- 1Joint BioEnergy Institute, Lawrence Berkeley National Laboratory, Emeryville, CA, United States
- 2Sandia National Laboratories, Livermore, CA, United States
- 3Biological Systems and Engineering Division, Lawrence Berkeley National Laboratory, Berkeley, CA, United States
Ionic liquids (ILs) are seen as a more sustainable alternative to volatile organic solvents that are accelerating innovations in many industries such as energy storage, separations, and bioprocessing. The ability to effectively deconstruct lignocellulosic biomass is a significant hurdle in the biorefining/bioprocessing industry and presents limitations towards the commercial production of bioproducts (such as biofuels, biomaterials, etc.). Certain ILs have been shown to promote effective lignin removal, cellulose recovery, and sugar yields from various biomass feedstocks such as corn stover, wheat straw, sugarcane bagasse, sorghum, switchgrass, miscanthus, poplar, pine, eucalyptus, and certain mixtures of municipal solid waste. However, these improvements are often counteracted by the limited biocompatibility of ILs, which results in an IL-induced reduction in enzyme activity and stability—an important downstream step in the conversion of biomass to biofuels/bioproducts. As a result, significant efforts have been made to discover and engineer compatible enzyme-IL systems and to improve our understanding on the effect that these ILs have on these systems. This review seeks to examine the impact of ionic liquids on enzymes involved in lignocellulosic biomass deconstruction, with a specific focus on their relevance in the context of pretreatment. Beyond presenting an overview of the ionic liquid pretreatment landscape, we outline the main factors that influence enzyme activity and stability in the presence of ILs This data is consolidated and analyzed to apply this body of knowledge towards new innovations that could lead to improvements in the processing of biomass to biofuels and bioproducts.
1 Introduction
Biofuels and bioproducts that are generated from lignocellulosic biomass offer numerous advantages for creating low cost, low net-carbon emission fuels and bioproducts, which are necessary to decarbonize our transportation industry and further a bio-based economy (Bhutto et al., 2016; OECD, 2019). Lignocellulosic biomass sources encompasses a wide range of plant-based materials such as agricultural residues (e.g., corn stover, sorghum stover, wheat straw), forestry residues, dedicated energy crops (e.g., switchgrass, miscanthus), and municipal solid waste (paper, cardboard, food scraps) that are abundant, accessible, and in some cases, advantageous to remove from their original environments (Barcelos et al., 2021). Furthermore, lignocellulosic biomass is renewable such that sustainably utilizing biomass as a feedstock may mitigate concerns about resource scarcity and dependence on non-renewable energy sources Adewuyi, 2022; Yadav et al., 2023; Jeswani et al., 2020).
Unlike first generation biofuels, which are typically grown on arable lands dedicated to food production, lignocellulosic biofuel crops can be responsibly extracted from forests and natural environments as well as cultivated from lands that are unsuitable for food production. In turn, these approaches ensure that lignocellulosic biofuel crops can be grown without encroaching on valuable agricultural resources-allowing us to meet our energy needs sustainably while maintaining food security. Another significant advantage of lignocellulosic biomass lies in its potential to minimize carbon emissions. When processed into biofuels or bioproducts, lignocellulosic biomass offers a more carbon-neutral relative to other fuel sources or even in some cases, a carbon-negative profile. Lignocellulosic-based bioproduction pathways are also incredibly versatile. Not only can these sources be converted to different fuels such as bioethanol, biodiesel, and jet fuel, they can also be converted into a variety of other products such as bioplastics, construction materials, food additives, dyes, and a host of other biochemicals. This versatility enhances the potential to meet our energy needs from a variety of industrial sectors as well as facilitate the transition to a bio-based economy.
Bioproducts derived from lignocellulosic biomass depend on the efficient chemical transformation of key biopolymers, namely, cellulose, hemicellulose, and lignin into targeted intermediates such as simple sugars and aromatics for their subsequent bioconversion into bioproducts (Sorek et al., 2014). However, lignocellulosic biomass tends to be highly recalcitrant and therefore, requires highly efficient deconstruction processes before downstream bioconversion can be achieved (Zoghlami and Paës, 2019). Biomass pretreatment is a broad term for a process that deconstructs lignocellulosic biomass such that it is more amenable for downstream enzymatic hydrolysis and bioconversion (Li et al., 2010). The pretreatment of lignocellulosic biomass is an important preliminary step that could include lignin removal and/or depolymerization, polysaccharide depolymerization or structural modification along with minor chemical reactions and separation processes with the overarching goal of enhancing the availability of biopolymers to enzymes, the rate of enzymatic hydrolysis to produce monomeric sugars, and improving the overall sugar yields. Regardless of the process involved, pretreatment is usually, but not always, done prior to the introduction of enzymes due to the limited effectiveness of enzymes on raw/native biomass (Souza et al., 2016; Sun et al., 2017a; Soltanian et al., 2020).
It is important to note that all of the pretreatment methods discussed in Table 1, including ionic liquid pretreatment, do not convert the majority of the cellulose to glucose or smaller oligomers. ILs tend to make lignocellulose more accessible to further depolymerization and in some cases separates the lignin from the cellulose and hemicellulose. However, a depolymerization method is still needed to refine the biomass. Of these depolymerization methods, enzyme mediated depolymerization is gaining traction as a viable commercial scale method (Birikh et al., 2022; Liu et al., 2021; Dhawane et al., 2022; Climent Barba et al., 2022). A primary advantage of using enzyme catalyzed processing of any material, including biomass is the lack of off target products. For instance, Acid/base conversion of cellulose can produce unwanted side products, such as hydroxymethylfurfural (Carvalheiro et al., 2008; Binder and Raines, 2010). Additionally enzymes have the ability to conduct reactions at speeds orders of magnitude without them and sometimes faster than compared to traditional chemical or thermal methods. Enzymatic processes are able to uphold these fast reaction rates while operating under milder conditions, such as lower temperatures and atmospheric pressure, which can reduce energy input costs (Yang et al., 2011; Haldar et al., 2016). Enzymatic processes are also versatile among a variety of different biomass feedstocks including low-value feedstocks that would otherwise be discarded or underutilized (Bhardwaj et al., 2021; Liu and Qu, 2021; Qiao et al., 2022). Finally, enzymes are reusable catalysts and are biologically derived and generally environmentally friendly. Using enzymes comes with some disadvantages, such as their expensive production. Additionally enzymes often operate under tight pH or temperature windows, which could be incompatible with other processes and require adjustments at various points of the process (Abdulsattar et al., 2020). Also, despite being reusable, it can sometimes be challenging to recover the enzymes in order to reuse them (Jørgensen and Pinelo, 2017).
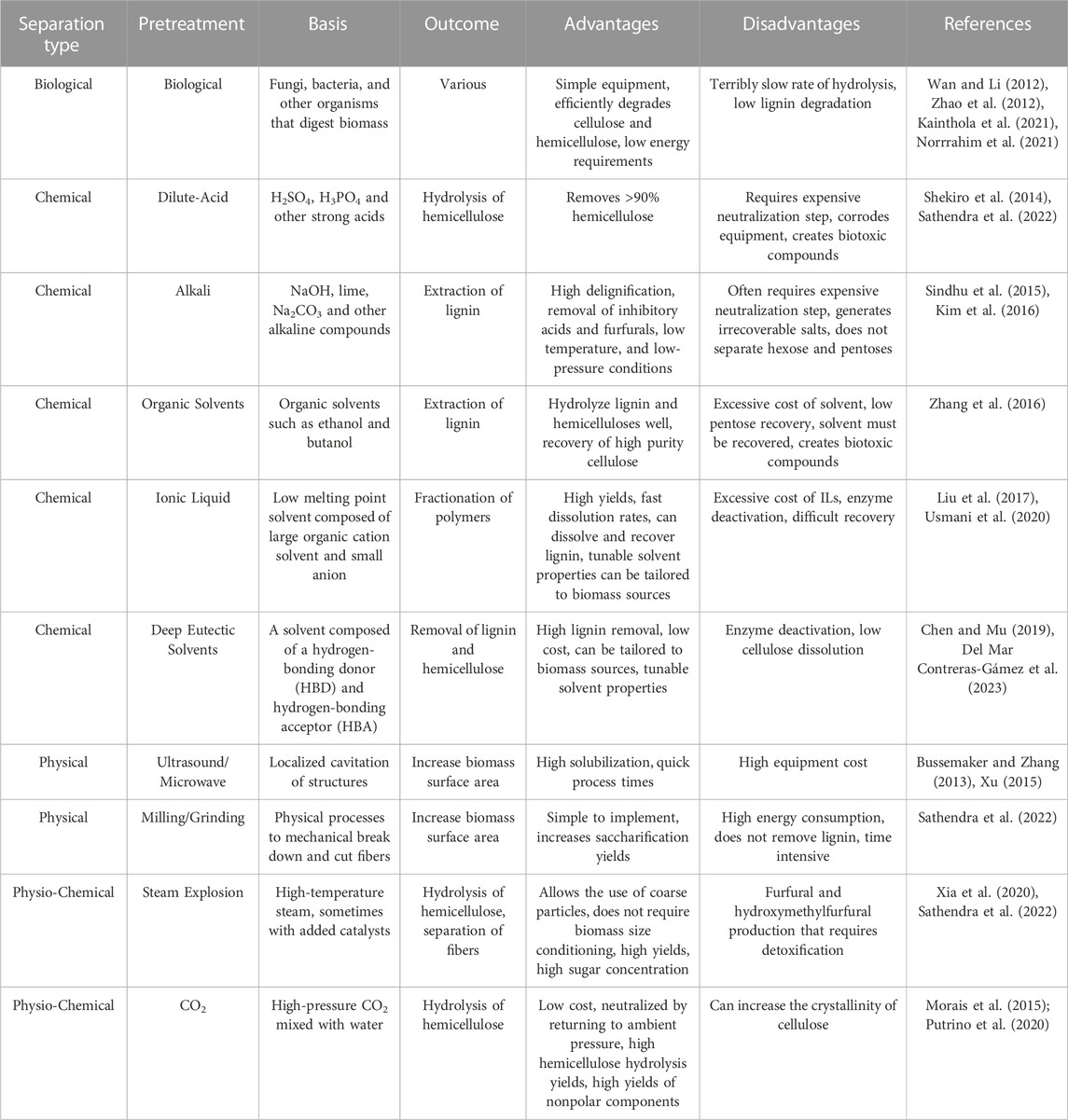
TABLE 1. Non-exhaustive list and descriptions of commonly used pretreatment methods for lignocellulosic biomass.
