeared. β-NaMnO2 showed high capacity (about 190 mAh g−1 at 0.05 C), good rate capacity (142 mA h g-1 at 2 C) and excellent cycling stability (100 mA h g-1 at 2 C rate after 100 cycles) in Figures 3E,F (Huang et al., 2021). Jo et al. (2014) synthesized pure α-NaMnO2 using solid state reaction of Mn2O3 and Na2CO3. The material displayed 146 mAh g−1 initial discharge capacity and remained 136 mAh g−1 after 20 cycles.
FIGURE 3. (A) Na0.7MnO2 (P2) and α-NaMnO2 (O′3) structures β-NaMnO2. (B) Schematic representation of β-NaMnO2 in the Pmnm space group. (C) Intergrowth model of α and β-NaMnO2. (D) In-situ XRD patterns of β-NaMnO2 in the initial cycle. (E) Rate and (F) Cycling performance of Na/α-NaMnO2 at different current densities. (G) In-situ XRD results of Na0.58Ni1/3Mn2/3O1.95 between different voltage windows and the corresponding charge/discharge profiles.
Na0.7MnO2 with the P2 structure displayed a high capacity of 200 mAh g−1. However, due to structural and chemical problems, Na0.7MnO2 suffers from severe capacity decay during repeated charging and discharging. Phase transitions (P2 to O2 or OP4) in high voltage range (low Na+ content) and the transitions between different Na+/vacancy ordered modes at specific Na+ stoichiometry were the main causes of capacity loss (Baster et al., 2016).
Le et al. (2021) evaluated the effect of different 3D metals (Fe, Co and Ni) replacing Mn on the electrochemical performance of P2-NaxMe1/3Mn2/3O2 cathodes. The results showed that both NaxCo1/3Mn2/3O2 and NaxFe1/3Mn2/3O2 had high specific capacities of larger than 140 mAh g−1, while NaxCo1/3Mn2/3O2 showed better cyclic performance and had a capacity retention rate of 83% after 50 rounds. In contrast, NaxNi1/3Mn2/3O2 exhibited an excellent cyclic stability, but the specific capacity was relatively low, which was only 110 mAh g−1 in the initial cycle. A kind of cathode material Ni-Mn-based P2-type Na0.58Ni1/3Mn2/3O1.95 was synthesized by a co-precipitation reaction, which displayed a high capacity of 153 mAh g-1 in the initial cycle. After optimizing the voltage window to 2.6–3.8 V, the capacity retention increased from 14% to 94.5% after 500 cycles. According to in-situ XRD, the existence of oxygen anion group [O2]x− (0 < x < 4) under high pressure was the main cause of battery capacity attenuation in Figure 3G (Cai et al., 2020). Arjunan et al. (2020) successfully prepared Na0.67Ni0.23Zn0.1Mn0.67O2 electrode material by a conventional solid-state method. When the cut-off voltage was exceeded (≥4.2 V), the capacity and cycle performance were attenuated due to stacking fault, and the substitution of Zn2+ ions acted as a stabilizer in the P2-layer structure of Na-Ni-Mn-O system.
In addition, Zhang et al. (2020) prepared Na0.91MnO2 as the precursor material, and prepared Na0.67Mn0.67Ni0.33O2 nanoparticles by Ni doping under the same conditions, which effectively improved the specific capacity. Furthermore, Na0.67Mn0.67Ni0.33-xCoxO2 was prepared by Co doping. It was found that the electrode material had more stable cyclic performance. The specific discharge capacity in the initial week delivered above 160 mAh g−1 at 0.1 C, and the specific discharge capacity remained 120 mAh g−1 after cycling for 200 rounds. As the current density increased to 1 C, the reversible capacity reached 90 mAh g−1. The results showed that Ni and Co co-doping could effectively improve the specific capacity, rate and cycling stability of electrode materials. Wang H. et al. (2020) systematically prepared and studied the structure and electrochemical performance of P2-type Na0.67Mn0.6Ni0.2Co0.2O2. The addition of La reduced Mn3+ the amount of manganese trivalent ions in the lattice, alleviated Jahn-Teller distortion, and enhanced Na+ diffusion kinetics. Even at 8 C, it could deliver 86 mAh g-1 reversible discharge capacity. Xiao et al. (2019) proposed Na2/3Ni1/6Mn2/3Cu1/9Mg1/18O2 layered oxide after the morphology and structure optimization, it showed good electrochemical performance under the cut-off voltage of 4.2 V. Kouthaman et al. (2020) prepared Na0.9Mn0.55Ni0.35Cu0.1O2 oxide with O3 structure, benefiting from the small amount of copper which effectively improve the O3 structure, the electrochemical performance was greatly enhanced.