Figure 1A depicts a generalized process flow of lignocellulosic biofuel production. First, raw biomass consisting mostly of cellulose, hemicellulose and lignin is pretreated whereby a variety of different physical and chemical methods can be employed to disrupt the biomass structure to i) enhance the energy utilization efficiency of the biomass and ii) increase the bioconversion rates. Pretreatment is typically done prior to enzymatic hydrolysis because it can increase the availability of biopolymers in forms that are convertible by enzymes. Likewise, the conditions required in many pretreatment methods are antagonistic to enzyme function. While the main purpose of enzymatic hydrolysis in biofuel production is to convert cellulose, hemicellulose, and sometimes lignin to intermediary bioproducts that are digestible by organisms and enzymes downstream, other valuable bioproducts can be produced in tandem. Often the final bioconversion step will be to create a biofuel (like ethanol or butanol) via microbial fermentation but a plethora of other bioproduct production pathways have been explored (Østby et al., 2020; van den Heuvel et al., 2001; Costa et al., 2020).
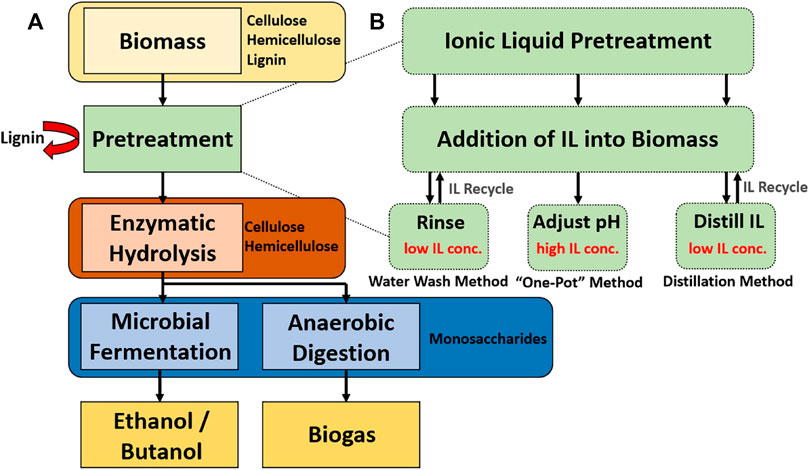
FIGURE 1. (A) Enzymatic biofuel production process chart highlighting mass balance of biopolymers (B) Comparison of “one-pot” and early separation ionic liquid pretreatment methods. The yellow arrows depict the flow of ionic liquids through the process, and the black arrows depict the flow of biomass.
At present, there exists a variety of different pretreatment methods that utilize mechanical separation (e.g., grinding, milling, sonication), chemical reactions (e.g., addition of acids/bases, oxidizing agents, ionic liquids, organics solvents, etc.), physicochemical methods (e.g., steam explosion, hydrothermolysis) and biological decomposition. A non-exhaustive list of common pretreatment methods is shown in Table 1. Each method has distinct advantages and disadvantages with regards to process time, energy input, efficacy of removing lignin and depolymerizing polysaccharides amongst other factors. These factors, discussed briefly in Table 1, play a critical role in selecting the most suitable pretreatment approach based on the specific requirements and characteristics of the biomass feedstock, as well as the desired outcomes of the subsequent conversion processes. More comprehensive discussion on the relative advantages and disadvantages of each pretreatment are analyzed elsewhere in literature (Silveira et al., 2015; Ramos et al., 2020).
Amongst these methods, the use of ionic liquids (ILs) for biomass pretreatment shows particular promise because they are seen as a versatile, environmentally friendly option that is able to selectively and efficiently degrade cellular structures, as well as extract lignin, which leads to high bioconversion rates and enables the formation of several value added compounds (Nanda et al., 2014; Hou et al., 2017; Leynaud Kieffer Curran et al., 2022). Ionic liquids can be used in conjunction with a variety of different methods of pretreatment to have collaborative effects on biomass breakdown.
For context, ionic liquids are salts with melting points below 100°C that are often liquids at room temperature and are composed of an organic cation and inorganic anion (Chiappe and Pieraccini, 2005; Lei et al., 2017). Despite high Coulombic forces between the ion pairs, the alkyl groups of each are often asymmetric, which prevents ionic bonding and allows ILs to be liquid at room temperature (Chiappe and Pieraccini, 2005). Substitution of different anion/cation pairs can change the polarity of the IL, and as a result, ILs can be custom designed to have specific properties (Chiappe and Pieraccini, 2005). Pertinent to this discussion, substitution of different alkanolammonium cations into acetate based ionic liquids have been shown to adjust the heat of vaporization, and different cation substitutions in acetate based ionic liquids have been shown to affect viscosity (Fendt et al., 2011). Many ionic liquids have an ability to enable chemical interactions between complex biopolymers and macromolecules that has made their use increasingly common in modern biomass processing and bioenergy production pathways (Das et al., 2021).
One of the principal advantages afforded by IL pretreatment is their enhanced selectivity for extracting and fractionating specific macromolecules in biomass (Silveira et al., 2015). For instance, ILs with imidazolium cations have been studied as options for biomass pretreatment due to their effectiveness at dissolving cellulose and the ability to regenerate dissolved cellulose from solution with the addition of antisolvent (Vitz et al., 2009), Whereas ILs such as Cholinium lysinate ([Cho][lys]) and Ethanolamine acetate ([EOA][OAc]) which both have a relatively high capacity for lignin removal, have relatively low capacity for dissolving cellulose relative to imidazolium based ILs (Sun et al., 2017a; Barcelos et al., 2021). By extension, the ability to tailor which ILs are used during pretreatment presents a means to design highly customizable deconstruction pathways that can address the specific composition and characteristics of different biomass feedstocks and enable the targeted formation of value added compounds (Nanda et al., 2014; Hou et al., 2017; Leynaud Kieffer Curran et al., 2022).
Additionally, some ILs are seen as “green” alternatives to VOC’s and other solvents used during pretreatment. One reason is that some ILs have low vapor pressures which prevents them from evaporating into the atmosphere and reduces air pollution and exposure risks. ILs are seen as “recyclable” in the sense that there many different methods of either regenerating more ILs in-situ or recovering ILs by means of extraction, adsorption, distillation, or ion exchange so that they can be reused in subsequent pretreatment processes (Mai et al., 2014; Zhou et al., 2018; Achinivu et al., 2022). Likewise, many ILs have high thermal stabilities and low flammability which make them less susceptible to combustion and decomposition at higher temperatures that may be advantageous for processing biomass at elevated temperatures. Most importantly, many ILs are less biotoxic than their VOC counterparts which mitigates concerns about post-process decontamination and accidental environmental contamination (Messali, 2014). It is important to note, however, that not all ILs exhibit these qualities, and that there exists a plethora of ILs that are either toxic, flammable and/or have high vapor pressures (Smiglak et al., 2006; Zhao et al., 2007; Meine et al., 2010; Flieger and Flieger, 2020).
With numerous combinations for ILs comes varying effectivenesses at dissolving cellulose and/or lignin, depolymerizing hemicellulose, and weakening the structure of the lignocellulosic matrix. Nevertheless, the overarching goal is to improve conditions for downstream processes-ideally in a manner that is both commercially scalable and economically feasible at scale. In practice, ILs modify biomass such that enzymes can more efficiently access lignocellulosic biopolymers and catalyze reactions to convert biomass into simpler molecules (Sun et al., 2014; 2016). However, the conditions necessary to effectively deconstruct lignocellulosic matter during pretreatment are often not conducive to the activity and long term stability of many enzymes (Wahlström and Suurnäkki, 2015; Pedersen et al., 2019). Even low, residual concentrations of ionic liquids have been demonstrated to reduce the activity and stability of enzymes commonly used in commercial bioproduct processing. In more moderate concentrations, some ILs have been found to deactivate or permanently denature enzymes (Konda et al., 2014). Enzyme deactivation slows saccharification yields within batches and requires that additional enzymes be added to subsequent batches to maintain the hydrolysis rate, contributing significantly to end product cost. Enzyme costs vary per process but contribute 10%–49% to the total price of biofuels-making them one of the most expensive inputs (Klein-Marcuschamer et al., 2012; Konda et al., 2014; Baral and Shah, 2016; Davis et al., 2018; Barcelos et al., 2021). This has in turn engendered a need to find and engineer biocompatible ILs as well as IL-tolerant enzymes and organisms that can work synergistically with ILs for high efficiency, low cost biomass deconstruction and bioconversion.
In response to IL-induced inactivation of enzymes, many IL-based pretreatment methods opt to remove ILs from the mixture containing the pretreated biomass. This is done in a variety of diverse ways. After ILs are introduced into biomass and allowed to fractionate and separate the biopolymers, the resultant slurry is washed to remove the bulk of ionic liquids. The ionic liquids are then recovered and purified by various methods including membrane separation, chemical extraction, and adsorption, which make ILs reusable for subsequent batches of pretreatment (Brennan et al., 2010; Zhou et al., 2018). However, there are distinct disadvantages to washing. For one, it often requires significant amounts of water and secondly, large scale recovery processes tend to be energy intensive. Both factors have a significant contribution to input cost (Klein-Marcuschamer et al., 2011; Konda et al., 2014; Ovejero-Pérez et al., 2021). ILs also represent a non-insignificant added cost to pretreatment, such that a secondary goal of many IL based pretreatment methods is to recover and reuse as much solvent as possible in order to reduce process cost. Techno-economic assessments of biomass pretreatments and ionic liquid recovery processes suggest that IL losses as low as 1% can seriously alter process economics (Xu et al., 2016a; Sun et al., 2017c).
To address substantial costs of removing ILs from biomass via washing, methods involving in situ saccharification of cellulose in aqueous-ionic liquid solution have been developed, whereby both the pretreatment and saccharification process is performed in a single vessel containing ionic liquids (Kamiya et al., 2008; Sun et al., 2017a). This “One-Pot'' method forgoes the need for an expensive water/solvent washing and cellulose regeneration step and also facilitates process consolidation and intensification. It could also save energy by limiting additional heating and cooling steps between reactors. However, the stability and performance of enzymes is often altered by the high concentration of ILs present in one-pot solutions. Likewise, extraction of bioproducts from solution presents challenges of its own with regards to enzyme preservation and reuse. Given that the “one pot” method offers a potentially viable route for reducing the cost of the production of bioproducts at scale, it is critical to find systems of ILs and enzymes that are compatible (Konda et al., 2014; Zang et al., 2020; Zhao et al., 2022). Thus efforts have thus been made to develop biocompatible ionic liquids in tandem with engineering new strains of IL-tolerant organisms and enzymes that are compatible with this process (Dabirmanesh et al., 2015; Wolski et al., 2016).
Figure 1B shows a generalized diagram of each discussed IL recovery process and how they fit into the overall biofuel production process seen in Figure 1A. There are clear tradeoffs in benefits and process complications presented by each method. An ideal pretreatment process optimizes the tradeoffs between the ability to decompose biomass components into monomeric sugars, the cost of energy inputs and utilities, the ability to recover ionic liquids, and the ability to retain enzyme performance over time (Vasconcelos et al., 2020).
Key to understanding this complex problem is identifying process parameters that affect enzyme performance in ILs and quantifying the nature and extent of these effects. It is well known that the interaction between ILs and enzymes is dependent on many factors including, but not limited to: substrate, cation-anion pair, enzyme expression method, temperature, and pH. Given the sheer number of combinations of ILs, enzymes, and assay conditions, it is difficult to systematically test and optimize the pretreatment effectiveness of each combination. In this paper we focus on these interactions within the context of the overall process of ionic liquid pretreatment and subsequent enzymatic hydrolysis for a variety of different substrate types and report key findings across the subject area to identify target improvement areas that will facilitate cost reductions in future processes.
2 Biomass composition
The efficacy of enzymes in breaking down lignocellulosic biomass in the pretreatment process is not only dependent on the chemical composition of the substrates they interact with, but also the mechanical and crystalline structure of the biomass itself. To this end, there exists immense diversity in the makeup of different candidate feedstocks. In general, the compounds of interest in these feedstocks can be sorted into several broad categories: cellulose, hemicellulose, lignin, and extractives.
2.1 Cellulose
Cellulose is the most abundant polymer in lignocellulosic biomass, and typically constitutes 40%–60% of its dry weight (Sharma et al., 2017). Cellulose is predominantly present in the cellular walls and is composed of β-D-glucopyranose units linked via β-(1,4) glycosidic bonds. Each glucopyranosyl unit of cellulose contains three hydroxyl functional groups, which impart hydrophilic properties and reactivity to various agents. Long, unbranched chains of several hundred cellulose units form structures termed nanofibrils. Parallel chains of nanofibrils are tightly bound to each via intermolecular hydrogen bonding to form microfibrils (Robak and Balcerek, 2018). In bulk, these microfibrils constitute the basis of cellular walls. A hierarchical chart depicting the relative length scales of these constituent structures is shown in Figure 2. Within each fibrils there can be intervaled sections of amorphous cellulose structures (Ling et al., 2018). The extent in which cellulose within a material forms crystalline structures vs amorphous structures is known as the index of crystallinity, and is well known to affect the rate of enzymatic hydrolysis by cellulases (Yoshida et al., 2008). A significant negative correlation between enzymatic hydrolysis performances of various biomass sources and their crystallinity has been reported among literature as more crystalline regions have stronger hydrogen bonding networks that make them less accessible to enzymes (Li et al., 2014). Ionic liquids have been found to more easily penetrate biomass in less crystalline regions, likely because of the reduced energy to break inter-structural bonding (Ling et al., 2017). Cellulose chains with higher degrees of polymerization (DP) constrain more hydrogen bonds that increase the difficulty of enzymatic hydrolysis. Conversely, shorter chains of cellulose have weaker hydrogen-bond systems making these chains more accessible for enzymes (Hallac and Ragauskas, 2011; Meng et al., 2017). Naturally, cellulose fibrils tend to become embedded into a lignocellulosic matrix that provides additional barriers for enzymatic hydrolysis, as this matrix is structurally diverse and requires several different synergistic enzyme systems or high energy inputs to decompose (Zoghlami and Paës, 2019). The proportion of cellulose that depolymerizes into glucose is a key metric to determine the effectiveness of a deconstruction process.
2.2 Hemicellulose
Hemicelluloses are heterogenous biopolymers that contain various monosaccharide pentose and hexose subunits, most notably, xylose. A section of an exemplar xylan polymer is shown in Figure 3 along with common constituent hexose and pentose subunits. Hemicellulose usually constitutes 20%–35% of total dry weight of biomass, and tends to have the highest fractional composition in wood substrates compared to other biomass (Chandel et al., 2018). In general hemicelluloses have much lower degrees of polymerization than cellulose (Rodrigues Mota et al., 2018). The vast majority of hemicellulose is amorphous and forms mechanically weak structures. These weak bonding systems allow hemicelluloses to be readily hydrolyzed by dilute acids or bases, as well as hemicellulase enzymes (Isikgor and Becer, 2015). Hemicelluloses cross-link with cellulose fibrils and lignin via ferulic acid residues and in doing so create further barriers limiting the accessibility of hydrolytic enzymes inside of the lignocellulosic matrix (Balakshin et al., 2011). Often the addition of acids or some species of ILs can fragment a significant portion of hemicellulose polymers without the addition of specialized enzymes. The proportion of hemicellulose that decomposes into monomeric sugars is also a key metric to determine the effectiveness of a deconstruction process.
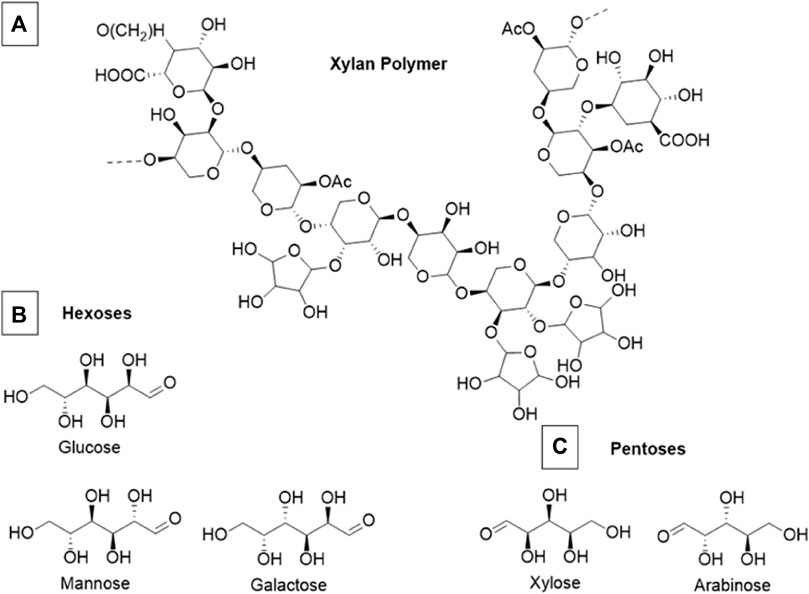
FIGURE 3. (A) Example section of a theoretical xylan polymer (B) Constituent monomeric hexoses commonly found in xylan (C) Constituent monomeric pentoses commonly found in xylan. This example polymer is primarily composed of xylose, arabinose, and glucose although the exact composition of xylan polymers is highly variable and species dependent.