Jiang et al. (2022) prepared P2-Na0.6Ni0.3–xMn0.7CuxO2 with different copper content of 0–0.2 and the mechanism of improving electrochemical properties was studied comprehensively. It has been discovered that a small amount of copper can inhibit the P2-O2 phase transition, consequently stabilizing the crystal structure and enhancing the cycling stability. The augmentation of the layer spacing, resulting from copper doping, contributes to the dynamics of charge transfer, thereby ensuring excellent rate performance. Furthermore, copper substitution enhances the moisture stability of the electrode due to the increased initial potential in the charging process from the Cu2+/Cu3+ redox couple.
To mitigate the Jahn-Teller effect, interfacial instability, and phase transition in Mn-Ni based materials with a P2 structure under high pressure, research has been conducted on cationic doping/substitution as a means to stabilize the lattice structure and enhance the electrochemical performance. For example, Cu-Mg co-doping (Chen T. et al., 2020), Li-doping (Chen et al., 2021) and Mg-doping (Yoda et al., 2020) were proved to be effective to promote the cycling stability of P2-type cathode material. In a recent study, the substitution of copper and cobalt formed stable copper oxide and cobalt oxide octahedrons, and the lattice structure was adjusted to enhance the diffusion kinetics of Na+ in layered manganese-based oxide Na0.67Mn0.6Ni0.1Cu0.20Co0.1O2 prepared by Zhao Q. Q. et al. (2021).
NaFeO2 is a traditional layered oxide which consists of a closed oxygen array as a possible candidate material for rechargeable SIBs, α-NaFeO2 was also regarded as “O3″ structure by Delmas. Qin et al. (2021) synthesized Na2/3Fe1/2Mn1/2O2 through sol-gel method. The optimum calcination temperature was 900°C. The material displayed hexagonal plate shape with an average diameter of 2–4 μm, and showed 243 mAh g−1 initial discharge capacity at 26 mA g-1 with excellent cycling performance.
Sui et al. (2019) prepared Na2/3Fe1/2Mn1/2O2 by adopting simple spray drying method at low temperature. The as-obtained Na2/3Fe1/2Mn1/2O2 displayed good rate and cycling performances. The initial discharge capacities was 217.9, 171.3 and 117.4 mAh g−1 at 0.1 C, 0.5 C and 2 C, respectively. This oxide was expected to be used as the suitable cathode for SIBs. Ding et al. (2017) studied the electrochemical properties of Na2/3Fe1/3Mn2/3O2 oxide in ionic liquid electrolyte which was heated to 90°C for the first time. At 20 mA g-1, the electrode demonstrated a 227 mAh g−1 initial discharge capacity. Furthermore, Kumar et al. (2021) found that the electrochemical performance of P2-Na0.67Mn0.5Fe0.5O2 decreased slowly due to surface reconstruction, ingrain cracks, reduction and dissolution of transition metal and decomposition of electrolyte, and suggested that element doping is an effective way to solve the above defects.
Recently, Darbar et al. (2021) reported a titanium doping strategy based on a wet chemical method to improve electrochemical properties of Na0.67Mn0.5Fe0.5O2. Ti4+ replaces Mn and Fe atoms in the material and effectively reduced the Jahn-teller distortion. It was found that Ti4+ could increase the thickness of sodium layer and minimize the volume strain in the layered structure, showing enhanced structural stability. Yoda et al. (2020) synthesized O3-Na5/6Fe1/2Mn1/2O2 and Na5/6Fe1/3Mn1/2Me1/6O2 (Me = Mg or Cu). Although the undoped sample provided more than 150 mAh g−1 discharge capacity, its exhibited poor wet-air stability and cycling stability in aprotic sodium batteries, but the addition of copper and magnesium can improve the wet air stability. The following effort results will provide ideas to improve the electrochemical performance of SIBs.
Wang S. et al. (2021)) designed a submicron O3-NaCrO2 microsphere (s-NaCrO2), which had a higher apparent Na+ diffusion coefficient. The as-prepared s-NaCrO2 showed impressive electrochemical performances, which had a very high reversible capacity of 90 mAh g−1 at 50 C. Moreover, when cycled at a quite high rate of 20 C, it remained 87% of its initial discharge capacity after 1,500 cycles. In addition, s-NaCrO2 had advantages at high temperature (50°C) and low temperature (−10°C). Hamani et al. (2011) observed that P2-Na0.7VO2 generated a very reversible charge-discharge behavior of ∼105 mAh g−1 within the voltage range of 1.2–2.6 V Na2RuO3 reached 140 mAh g−1 with average potential of 2.8 V/Na+. In the 20 cycles, the capacitance retention and coulomb efficiency were close to 100%, indicating that the electrochemical reaction has high reversibility. Siriwardena et al. (2019) synthesized iron substituted Na1.33Ru0.67O2 (NRFOx) phase by conventional solid-state method, NRFO5 showed the highest specific discharge capacity of 110 mAh g−1 and 61 mAh g−1 at 0.2 C and 2 C rates in the voltage range of 2.0–3.7 V. The results showed that the level of iron substitution significantly affected the structural stability and discharge capacity.
The exploration of layered Na2MO3 is of great necessity to prepare new TMOs, although the structural evolution and electrochemical performance optimization during Na+ insertion and extraction still need to be further studied.