2.3 Lignin
Lignin is the second most abundant lignocellulosic polymer, constituting roughly 15%–40% of the dry weight of biomass (Ragauskas et al., 2014). It forms an amorphous structure out of phenylpropanoid building units including p-coumaryl, coniferyl, and sinapyl alcohols, which are shown in Figure 4 (Agbor et al., 2011). Lignin is capable of binding hemicelluloses to cellulose in the cell wall and in doing so adds rigidity to the structure of cell walls. The high degree of heterogeneity of lignin structures typically makes it difficult for targeted enzymatic hydrolysis although the extent that lignin resists decay varies with species and tissue type. For example, lignin polymers with fewer aryl-aryl bonds such as syringyl (S) lignol are found to be more susceptible to decay vs guaiacyl (G) units, and thus the S/G ratio is a common metric of interest when determining the contents of biomass (Vane et al., 2006). Overall, the presence of lignin is well known to inhibit the conversion of cellulose as it is prevents enzyme access to cellulose, can non-specifically bind to cellulases, and is highly recalcitrant to decomposition itself, so it is often in the interest of biofuel processors to remove lignin from biomass prior to introducing enzymes (Santos et al., 2012).
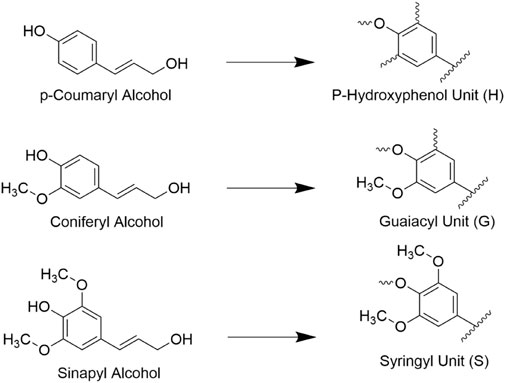
FIGURE 4. Three traditionally cited monolignols (hydroxycinnamyl acids) and the lignin units they form. These units form the basis of many lignin polymers.
2.4 Extractives
Extractives are the nonstructural components of lignocellulosic matter and include fats, proteins, phenolics, resins, waxes, along with many other trace organic compounds (Pecha and Garcia-Perez, 2020). Inorganic trace metals are known to decrease the yield of sugars from cellulose and change the properties of lignin products (Pecha and Garcia-Perez, 2020). Typically extractives can be removed by solvents, water or weak acids. They are also typically inhibitors of biofuel processing but can be used to generate valuable byproducts such as terpenes, proteins, triacylglycerides, and fatty acids including stearic acid, and linoleic acid.
3 Lignocellulosic enzymes
The complete enzymatic hydrolysis of cellulose to monomeric sugar units necessary for fermentation requires a combination of different enzymes working synergistically. Each enzyme targets different, and sometimes extremely specific bonds in biomass polymers. Lignocellulose degrading enzymes fit into several broad categories, and their hierarchical relationship is shown below in Figure 5. Further insight on cellulases, hemicellulases and some classes of LME’s is included in sections 3.1–3.3.
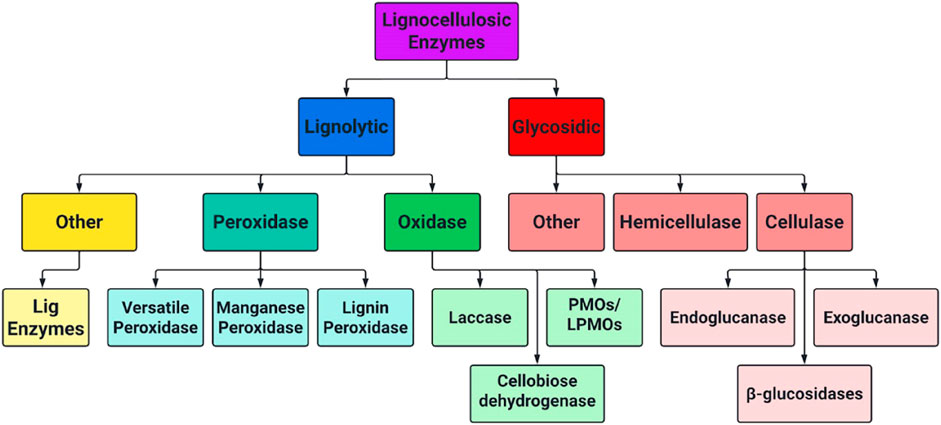
FIGURE 5. Hierarchical chart of lignocellulose degrading enzymes separated by lignolytic and glycosidic mechanisms.
3.1 Cellulases
Cellulases serve to break down cellulose molecules into monosaccharides and shorter oligosaccharides. Specifically, cellulases hydrolyze 1,4-beta-D-glycosidic linkages in cellulose and some hemicelluloses. The hydrolysis of cellulose is typically less thermodynamically favorable than that of starches because of the strong hydrogen bonding systems between cellulose units. There are several broad categories of cellulases-each differing structurally and mechanistically. A combination of these cellulases working synergistically can theoretically fully reduce cellulose into monosaccharides. Endoglucanases (EC 3.2.1.176) randomly cleave the β-1,4-glycosidic linkages of cellulose and xylan to create a reducing and non-reducing end (Chen and Wang, 2017). Reducing ends being characterized by the presence of aldehyde groups in place of a hydroxyl group. Exoglucanases (EC 3.2.1.91), which are also known as cellobiohydrolases and exocellulases, cleave off cellobiose units from the reducing or nonreducing end of a cellulose chain. β-glucosidases (EC 3.2.1.21), also known as cellobiases, hydrolyze the released cellobiose to glucose. Figure 6 shows a simplistic overview of the reduction of a cellulose polymer chain to glucose via these three enzymes. There are discrepancies in the specificity of specific enzymes in each category with regards to crystallinity, polymer length, and other ligno-carbohydrate interactions.
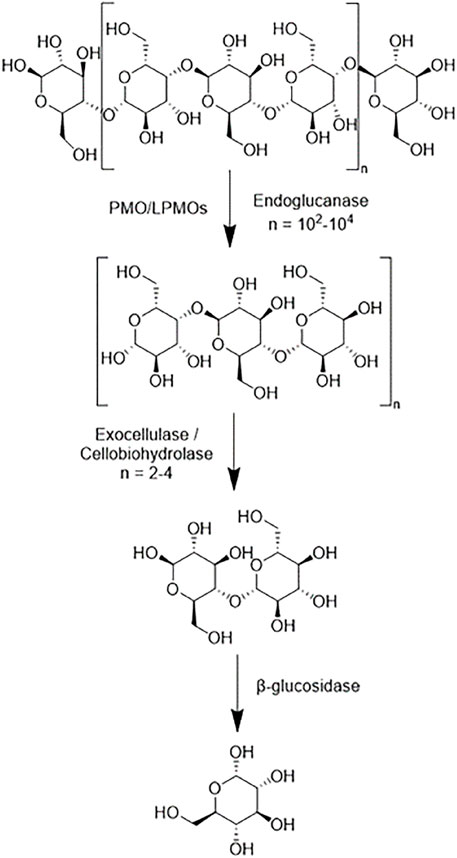
FIGURE 6. A simplistic scheme of the main sites of actions of different cellulases on cellulose polymer chains.
More recently, other cellulases such as oxidative cellulases, that use radical reactions to depolymerize cellulose, and cellulose phosphorylases that use phosphates rather than water as a reactant, have attracted interest in the academic community. These include (lytic) polysaccharide monooxygenases (PMOs/LPMOs) (EC 1.14.99.56) and cellobiose dehydrogenases (CDHs) (EC 1.1.99.18), which use oxidative cleaving to break apart cellulose. PMOs/LPMOs are enzymes that depend on copper ions as metal cofactors to oxidize the carbon-hydrogen bonds of polysaccharides (Hemsworth et al., 2014; Hedegård and Ryde, 2018). The enzyme reacts with a hydrocarbon substrate by employing O2 as a co-substrate and accepting two protons and two electrons. The copper ion aids in accepting electrons and oxygen atoms within the mechanism to ultimately produce a water molecule and an alcohol group attached to the previous hydrocarbon. The state of the copper ion is dependent on the stage of the mechanism. PMOs have varying degrees of substrate specificity which are dependent on their primary structure (Dimarogona et al., 2012). They are useful when using crystalline cellulose. The crystalline cellulose can help to produce new cellulose chain ends that can then be accessed by other enzymes. In non-crystalline cellulose, the endoglucanases can effectively produce enough new chain ends for the exo-glucanases.
3.2 Hemicellulases
Hemicellulases are a broad category of enzymes that participate in the breakdown and hydrolysis of galactans, xylans, mannans, and arabinans. Some of the most important hemicellulase categories are xylanase (EC 3.2.1.8) which hydrolyzes β-d xylano pyranosyl linkages of xylan to form xylo-oligosaccharides, β-mannanase (EC 3.2.1.78), arabinofuranosidase (EC 3.2.1.55), and β-xylosidases (EC 3.2.1.37) which also known as xylobiase, that catalyzes the hydrolysis of xylo-oligosaccharides into D-xylose sugars, although this is by no means an exhaustive list (Zhang et al., 2019).
3.3 Lignin modifying enzymes
Lignin modifying enzymes (LMEs) are a class of enzymes that catalyze lignin polymer deconstruction. Unlike most cellulases and hemicellulases, the most well-studied LMEs operate using oxidative processes that involve the formation of radical species rather than hydrolytic mechanisms. However, alternative reduction pathways in LMEs do exist (Lee et al., 2019; Chan et al., 2020). Radical chain reactions can cleave ether and carbon-carbon bonds-fracturing lignin as well as breaking down aromatic rings (Peng et al., 2002; Lange et al., 2013). A number of microorganisms, mainly white rot fungi (e.g., Trametes, Phanerochaete) and bacteria (e.g., Streptomycete, Pseudomonas, Rhodococcus) naturally produce enzymes capable of degrading and/or modifying lignin. Since lignin forms complex heterogeneous macromolecular structures, it is highly recalcitrant to degradation. In particular, its heterologous nature and large combination of structural bonds makes targeted reduction pathways ineffective. Among the most prominent categories of LMEs are laccases (EC 1.10.3.2), which can both polymerize and depolymerize lignin compounds via C-C cleavage, C oxidation, and alkyl-aryl cleavage, lignin peroxidases (EC 1.11.1.14), which targets the non-phenolic components of lignin (which can constitute up to 90% of lignin), manganese peroxidase (EC 1.11.1.13) which specifically targets phenolic components of lignin by catalyzing the reaction 2 Mn(II) + 2 H+ + H2O2 = 2 Mn(III) + 2 H2O, and versatile peroxidase (EC 1.11.1.16) which, as their name suggests, can target both phenolic and non-phenolic components of lignin (Youn et al., 1995; Martínez et al., 2005; Falade et al., 2017).
4 Factors affecting enzyme activity in ionic liquids
To develop more robust ionic liquid tolerant enzymes for biomass deconstruction and bioprocessing, key factors such as ionic liquid composition and concentration, temperature, pH, enzyme source, and expression method must be optimized. In subsequent sections we comprehensively discuss the roles of these influences in pretreatment processes and their respective IL-enzyme interactions that alter the properties of enzymes of interest.
4.1 Ionic liquids and relevant enzyme activity
The cation and anion structural components influence the properties of ILs, which can have significant impacts on the activity and stability of enzymes when utilized as a solvent medium. Several factors instigate different reactions from the enzymes including but not limited to structural dynamics, hydrophilicity/hydrophobicity, alkyl chain length of the cation, the hydrogen bond forming capabilities of the anion, rheological properties, co-solvents, and experimental conditions. With numerous variations in experimental, pretreatment conditions, and enzyme origin, it may be difficult to draw linear relationships between ionic liquid concentration and enzyme activity. Furthermore, trends in enzyme activity are investigated by classifying ionic liquids that share similar physicochemical properties into comparable groups where their deconstruction metrics are discussed below.
4.1.1 Imidazolium-based ionic liquids
As one of the most well-investigated classifications of ionic liquids, ILs with an imidazolium cation have been host to many experiments conducted to analyze their effects on various lignocellulosic enzymes. Imidazolium-based ionic liquids are synthesized starting with N-alkylimidazoles followed by the addition of an anion by means of a metathesis reaction, with the most common modifications being alteration of the N-alkyl substituent or anion. Ionic liquid cations such as 1-ethyl-3-methylimidazolium (EMIM), 1-butyl-3-methylimidazolium (BMIM), and 1-hexyl-3-methylimidazolium (HMIM), pictured in Figure 7A, are among the most prevalent N-imidazole cations studied in relevant literature. Across published works, interchangeable nomenclature for the aforementioned ionic liquids is utilized; for instance [EMIM]+ and [C2MIM]+ both refer to 1-ethyl-3-methylimidazolium just as [MMIM]+ and [DMIM]+ both refer to 1,3-dimethylimidazolium.
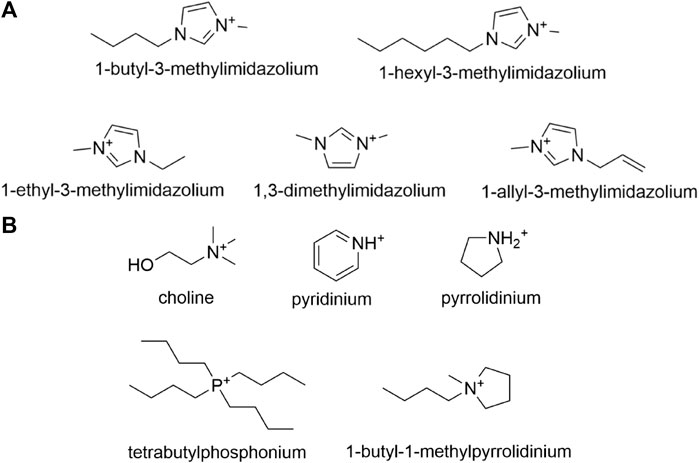
FIGURE 7. (A) Common N-alkylimidazole cations utilized in the synthesis of imidazolium-based ionic liquids, and (B) Other less common cations that are utilized in the synthesis of imidazolium-based ionic liquids.
Several enzymes were screened for their activity and stability in imidazolium-based ionic liquids. While imidazolium-based ionic liquids all share similar cationic structures, the size and variability of their respective anions largely alter their effects on enzymes. Anions well reported in literature include but are not limited to: acetate ([OAc]- also sometimes written as [Ac]-), chloride ([Cl]-), bromide ([Br]-), dimethyl phosphate ([DMP]-), diethyl phosphate ([DEP]-), and ethyl sulfate ([EtSO4]-). We have classified imidazolium-based ionic liquids into groups based on their anions, focusing primarily on acetate, halide, phosphate, and sulfate-based anions, as these ILs are commonly cited in literature and thoroughly studied relative to lignocellulosic biomass dissolution.
4.1.1.1 Imidazolium acetate ILs
Most enzymes tend to perform better in lower concentrations of ionic liquids, as higher concentrations destabilize or deactivate the enzyme. At lower concentrations of ILs many enzymes benefit from the IL-induced deconstruction of biomass that make cellulose chains more available, while not strongly affecting the function of the enzyme. Numerous reports of immobilized and other modified enzymes observe higher ranges of activity in increasing concentrations of ionic liquid, but no direct correlation between chemical modification and enzyme activity has been proven. Across all studies, there exists a general pattern of cellulolytic activity retention between 60% and 95% in 10%–20% of imidazolium-acetate based ionic liquids (see Table 2). In one study various thermophilic cellulases in 10% 1-ethyl-3-methylimidazolium ([EMIM][OAc]) were recorded to retain above 80% activity for most enzymes, with 70% of all enzymes being extremely tolerant to the ionic liquid (Gladden et al., 2014). Some of these enzymes even had activity in 40% IL ([EMIM][OAc]) greater than their activity in aqueous solutions. This is due to the thermophilic and rigid structure of these enzymes, and high IL concentrations can loosen up the structure of the enzyme, increasing their activity at lower temperatures, in lieu of higher temperatures in aqueous buffers.
Gunny et al. reported 95% relative activity of cellulases, compared to the control in 10% [EMIM][OAc] and also observe activity retention of ∼60% in 10% of [BMIM][OAc] (Gunny et al., 2014). Cellulases from Trichoderma reesei were observed to have activity maintained at 85% of its initial activity in 10%–40% [EMIM][OAc] after 6 h in the IL (Elgharbawy et al., 2016). A halophilic cellulase from Paenibacillus tarimensis in 20% [EMIM][OAc] was found to retain above 90% of its initial activity at both 50°C and 80°C, while a Pseudoalteromonas sp. cellulase retained above 80% activity in 15% of the same ionic liquid (Raddadi et al., 2013; Trivedi et al., 2013). Zhang et al. investigated T. reesei cellulases that only retain up to 60% of initial activity in 20% [EMIM][OAc] while more extremophilic cellulases from Halorhabdus utahensis retain above 100% relative activity in 20% of the same ionic liquid (Zhang et al., 2011). A mixture of fungal cellulases performed well following preincubation in 15% and 20% [EMIM][OAc], retaining 77% and 65% activity, respectively (Wang et al., 2011b). Ultimately, over 60% of initial activity is retained in lower concentrations of IL.