In this paper, we provide a comprehensive summary of various layered transition metal oxides (TMOs) used as cathode materials for sodium-ion batteries (SIBs), such as cobalt-based, manganese-based, iron-based, and other related materials. While significant research has been conducted on layered TMOs, there is still a need for continuous improvement and further exploration of their electrochemical properties and Na+ de/intercalation mechanisms. For future exploration of cathode materials, we suggest focusing on the following aspects.
(1) It is important to further explore the synthesis process and conditions, establish mathematical model and theoretical simulation to predict the performance of cathode materials, and then continuously optimize the cathode materials that are suitable for energy storage system.
(2) Advanced testing technologies such as in-situ XRD and synchrotron radiation are needed to further study the charge discharge process and Na+ de/intercalation mechanism of cathode materials, and build the structure-activity relationship between phase transformation process and electrochemical properties.
(3) It is important to develop new kinds of electrolytes to alleviate side reactions, thus preventing the dissolution of active substances in electrolytes. This is an urgent problem to be solved before the industrialization of SIBs.
(4) The electrochemical properties can be significantly improved by doping metal ions, surface coating or structural design, and further investigation of the relationship between structure/composition and properties is still needed.
Finally, it is hoped that the summary of this paper can provide some references for the design of new materials. With the in-depth study of SIBs, it is believed that low-cost SIBs will be widely employed in the field of large-scale energy storage.
JL: Investigation, Writing—original draft. JZ: Review and Editing. YH: Review and Editing. YZ: Review and Editing. YY: Review and Editing. SB: Supervision, Review and Editing.
This work was supported by the National Natural Science Foundation of China (52004121, 52274296, 52001081, 52071091).
The authors declare that the research was conducted in the absence of any commercial or financial relationships that could be construed as a potential conflict of interest.
All claims expressed in this article are solely those of the authors and do not necessarily represent those of their affiliated organizations, or those of the publisher, the editors and the reviewers. Any product that may be evaluated in this article, or claim that may be made by its manufacturer, is not guaranteed or endorsed by the publisher.
Arjunan, P., Kouthaman, M., Kannan, K., Diwakar, K., Sivakumar, M., Raghu, S., et al. (2020). Stable prismatic layer structured cathode material via cation mixing for sodium ion battery. Ionics 26 (10), 4543–4551. doi:10.1007/s11581-020-03592-8
Armand, M.-B. (1980). Intercalation electrodes. Mater. Adv. Batter. 2, 145–161. doi:10.1007/978-1-4684-3851-2_7
Baster, D., Zajaz, W., Kondracki, L., Hartman, F., and Molenda, J. (2016). Improvement of electrochemical performance of Na0.7Co1−Mn O2—Cathode material for rechargeable sodium-ion batteries. Solid State Ionics 288, 213–218. doi:10.1016/j.ssi.2016.01.003
Bhide, A., and Hariharan, K. (2011). Physicochemical properties of NaxCoO2 as a cathode for solid state sodium battery. Solid State Ionics 192 (1), 360–363. doi:10.1016/j.ssi.2010.04.022
Billaud, J., Clément, R. J., Armstrong, R., Jesús, C. -V., Patrick, R., Grey, C.-P., et al. (2015). ChemInform abstract: β-NaMnO2: A high-performance cathode for sodium-ion batteries. J. Am. Chem. Soc. 46 (49), 17243–17248. doi:10.1002/chin.201514014
Bucher, N., Hartung, S., Nagasubramanian, A., Yan, L. -C., and Madhavi, S. (2014). Layered Na<i>x</i>MnO2+<i>z</i> in sodium ion batteries–influence of morphology on cycle performance. ACS. Appl. Mat. Interf. 6 (11), 8059–8065. doi:10.1021/am406009t