In ever lower concentrations, activity is further retained in several types of cellulases. Numerous studies of cellulase activity report relative activity to surpass 90% in 5% IL. Li et al. report 90% cellulase activity in 5% 1-(2-Hydroxyethyl)-3-methyl imidazolium acetate [C2OHMIM][OAc] following a 24 h incubation, which then decreased to 56% in activity in 25% IL under the same time. For a shorter incubation period of 1.5 h, they report 93% activity in 5% IL, and 72% activity in 25% IL (Li et al., 2012). Paenibacillus sp. LLZ1 cellulases with improved in situ saccharification in 5% IL/DMSO systems are reported, with 162% and 123% enhancements in activity in [EMIM][OAc] and [BMIM][OAc], respectively (Hu et al., 2016a).
Laccase activity is often maintained at a steady elevated level in moderate concentrations of imidazolium-acetate based ionic liquids. This of course cannot be used as a blanket statement for all experiments, as varying conditions and inhibition mechanisms will cause disparities in data that are difficult to explain. Laccase S1-20LAC from Staphylococcus arlettae S1-20 retained above 90% activity in 5% of all the ILs that were tested and 112.89% activity in 10% [EMIM][OAc] after a 3 h preincubation period (Chauhan et al., 2018). Following an incubation of 7 days, thermophilic Trametes versicolor laccase faced an 11% decrease from its initial activity in 15% [EMIM][OAc], a significant improvement compared to the 80% activity loss in buffer solution (Harwardt et al., 2014). Trametes KS-2 laccase retained above 90% activity in 2.5% [EMIM][OAc] while activity decreased to roughly 40% in 7.5% IL compared to the enzyme in 0% IL (Dong et al., 2019). Although laccase from Thermus thermophilus is extremely thermophilic, the enzyme experienced greater than a 50% loss in activity in concentrations as low as 2% [EMIM][OAc] (Stevens et al., 2020a; Stevens et al., 2020b). Although all the above laccases were tested in the same ionic liquid, there is an apparent disparity in the data which can be attributed to the difference in experimental conditions and/or origin of the enzyme. Many of these laccases are evaluated under wide ranges of pHs (4.5–9) and temperatures (28°C–85°C) and even those that operate under similar conditions vary greatly in activity ranges. Further, fungal enzymes and bacterial enzymes behave quite differently in ionic liquids as different inhibition/mediator mechanisms and glycosylation come into play as well as possible sampling errors (Longe et al., 2018; Zhu et al., 2020). Most laccases tested have been from fungal origin, although bacterial laccases have recently been discovered that show promise in potentially being only able to break some lignin bonds and not be able to form them as well (Zhu et al., 2020).
Xylanase activity in imidazolium-based ionic liquids is affected by the nature of the enzyme as well as the substrates it acts upon. Family GH10 and GH11 xylanases are amongst the most used xylanases in biomass hydrolysis, but behave differently in ionic liquids, due to variations in structure and thermostability. Xylanase from Thermopolyspora flexuosa GH10 was increasingly stable in high concentrations of [EMIM][OAc], facing a 54% increase in half-life in 15% of the IL. The activity of the enzyme was inhibited by [EMIM][OAc], but overall the enzyme remained stable during a 24 h incubation period in up to 35% of the IL (Anbarasan et al., 2017). Activity of Thermoascus aurantiacus SL16W GH10 xylanase was inhibited by varying concentrations of [EMIM][OAc] depending on the substrate concentration and its access to the active site. Xylanase performed at 47% relative activity compared to control in 25% IL, which decreased to 37% activity in 50% IL (Chawachart et al., 2014). Trichoderma longibrachiatum family 11 xylanases performed at around 70% relative activity in 20% [EMIM][OAc] in a non-incubated system, but above 100% relative activity in a substrate-free incubation system in the same concentration of IL. In both cases, enzyme activity was inhibited at high concentrations of IL (up to 50%). Following stabilization by an engineered N-terminal disulfide bridge, an extremophilic xylanase from Dictyoglomus thermophilum GH11 was inactivated almost completely in 25% [EMIM][OAc]. However, the enzyme did perform relatively better in higher temperatures (100°C–110°C) (Li et al., 2013). Due to the difference in kinetic parameters and substrate-based inhibition, it is difficult to note correlation between the types of xylanase and their performance in ionic liquids through experimentation.
Structural analysis and understanding of the mechanisms by which xylanases hydrolyze pretreated biomass may help gather a more holistic understanding of the hydrolytic behavior of xylanases. An experiment in which T. flexuosa GH10 xylanase and D. thermophilum GH11 were compared in various ionic liquids demonstrated high tolerance of GH10 xylanase to 25%–35% [EMIM][OAc], but lower tolerance of GH11 xylanases to even 15% of the IL (Hebal et al., 2020). GH10 xylanases generally tend to perform better than their GH11 counterparts, due to higher thermostability and structural advantage. GH10 xylanases are able to better access the xylan backbone of pretreated biomass, while GH11 xylanases are limited in this manner due to the influence of acetyl group substitution on the enzyme (Hu and Saddler, 2018). Table 2 summarizes the activity of various enzymes in imidazolium acetate-based ILs. The main interest in acetate based ILs is twofold: First, they are usually liquid at room temperature and second: they are generally able to dissolve cellulose while also supporting after dilution some degree of enzyme activity (Zhao et al., 2008; Du and Qian, 2011).
4.1.1.2 Imidazolium halide ILs
Cellulase activity in imidazolium-halide ionic liquids is notably more variable, as many studies report complete enzyme deactivation in even low concentrations of ILs, while others report high activity in up to 20% IL. Thermophilic cellulases from Aspergillus niger are reported to retain up to 70% activity in 5% [BMIM][Cl]. At higher concentrations, activity steadily decreases; it demonstrates 65% and 50% activity in 10% and 20% [BMIM][Cl] respectively (Salvador et al., 2010). Stachybotrys microspora cellulases in [BMIM][Cl] were found to retain 100% and 80% activity in 5% and 10% ionic liquid, respectively. However, the activity decreases as the concentration increases, to 50% at 20% IL (Ben Hmad et al., 2017). Unlike other cellulases, which tend to retain higher activity in even low concentrations of chloride-based ionic liquids, He et al. report a Galactomyces sp. cellulase that loses all activity in 10% and higher concentrations of [BMIM][Cl] (He et al., 2016).
Like the enzymatic behavior exhibited by laccases imidazolium-acetate based ionic liquids, laccases in the presence of imidazolium-halide based ILs perform varyingly based on enzymatic origin, expression, and experimental conditions. S1-20LAC performed exceptionally in [BMIM][Cl] [DMIM][Cl], and [EMIM][Br] following a 3 h preincubation period, retaining activity at 144.02%, 130.89%, and 99.55%, respectively (Chauhan et al., 2018). Laccases from Agaricus bisporus (LAB) and Trametes versicolor (LTV) were studied for their activity in varying concentrations of [BMIM][Br]. Enzyme activity for LAB increased 1.5-fold at 20% of the IL but was almost completely deactivated at 60% IL. Activity of LTV was increased twofold in 10% [BMIM][Br] but also inactive at IL concentrations above 70% (Shipovskov et al., 2008). A T. versicolor laccase demonstrated inhibition of activity in both [BMIM][Cl−] and [HMIM][Br−] but an increase in long term stability compared to the control in [BMIM][Cl−] (Domínguez et al., 2011). The disparity in the data can be attributed to differences in experimental conditions or the environmental preferences of the enzyme. According to the literature, it remains true that enzymes perform better in imidazolium-based ionic liquids with an acetate anion compared to a halide anion. This is likely due to the fact that the CH3COO− (OAc) anion is more kosmotropic (further discussion in section 4.1.3) in comparison to the Cl− anion, and acts as a stabilizing agent for the enzyme (Zhao et al., 2006). Table 3 outlines the enzymatic activity of various lignocellulosic enzymes in imidazolium halide-based ILs.
4.1.1.3 Imidazolium dialkyl-phosphate ILs
There is much variation in the results presented by studies that investigate cellulase activity in imidazolium-based ionic liquids with phosphate-based anions. While some report unusually low activity, as He and coworkers do in their study of a Galactomyces sp. cellulase, which loses all activity in 15% and higher concentrations of [DMIM][DMP](He et al., 2016), others report much higher activity. For instance, Elgharbawy et al. report cellulases that retain 100% activity in 10%–40% [DMIM][DMP], only losing 20% activity in 60% of the IL in the first 2 h of incubation (Elgharbawy et al., 2016). Further, a fungal cellulase from Fusarium oxysporum retains above 80% relative activity in two ionic liquids after 60 h [EMIM][DMP] being one of them (Xu et al., 2015b).
Xylanase E2 hydrolyzing p-nitrophenyl substrates maintained ∼86% activity in up to 20% [EMIM][OAc] and over 100% hydrolysis activity in low concentrations (5%) of the IL. Notably, the same enzymes maintained above 100% hydrolytic activity in 20% of both [DMIM][DMP] and [EMIM][DMP]. However, severe activity loss was noted in the presence of [EMIM][DEP], with almost a 97% activity loss in 20% of the IL (Thomas et al., 2011). Table 4 presents a summary of cellulase and xylanase activities in imidazolium phosphate-based ILs.
4.1.1.4 Imidazolium alkyl-sulfate ILs
Laccases are commonly evaluated in imidazolium-based ionic liquids with an ethyl-sulfate anion. Commercially available fungal laccases performed well in [EMIM][EtSO4], facing only up to ∼25% activity loss in 50% of the ionic liquid at pH 5 (Tavares et al., 2008). Chemically modified laccases by various means often display higher levels of stability in increased retention of activity compared to free enzymes, which is explored further in section 5.
Fungal peroxidases were studied in 5% [EMIM][EtSO4] and [EMIM][MDEGSO4] and reported to retain 86% and 75% residual activity following a 7-day incubation period at pH 7, respectively. Increasing the ionic liquid content resulted in inactivation of the enzyme, at high concentrations of 50% (Carneiro et al., 2009). Caldariomyces fumago peroxidases tolerated up to 30% [MMIM][MeSO4] and [BMIM][MeSO4], but ultimately performed best in 10% [MMIM][MeSO4] in terms of substrate conversion (Sanfilippo et al., 2004). Horseradish peroxidases faced uncompetitive inhibition in [BMIM][MESO4], resulting in an overall decrease in activity and measured kinetic parameters (Park et al., 2011). Table 5 consolidates data regarding peroxidase and laccase activity in various alkyl-sulfate based ILs.
4.1.2 Other ionic liquids
Aside from the previously discussed imidazolium based ILs, there are several other ILs that constitute different cations with varying pretreatment properties. Among these are choline, pyrrolidinium, pyridinium, and phosphonium based ionic liquids. The structures of the cations of these often-used ILs are depicted below in Figure 7B.
The chemical composition of cholinium-based ionic liquids comprises a choline cation and an anion often from an amino acid and can be synthesized by several methods–most commonly by neutralization reactions. Common choline-based ILs studied in literature include choline acetate [Cho][OAc] and choline butyrate [Cho][Bu], amongst others. Generally, choline-based ILs are successful in dissolving lignin and allowing lignocellulosic enzymes to retain elevated levels of activity. After 6 h in [Cho][OAc], microbial cellulase from palm kernel cake (PKC-Cel) retained >90% activity in 10%–20% IL, 85% activity in 40% IL, and 80% activity in 60%–80% IL. Activity of PKC-Cel in [Cho][Bu] after 6 h was maintained >80% in 10%–20% IL and <50% activity in 80%–100% IL. Compared to imidazolium-based ionic liquids tested in this study [Cho][OAc] and [Cho][Bu] allowed for highest stability of the cellulases (Elgharbawy et al., 2016). H. aswanesis lignin and manganese peroxidases as well as laccases were investigated in cholinium laureate based ILs, in which all three enzymes retained 433%–495% relative activity in 0.15 mM IL. Increasing the IL concentration did not further improve enzyme activity (Chauhan and Choudhury, 2020). Laccase from T. versicolor was inhibited in all but the lowest concentrations of cholinium lysinate, which acts as a mixed inhibitor (Stevens et al., 2019). Other T. versicolor laccases were deactivated by choline chloride (ChCl) based deep eutectic solvents (DES), in which they retained below 40% residual activity in 50% DES. Comparatively, better results were obtained with laccases in choline dihydrogen citrate (ChDHC) based DES, which increases relative activities to over 170% at concentrations of 25% and 50%. Toledo et al. attribute this increase in activity to the increased number of hydroxyl groups in the ChDHC based DES compared to the other studied solvents (Toledo et al., 2019). Based on existing literature and the apparent properties of cholinium based ILs, they serve as potential candidates for further use in lignocellulosic dissolution and pretreatment processes.
Another commonly used IL is the pyrrolidinium-based [BMPL][OTF], or 1-butyl-1-methylpyrrolidinium trifluoromethanesulfonate. Many reports that investigate imidazolium-based ILs also include studies with [BMPL][OTF] due to its comparable properties. Pseudoalteromonas sp. cellulases demonstrated 92.67% activity in [BMPL][OTF] and >80% in 15% of the IL (Trivedi et al., 2013). Compared to other imidazolium-based ILs, bacterial cellulase CelA10 was found to retain highest activity in 30% [BMPL][OTF] at roughly 1.8 U/mg, while other ILs allowed activity retention consistently below 1.4 U/mg (Pottkämper et al., 2009). T. versicolor laccases were destabilized in [BMPL][OTF] compared to other ILs, especially in higher concentrations and longer periods of time, due to its comparative kosmotropicity (Yu et al., 2013). This brings us to the discussion regarding the Hofmeister series, and comparative analysis of different conformations of ionic liquids and their respective effects on enzyme activity. An issue with using these ionic liquids for the purpose of lignocellulose conversion is that ions like OTF are not likely going to dissolve cellulose, as they cannot strongly accept hydrogen bonds and disrupt the cellulose structure that has one chain bonded to another. Regarding having functioning enzymes in the presence of cellulose dissolving ionic liquids there is a delicate balance of having enough hydrogen bond basicity to pretreat the cellulose, but not so much that it denatures the enzymes required to convert the cellulose to glucose or smaller oligomers.
4.1.3 Conformational changes of the ionic liquids
The ability to dissolve cellulose is mostly attributed to IL’s anions’ high H-bond basicity that allows them to bond strongly with the equatorial hydroxyl groups in cellulose (Remsing et al., 2008). Typically small anions with high H-bond basicities have been shown to be effective at dissolving cellulose, whereas aromatic cations with lower enzyme-interaction strengths have been shown to be the best for dissolving cellulose (Casas et al., 2012; Nordwald et al., 2014; Wahlström and Suurnäkki, 2015). While enzyme activity in ionic liquids is governed by a multitude of factors, conformational changes of the ionic liquids directly affect their ability to interact with the enzymes due to differences in kosmotropicity and chaotropicity. Kosmotropes are defined as ions that interact strongly with water, favoring its structure, while chaotropes break the structure of water through interactions (Zhao, 2006). Figure 8 orders both common cations and anions based on their relative kosmotropicity. Yang details the effects of kosmotropic versus chaotropic anions and cations on protein stability, in reference to their ranking in the Hofmeister series. They note that generally, enzymes tend to favor ionic liquids with chaotropic cations and kosmotropic anions due to their ability to interact strongly with water and stabilize the enzyme (Yang, 2009). As they experience increased hydrophobic hydration, cations with longer alkyl chains tend to be more kosmotropic, and thus more destabilizing towards the enzymes (Zhao, 2006). Generally, anions present dominating Hofmeister effects compared to cations, due to their greater polarizability in water (Yang, 2009). Across the reviewed literature, we note that the enzymes tend to follow this pattern and act in accordance with the Hofmeister series.
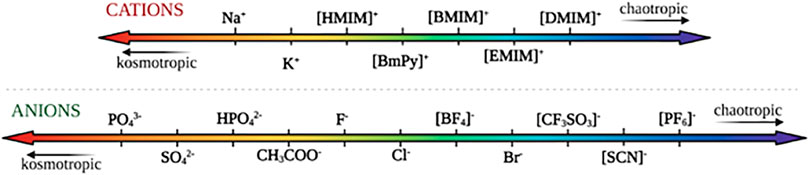
FIGURE 8. Commonly used cations and anions in ionic liquids, ranked according to their relative kosmotropicity/chaotropicity.
A notable trend amongst ILs with halide-based anions is the discernible increase in activity retention of cellulases in [EMIM][Cl] in comparison to [BMIM][Cl]. Zhang et al. report T. reesei cellulases that retain up to 60% activity in 20% [EMIM][Cl], but below 20% activity in the same concentration of [BMIM][Cl] and [AMIM][Cl] (Zhang et al., 2011). Johnson et al. reports higher activity of supercharged cellulases, with a positive surface charge, in 10% [EMIM][Cl]– around 75%– compared to activity in [BMIM][Cl] (Johnson et al., 2016). Raddadi et al. extensively investigated the performance of a halophilic Paenibacillus tarimensis cellulase in [BMIM][Cl] and [EMIM][Cl]. They reported the highest activity, nearly 90%, in 20% [EMIM][Cl] at two different temperatures and 85% activity in 20% [BMIM][Cl]. As the concentration of both ILs was increased, the activity decreased correspondingly (Raddadi et al., 2013). The larger alkylic substitute attributed to the [BMIM][Cl] cation is probable cause for the corresponding decrease in cellulase activity.