Caballero, A., Hernan, L., Morales, J., Sanchez, L., Santos Pena, J., and Aranda, M. A. G. (2002). Synthesis and characterization of high-temperature hexagonal P2-Na0.6MnO2 and its electrochemical behaviour as cathode in sodium cells. J. Mat. Chem. 12 (4), 1142–1147. doi:10.1039/B108830K
Cai, X.-Y., Xu, Y. -N., Meng, L., Wei, X. -J., Xiong, F. -Y., Xiong, T. -F., et al. (2020). Insight into the capacity decay of layered sodium nickel manganese oxide cathodes in sodium ion batteries. J. Alloy. Comp. 820, 153093. doi:10.1016/j.jallcom.2019.153093
Carlier, D., Cheng, J.-H., Berthelot, M., Guignard, M., Yoncheva, R., Stoyanova, B., et al. (2011). The P2-Na2/3Co2/3Mn1/3O2 phase: structure, physical properties and electrochemical behavior as positive electrode in sodium battery. Dalton. T 40 (36), 9306–9312. doi:10.1039/C1DT10798D
Chen, T., Liu, W. F., Zhuo, Y., Hu, H., Zhu, M. L., Cai, R. Z., et al. (2020a). Single-phase P2-type layered oxide with Cu-substitution for sodium ion batteries. J. Energy. Chem. 43, 148–154. doi:10.1016/j.jechem.2019.08.016
Chen, X., Zhao, Z., Huang, K., and Tang, H. (2021). Al-substituted stable-layered P2-Na0.6Li0.15Al0.15Mn0.7O2 cathode for sodium ion batteries. Int. J. Energy Res. 45 (7), 11338–11345. doi:10.1002/er.6606
Chen, Y., Su, G., Cheng, X., Du, T., Han, Y., Qiang, W., et al. (2020b). Electrochemical performances of P2-Na2/3Ni1/3Mn2/3O2 doped with Li and Mg for high cycle stability. J. Alloy. Comp. 858, 157717. doi:10.1016/j.jallcom.2020.157717
Darbar, D., Muralidharan, N., Hermann, R. P., Nanda, J., and Bhattacharya, I. (2021). Evaluation of electrochemical performance and redox activity of Fe in Ti doped layered P2-Na0.67Mn0.5Fe0.5O2 cathode for sodium ion batteries. Electrochimica Acta 380, 138156. doi:10.1016/j.electacta.2021.138156
Delmas, C., Braconnier, J. J., Fouassier, C., and Hagenmuller, P. (1981). Electrochemical intercalation of sodium in NaxCoO2 bronzes. Solid State Ionics 3, 165–169. doi:10.1016/0167-2738(81)90076-X
Ding, C.-S., Nohira, T., and Hagiwara, R. (2017). Charge-discharge performance of Na 2/3 Fe 1/3 Mn 2/3 O 2 positive electrode in an ionic liquid electrolyte at 90 °C for sodium secondary batteries. Electrochim. Acta 271, 412–416. doi:10.1016/j.electacta.2017.02.069
Ding, J.-J., Zhou, Y.-N., Sun, Q., Yu, X.-Q., Yang, X.-Q., and Fu, Z.-W. (2013b). Electrochemical properties of P2-phase Na0.74CoO2 compounds as cathode material for rechargeable sodium-ion batteries. Electrochim. Acta 87, 388–393. doi:10.1016/j.electacta.2012.09.058
Ding, J., Wang, H.-L., Li, Z., Kohandehghan, A., Cui, K., Xu, Z.-W., et al. (2013a). Carbon nanosheet frameworks derived from peat moss as high performance sodium ion battery anodes. ACS Nano 7, 11004–11015. doi:10.1021/nn404640c
Doeff, M. -M., Richardson, T. -J., Hollingsworth, J., Yuan, C. -W., and Gonzals, M. (2002). Synthesis and characterization of a copper-substituted manganese oxide with the Na0.44MnO2 structure. J. Adhes. 112 (1), 294–297. doi:10.1016/S0378-7753(02)00449-4
Du, G., and Pang, H. (2021). Recent advancements in prussian blue analogues: preparation and application in batteries. Energy Storage Mater 36, 387–408. doi:10.1016/j.ensm.2021.01.006
Ellis, L. D., Hatchard, T. D., and Obrovac, M. N. (2012). Reversible insertion of sodium in tin. J. Electrochem. Soc. 159, A1801–A1805. doi:10.1149/2.037211jes
Essehli, R., Alkhateeb, A., Mahmoud, A., Boschini, F., Ben, Y. H., Amin, R., et al. (2021). Optimization of the compositions of polyanionic sodium-ion battery cathode NaFe2xVx(PO4)(SO4)2. J. Power Sources 469, 228417. doi:10.1016/j.jpowsour.2020.228417
Fang, Y., Chen, Z. X. A., Yang, H.-X., and Cao, Y.-L. (2017). Recent developments in cathode materials for Na ion batteries. Acta Phys. Chim. Sin. 33 (1), 211–241. doi:10.3866/PKU.WHXB201610111
Gao, R.-M., Zheng, Z.-J., Wang, P.-F., Hu, Y., and Cao, F.-F. (2020). Recent advances and prospects of layered transition metal oxide cathodes for sodium-ion batteries. Energy Storage Mater. 30, 9–26. doi:10.1016/j.ensm.2020.04.040
González, A., Goikolea, E., Barrena, J., and Mysyk, R. (2016). Review on supercapacitors: technologies and materials. Renew. Sust. Energy Rev. 58, 1189–1206. doi:10.1016/j.rser.2015.12.249
Guo, Y., Wang, P., Niu, Y., Zhang, X., Li, Q., Yu, X., et al. (2021). Boron-doped sodium layered oxide for reversible oxygen redox reaction in Na-ion battery cathodes. Nat. Commun. 12, 5267. doi:10.1038/s41467-021-25610-7
Hamani, D., Ati, M., Tarascon, J. M., and Rozier, P. (2011). NaxVO2 as possible electrode for Na-ion batteries. Electrochem. Commun. 13, 938–941. doi:10.1016/j.elecom.2011.06.005
Huang, X.-Q., Li, D.-L., Huang, H.-J., Jiang, X., Yang, Z.-H., and Zhang, W.-X. (2021). Fast and highly reversible Na+ intercalation/extraction in Zn/Mg dual-doped P2-Na0.67MnO2 cathode material for high-performance Na-ion batteries. Nano. Res. 14 (10), 3531–3537. doi:10.1007/s12274-021-3715-2
Huang, Y., Fang, C., Zeng, R., Liu, Y.-J., Zhang, W., Wang, Y.-J., et al. (2017). In-situ formed hierarchical metal-organic flexible cathode for High-Energy Sodium-Ion Batteries. Chemsuschem 10, 4704–4708. doi:10.1002/cssc.201701484
Jamil, S., Feng, Y., Fasehullah, M., Ali, G., Wu, B., Guo, Y., et al. (2023). Stabilizing anionic redox in Mn-rich P2-type layered oxide material by Mg substitution. Chem. Eng. J. 471, 144450. doi:10.1016/j.cej.2023.144450
Jean, P., Parant, R. O., Michel, D., Fouassier, C., and Hagenmuller, P. (1971). Sur quelques nouvelles phases de formule NaxMnO2(x<1). J. Solid State Chem. 3 (1), 1–11. doi:10.1016/0022-4596(71)90001-6
Jiang, J., Hu, M., Zhang, D., Yuan, T., Sun, W., Xu, B., et al. (2014). Transition metal oxides for high performance sodium ion battery anodes. Nano Energy 5, 60–66. doi:10.1016/j.nanoen.2014.02.002
Jiang, J. S., He, H. C., Cheng, C., Yan, T. N., Xia, X., Ding, M. L., et al. (2022). Improving structural and moisture stability of P2-layered cathode materials for sodium-ion batteries. ACS Appl. Energy Mater 5 (1), 1252–1261. doi:10.1021/acsaem.1c03656
Jo, I. -H., Ryu, H. -S., Gu, D.-G., Park, J. -S., Ahn, I. -S., Ahn, H. -J., et al. (2014). The effect of electrolyte on the electrochemical properties of Na/ɑ-NaMnO2 batteries. Mat. Res. Bull. 58 (4), 74–77. doi:10.1016/j.materresbull.2014.02.024
Komaba, S., Takei, C., Nakayama, T., Ogata, A., and Yabuuchi, N. (2010). Electrochemical intercalation activity of layered NaCrO2 vs. LiCrO2. LiCrO2. Electrochem. Commun. 12, 355–358. doi:10.1016/j.elecom.2009.12.033
Kong, W., Gao, R., Li, Q., Yang, W., Yang, J., Sun, L., et al. (2019). Simultaneously tuning cationic and anionic redox in a P2-Na0.67Mn0.75Ni0.25O2 cathode material through synergic Cu/Mg co-doping. J. Mat. Chem. A 7 (15), 9099–9109. doi:10.1039/C9TA00968J
Kouthaman, M., Arjunan, P., Kannan, K., Priyanka, V., Subadevi, R., Kumaran, V., et al. (2020). Enhancing structural stability of layered O3-type Na-Mn-Ni-Cu-O cathode material through copper substitution for sodium batteries. J. Taiwan Inst. Chem. E 117, 86–92. doi:10.1016/j.jtice.2020.11.032
Kumar, V. K., Ghosh, S., Biswas, S., and Martha, V. -K. (2021). P2-Type Na0.67Mn0.5Fe0.5O2 synthesized by solution combustion method as an efficient cathode material for sodium-ion batteries. J. Electrochem. Soc. 168 (3), 030512. doi:10.1149/1945-7111/abe985
Kundu, D., Talaie, E., Duffort, V., and Nazar, L. (2015). The emerging chemistry of sodium ion batteries for electrochemical energy storage. Angew. Chem. Int. Ed. 54 (11), 3431–3448. doi:10.1002/anie.201410376
Le, N., Huynh, N., An, L., Nguyen, D., Nguyen, T., and Nguyen, H. V. (2021). Effect of 3d metal on electrochemical properties of sodium intercalation cathode P2-NaxMe1/3Mn2/3O2 (M = Co, Ni, or Fe). J. Chem. 1, 2–9. doi:10.1155/2021/2680849
Li, Z.-Y., Zhang, J., Gao, R., Zhang, H., Hu, Z., and Liu, X. (2016). Unveiling the role of Co in improving the high-rate capability and cycling performance of layered Na0.7Mn0.7Ni0.3-xCoxO2 cathode materials for sodium-ion batteries. ACS Appl. Mater Interf. 8 (24), 15 439–515 448. doi:10.1021/acsami.6b04073
Liu, Q.-N., Hu, Z., Chen, M.-Z., Zou, C., Jin, H.-L., Wang, S., et al. (2020). The cathode choice for commercialization of sodium-ion batteries: layered transition metal oxides versus prussian blue analogs. Adv. Funct. Mater 30 (14), 1909530. doi:10.1002/adfm.201909530
Lv, Z., Ling, M., Yue, M., Li, X.-F., Song, M.-M., Zheng, Q., et al. (2020). Vanadium-based polyanionic compounds as cathode materials for sodium-ion batteries: toward high-energy and high-power applications. J. Energy Chem. 55, 361–390. doi:10.1016/j.jechem.2020.07.008
Mendiboure, A., Delmas, C., and Hagenmuller, P. (1985). Electrochemical intercalation and deintercalation of NaxMnO2 bronzes. J. Solid State Chem. 57 (3), 323–331. doi:10.1016/0022-4596(85)90194-X
Niu, Y., Zhao, Y., and Xu, M. (2023). Manganese-based polyanionic cathodes for sodium-ion batteries. Carbon Neutralization 2 (2), 150–168. doi:10.1002/cnl2.48
Noha, S., Siham, D., Kazuki, H., Shinichi, K., Khalil, A., Abderrahim, S., et al. (2017). Layered P2-Na2/3Co1/2Ti1/2O2 as a high-performance cathode material for sodium-ion batteries. J. Power Sources 342, 998–1005. doi:10.1016/j.jpowsour.2017.01.025
Pang, W.-L., Guo, J.-Z., Zhang, X.-H., Fan, C.-Y., Nie, X.-J., Yu, H.-Y., et al. (2019). P2-type Na2/3Mn1/2Co1/3Cu1/6O2 as advanced cathode material for sodium-ion batteries: electrochemical properties and electrode kinetics. J. Alloys Compd. 790, 1092–1100. doi:10.1016/j.jallcom.2019.03.257
Parant, J. -P., Olazcuaga, R., Devallete, M., Fouassier, C., and Hagenmuller, P. (1971). Sur quelques nouvelles phases de formule NaxMnO2 (x-1). J. Solid State Chem. 3 (1), 1–11. doi:10.1016/0022-4596(71)90001-6
Qian, J., Wu, X., Cao, Y., Ai, X., and Yang, H. (2013). High capacity and rate capability of amorphous phosphorus for sodium ion batteries. Angew. Chem. Int. Ed. 52, 4633–4636. doi:10.1002/anie.201209689
Qin, M. L., Yin, C. Y., Xu, W., Liu, Y., Wen, J. H., Shen, B., et al. (2021). Facile synthesis of high capacity P2-type Na2/3Fe1/2Mn1/2O2 cathode material for sodium-ion batteries. Trans. Nonferrous Metal Soc. China 7 (31), 2074–2080. doi:10.1016/S1003-6326(21)65639-X
Rai, A. K., Anh, L. T., Gim, J., Mathew, V., and Kim, J. (2013). Electrochemical properties of NaxCoO2 (x∼0.71) cathode for rechargeable sodium-ion batteries. Ceram. Int. 40 (1), 2411–2417. doi:10.1016/j.ceramint.2013.08.013
Sabi, N., Doubaji, S., Hashimoto, K., Komaba, S., Amine, K., Solhy, A., et al. (2017). Layered P2-Na2/3Co1/2Ti1/2O2 as a high-performance cathode material for sodium-ion batteries. J. Power Sources 342, 998–1005. doi:10.1016/j.jpowsour.2017.01.025
Schulze, T. F., Häfliger, P. S., Niedermayer, C., Mattenberger, K., Bubenhofer, S., and Batlogg, B. (2008). Direct link between low temperature magnetism and high temperature sodium order in NaxCoO2. Phys. Rev. Lett. 100 (2), 026407. doi:10.1103/PhysRevLett.100.026407
Shibata, T., Kobayashi, W., and Moritomo, Y. (2014). Sodium ion diffusion in layered NaxMnO2(0.49 ≤x≤ 0.75): comparison with NaxCoO2. Appl. Phys. Express 7 (6), 067101. doi:10.7567/APEX.7.067101
Siriwardena, D. P., Fernando, J. F. S., Wang, T., Firestein, K. L., Zhang, C., Treifeldt, J. E., et al. (2019). Effect of Fe3+ for Ru4+ substitution in disordered Na1.33Ru0.67O2 cathode for sodium-ion batteries: structural and electrochemical characterizations. Electrochimica Acta 325, 134926. doi:10.1016/j.electacta.2019.134926
Slater, M.-D., Kim, D., Lee, E., and Johnson, C.-S. (2013). Correction: sodium-Ion batteries. Adv. Funct. Mater 23 (26), 3255–3958. doi:10.1002/adfm.201301540
Su, D., Wang, C., Ahn, H. -J., and Wang, G. (2013). Single crystalline Na0.7MnO2 nanoplates as cathode materials for sodium-ion batteries with enhanced performance. Chemistry 19 (33), 10884–10889. doi:10.1002/chem.201301563
Sui, Y., Hao, Y., Zhang, X., Zhong, S., Wu, L., Li, J., et al. (2019). Spray-drying synthesis of P2-Na2/3Fe1/2Mn1/2O2 with improved electrochemical properties. Adv. Powder. Technol. 31 (1), 190–197. doi:10.1016/j.apt.2019.10.010
Tamaru, M., Wang, X., Okubo, M., and Yamada, A. (2013). Layered Na2RuO3 as a cathode material for Na-ion batteries. Electrochem. Commun. 33, 23–26. doi:10.1016/j.elecom.2013.04.011
Wang, H., Li, L., Han, W., Guo, H., Sun, L., and Liu, X. (2020c). Unraveling the roles of La substitution for different transition metals on P2-Na0.67Mn0.6Ni0.2Co0.2O2 cathode materials. J. Electrochem. Soc. 167 (16), 160506. doi:10.1149/1945-7111/abca70
Wang, L., Wang, Y., Zhao, J., Li, Y., Wang, J., and Yang, X. (2019). Nb5+-doped P2-type Mn-based layered oxide cathode with an excellent high-rate cycling stability for sodium-ion batteries. Ionics 25, 4775–4786. doi:10.1007/s11581-019-03035-z
Wang, L., Zhang, X., Yang, X., Wang, Ji., Deng, J., and Wang, Y. (2020b). Co3O4-modified P2-Na2/3Mn0.75Co0.25O2 cathode for Na-ion batteries with high capacity and excellent cyclability. J. Alloy. Comp. 832, 154960. doi:10.1016/j.jallcom.2020.154960
Wang, Q., Chu, S.-Y., and Guo, S.-H. (2020a). Progress on multiphase layered transition metal oxide cathodes of sodium ion batteries. Chin. Chem. Lett. 31 (9), 2167–2176. doi:10.1016/j.cclet.2019.12.008
Wang, S., Chen, F., He, X. D., Zhang, L. M., Chen, F., Wang, J. R., et al. (2021b). Self-template synthesis of NaCrO2 submicrospheres for stable sodium storage. ACS. Appl. Mat. Interf. 13, 10 12203–12210. doi:10.1021/acsami.0c23069
Wang, S., Wang, L., Zhu, Z., Hu, Z., Zhao, Q., and Chen, J. (2014). All organic sodium-ion batteries with Na4C8H2O6. Angew. Chem. 53, 5892–5896. doi:10.1002/anie.201400032
Wang, S., Xia, L., Yu, L., Zhang, L., Wang, H.-H., and Lou, X.-W. (2016). Free-standing nitrogen-doped carbon nanofiber films: integrated electrodes for sodium-ion batteries with ultralong cycle life and superior rate capability. Adv. Energy Mater 6, 1502217. doi:10.1002/aenm.201502217
Wang, T.-Y., Su, D.-W., Shanmukaraj, D., Rojo, T., Armand, M., and Wang, G.-X. (2018b). Electrode materials for sodium-ion batteries: considerations on crystal structures and sodium storage mechanisms. Electrochem. Energy R. 1, 200–237. doi:10.1007/s41918-018-0009-9
Wang, T., Su, D., Shanmukaraj, D., Rojo, T., Armand, M., and Wang, G. (2018a). Electrode materials for sodium-ion batteries: considerations on crystal structures and sodium storage mechanisms. Electrochem. Energy Rev. 1, 200–237. doi:10.1007/s41918-018-0009-9
Wang, X., Yin, X. P., Feng, X. C., Li, Y., Dong, X. P., and Shi, Qi. H. (2021a). Superior cyclability of Ce-doped P2-Na0.67Co0.20Mn0.80O2 cathode for sodium storage. J. Phys. Chem. Solid 148, 130990. doi:10.1016/j.jpcs.2020.109750
Wang, Y., Zhao, F., Qian, Y., and Ji, H. (2018c). High-performance P2-Na0.70Mn0.80Co0.15Zr0.05O2 cathode for sodium-ion batteries. ACS. Appl. Mat. Interf. 10, 42380–42386. doi:10.1021/acsami.8b15693
Wei, F.-L., Zhang, Q.-P., Zhang, P., Tian, W.-Q., Dai, K.-H., Zhang, L., et al. (2021). Review-research progress on layered transition metal oxide cathode materials for sodium ion batteries. J. Electrochem. Soc. 168 (5), 050524. doi:10.1149/1945-7111/abf9bf
Wen, Y., He, K., Zhu, Y., Han, F., Xu, Y., Matsuda, I., et al. (2014). Expanded graphite as superior anode for sodium-ion batteries. Nat. Commun. 5, 4033. doi:10.1038/ncomms5033
Xiang, X.-D., Zhang, K., and Chen, J. (2015). ChemInform abstract: recent advances and prospects of cathode materials for sodium-ion batteries. Cheminform 46 (44), 5343–5364. doi:10.1002/adma.201501527
Xiao, Y., Zhu, Y.-F., Yao, H.-R., Wang, P.-F., Zhang, X.-D., Li, H., et al. (2019). A stable layered oxide cathode material for high-performance sodium-ion battery. Adv. Energy Mat. 9 (19), 1803978. doi:10.1002/aenm.201803978
Xu, J., Lee, D. H., Clément, R. J., Yu, X., Leskes, M., Pell, A. J., et al. (2014). Identifying the Critical Role of Li Substitution in P2-Nax[LiyNizMn1-y-z]O2 (0<x, y, z<1) Intercalation Cathode Materials for High-Energy Na-Ion Batteries. Chem. Mater 26 (2), 1260–1269. doi:10.1021/cm403855t
Yang, Y., and Wei, W.-F. (2020). Electrochemical mechanism of high Na-content P2-type layered oxides for sodium-ion batteries. Rare Met. 39, 332–334. doi:10.1007/s12598-020-01403-7
Yoda, U., Kubota, K., Kuroki, K., Suzuki, S., Yamanaka, K., Yaji, T., et al. (2020). Elucidating influence of Mg- and Cu-doping on electrochemical properties of O3-Nax [Fe,Mn]O 2 for Na-ion batteries. Small 16 (50), 2006483. doi:10.1002/smll.202006483
Yong, J.-F., Xin, Y.-Y., and Lou, X.-W. (2017). A practical high-energy cathode for sodium-ion batteries based on uniform P2-Na0.7CoO2 microspheres. Angew. Chem. Int. Ed. 129 (21), 5895–5899. doi:10.1002/ange.201702024
Zhang, Y., Zhao, F., Zhang, G., Yang, J., and Duan, L. F. (2020). Preparation of Na0.67Mn0.67Ni0.33-<i>x</i>Co<i>x</i>O2 as cathode material for sodium ion battery by multi-metal substitution and electrochemical performance. J. Mech. Eng. 56 (10), 117. doi:10.3901/JME.2020.10.117
Zhao, Q. Q., Butt, F. K., Yang, M., Guo, Z.-F., Yao, X.-Y., Zapata, M., et al. (2021b). Tuning oxygen redox chemistry of P2-type manganese-based oxide cathode via dual Cu and Co substitution for sodium-ion batteries. Energy Storage Mater 41, 581–587. doi:10.1016/j.ensm.2021.06.029
Zhao, X.-D., Fan, L.-Z., and Zhou, Z. (2021a). Cationic potential: an effective descriptor for rational design of layered oxides for sodium-ion batteries. Green Energy Environ. 6, 455–457. doi:10.1016/j.gee.2020.11.022
Zhu, P., Takeuchi, T., Ohta, H., Seo, W. S., and Koumoto, K. (2005). Preparation and Thermoelectric Properties of Na<I><SUB>x</SUB></I>CoO<SUB>2</SUB>/Co<SUB>3</SUB>O<SUB>4</SUB> Layered Nano-Composite. Mat. Trans. 46 (7), 1453–1455. doi:10.2320/matertrans.46.1453
Zhu, Y.-E., Zhu, Y.-E., Qi, X., Zhou, X., Zhang, X., Wei, J., et al. (2016). A P2-Na0.67Co0.5Mn0.5O2 cathode material with excellent rate capability and cycling stability for sodium ion batteries. J. Mat. Chem. A 4 (28), 11103–11109. doi:10.1039/C6TA02845D
Zhu, Y., Wang, B.-X., Gan, Q.-M., Wang, Y.-F., Wang, Z.-Y., Xie, J.-W., et al. (2019). Selective edge etching to improve the rate capability of prussian blue analogues for sodium ion batteries. Inorg. Chem. Front. 6, 1361–1366. doi:10.1039/c9qi00090a
Keywords: sodium-ion battery, cathode material, layered transition-metal oxides, electrochemical performance, energy storage
Citation: Lu J, Zhang J, Huang Y, Zhang Y, Yin Y and Bao S (2023) Advances on layered transition-metal oxides for sodium-ion batteries: a mini review. Front. Energy Res. 11:1246327. doi: 10.3389/fenrg.2023.1246327
Received: 24 June 2023; Accepted: 29 August 2023;
Published: 07 September 2023.
Edited by:
Tingting Zhu, University of Twente, NetherlandsReviewed by:
Yu-Bin Niu, Southwest University, ChinaCopyright © 2023 Lu, Zhang, Huang, Zhang, Yin and Bao. This is an open-access article distributed under the terms of the Creative Commons Attribution License (CC BY). The use, distribution or reproduction in other forums is permitted, provided the original author(s) and the copyright owner(s) are credited and that the original publication in this journal is cited, in accordance with accepted academic practice. No use, distribution or reproduction is permitted which does not comply with these terms.
*Correspondence: Shuo Bao, YmFvc2h1bzE5ODlAMTYzLmNvbQ==
Disclaimer: All claims expressed in this article are solely those of the authors and do not necessarily represent those of their affiliated organizations, or those of the publisher, the editors and the reviewers. Any product that may be evaluated in this article or claim that may be made by its manufacturer is not guaranteed or endorsed by the publisher.
Research integrity at Frontiers
Learn more about the work of our research integrity team to safeguard the quality of each article we publish.