Similarly, a study done on fungal laccase activity in three ILs [C4mim][Cl] [C8mim][Cl], and [C10mim][Cl], demonstrated an inverse relationship between enzyme activity and alkyl chain length of the ionic liquid (Rodríguez et al., 2011). Feder-Kubis and Bryjak report a fungal laccase’s activity in five menthol based ionic liquids, finding that they ultimately performed better in 3-butyl1-[(1R,2S,5R)-(−)-menthoxymethyl]imidazolium and heptyl [(1R,2S,5R)-(−)-menthoxymethyl]dimethylammonium compared to 1-[(1R,2S,5R)-(−)-menthoxymethyl]-3-heptylimidazolium and decyl [(1R,2S,5R)-(−)-menthoxy-methyl]dimethylammonium, presumably due to their lengthened alkyl chains (Feder-Kubis and Bryjak, 2013). An engineered Bacillus HR03 laccase experienced destabilizing effects in ionic liquids with increasingly kosmotropic cations, as it retained highest activity in [EMIM][Cl], followed by [BMIM][Cl] and [HMIM][Cl] (Dabirmanesh et al., 2015). Trametes versicolor laccase, although was fairly stable at low concentrations of [EMIM][EtSO4-], was inhibited by the ionic liquid. However, it is worth noting that about 10 times the amount of its halide-based counterpart was required of this ionic liquid to achieve the same level of inhibition. Because EtSO4- is a more kosmotropic anion compared to Cl−, the laccase has a higher biocompatibility with the ionic liquid (Domínguez et al., 2011; Sun et al., 2017b).
While there are many potential inhibitors that could be derived from the biomass, various studies have shown ionic liquids acting as potential inhibitors for certain enzymes (Stock et al., 2004; Nascimento et al., 2019; Hebal et al., 2020). The conformation of an ionic liquid can potentially result in inhibition of activity by means of unfavorable structural interactions, also generally following the Hofmeister series. This is because interactions of the enzymes with the solvent on a molecular level may negatively affect substrate binding, reaction rates, and the structure of the active site of the enzyme. Competitive inhibition commonly affects the activity of pretreated lignocellulosic enzymes and occurs when inhibitors occupy the active site of the enzymes, effectively preventing the formation of an enzyme-substrate complex. An enzyme’s high affinity toward a substrate could potentially cause lower competitive inhibition, which is demonstrated in a study with Thermopolyspora flexuosa GH10 xylanase. The enzyme was inhibited less by [EMIM][OAc] compared to [EMIM][DMP] and [DBNH][OAc], probably due to the acetate anion’s inability to strongly bind to the active site (Anbarasan et al., 2017). Cations of the ionic liquid [EMIM][OAc] performed as a competitive inhibitor for Trichoderma longibrachiatum xylanase at concentrations of IL greater than 10% (v/v) (Jaeger and Pfaendtner, 2013). Thermoascus aurantiacus GH10 xylanase was also competitively inhibited by [EMIM][OAc], because of the competition between the substrate and the [EMIM] cation for the enzyme’s active site (Chawachart et al., 2014). While competitive inhibition is prevalent amongst lignocellulosic enzymes by ILs, other interactions with the enzymes can result in diverse types and varying degrees of inhibition.
Another type of inhibition that can occur is noncompetitive inhibition, which does not affect the enzyme-substrate complex, but rather decreases the efficacy of the enzyme and affects the overall reaction rate. For example, Carneiro et al. investigated the effect of [EMIM][EtSO4] and [EMIM][MDEGSO4] on peroxidase activity, and while both ILs functioned as noncompetitive inhibitors [EMIM][ETSO4] had a larger effect. It is suggested that the electrostatic interaction between the charged enzymes and the ILs potentially caused the conformational change, which then resulted in the inhibitory behavior (Carneiro et al., 2009). Hong et al.‘s study on the catalysis of horseradish peroxidase demonstrates [BMIM][BF4] acting as a weak non-competitive inhibitor on the enzyme (Hong et al., 2008). It is important to note that particular ILs do not always result in the same type of inhibition, as the experimental conditions play a role in determining the structural interactions between enzymes and ILs.
4.2 Effect of temperature on enzyme activity
Changes in temperature can have a direct impact on kinetic energy, which can alter the activation energy and affect the likelihood of molecular collisions occurring—both factors that can be engineered to improve the likelihood of enzyme binding (Peterson et al., 2007). However, enzymes are also susceptible to structural changes induced by variations in temperature (Peterson et al., 2007). These effects occur regardless of whether ILs are introduced (Brogan et al., 2018). Heating enzymes to a sufficiently high temperature can permanently unfold enzymes and conversely, at too low of temperatures, the likelihood of enzyme-substrate interactions with sufficient activation energy for catalysis can be low enough so that virtually no activity occurs. This range of temperatures wherein the enzyme is active is unique to each enzyme. Improvements in enzyme activity and stability from changes in temperature compete with deconstructive effects of ionic liquids on the biomass substrate. Therefore, the reaction temperature needs to be adequately optimized to balance these effects and produce the desired enzymatic activity and product yields.
One important method concerning biofuel production is the temperature at which enzymes of interest have the highest activity. Most procedures to measure the optimal temperature and pH of an enzyme occur before adding ILs-effectively assuming that these parameters are decoupled from IL concentration. Kodou et al. found that within the range of 0–500 mM of added [BMIM][OAc], the optimal temperature of β-glucosidase from Themotoga maritima remained constant, which substantiates this assumption for small concentrations of ILs (Kudou et al., 2014). However, results from Campen et al., which includes data at much higher IL concentrations, shows a small negative correlation of IL concentration on optimal activity temperature of A. niger A5IL97 between 0% and 30% (v/v) [EMIM][OAc] (Amaike Campen et al., 2017). Finding an optimal temperature becomes less straightforward in multi-enzyme systems where each enzyme can have different temperature-reaction rate dependencies.
Sufficient evidence does exist to suggest that the presence of even small amounts of ILs can substantially modify the unfolding temperature of different cellulases (Turner et al., 2003). For the majority of studied IL-enzyme pairs, the addition of ILs decreases the unfolding temperature of the enzyme. Table 6 shows several examples of cellulases across multiple studies that directly measured the effects of increasing temperature of denaturing enzymes in IL solutions. A clear negative correlation (.25°C–1°C/% concentration (v/v) IL) between the denaturing temperature and IL concentration is observed for the listed enzymes.
Notable counterexamples do exist. In one study, Bose et al. found that GC 220 cellulase from Tricoderma reesei was stabilized in solutions containing tris-(2-hydroxyethyl)methylammonium methylsulfate (HEMA) at temperatures close to 100°C, whereas it denatured in solutions without HEMA at temperatures approximately 50°C (Bose et al., 2010). Brogan et al. found that β-Glucosidase with surface modifications could be stabilized in 1-butyl-1-methylpyrrolidinium ([BMPL]) based ionic liquids ([BMPL][OAc] [BMPL][OTf] [BMPL][NTf2]) to temperatures up exceeding 130°C- a temperature nearly 80°C warmer than in aqueous media alone (Brogan et al., 2018). In doing so, the enzymatic capability of glucosidase, which is indispensable in biomass processing, increased by over 30-fold.
While seemingly promising, HEMA has shown relatively low potential for dissolving cellulose and its high viscosity reduces diffusion of enzymes to substrate making it a poor candidate for practical biomass systems by itself (Bose et al., 2012). Combined with another IL with a better ability to solubilize biomass components, a multi-IL cocktail might be able to best utilize the stabilization properties of HEMA (or another IL with analogous stabilization properties) while being able to effectively dissolve and degrade cellulose and hemicellulose. Indeed, Bose et al. found that the combination of HEMA and [BMIM][Cl] enhanced the process of glucose production from cellulose (Bose et al., 2012). The exact nature of the effect of ILs on temperature related parameters is variable on the specific IL-enzyme system. For instance, HEMA proved to be detrimental for other enzymes, such as penicillin G amidase (Cantone et al., 2007), and Cal B (Madeira Lau et al., 2004), but for future experimentation, beneficial properties of multicomponent systems might be able to be exploited to create more cost effective biorefinery production processes.
Temperature also has an effect on swelling and dissolution rates of lignocellulose in ILs (Sun et al., 2009). In part this is attributed to the destabilizing effects of higher temperatures on hydrogen bonds in the 3-D structure of cellulose (Zavrel et al., 2009). Swelling can fragment cellulose fibers as well as free cellulose fibers from the lignocellulosic matrix via applied inter and intra structural stresses. As solvating molecules have increased accessibility within the fiber structure after fragmentation they are able to better approach cellulose chains and dissolve them into solution (Cuissinat et al., 2008). Dissolved cellulose is in turn easier for enzymes to access, allowing for increased enzymatic activity. Wang et al. found that in wood chips treated with [AMIM][Cl], swelling did not begin until 70°C and cellulose did not begin being substantially dissolved until 100°C (Wang et al., 2011a). These temperatures are above temperatures that some enzymes are active, potentially limiting the practical application of these temperature induced effects. Hemicellulose is found to dissolve at lower temperatures in ionic liquids than cellulose. Labbé et al. reported that at temperatures 60°C–80°C [EMIM][OAc] is capable of cleaving the acetyl groups covalently attached to hemicellulose components in poplar wood (Labbé et al., 2012). This result suggests that at these more moderate temperatures ILs could more effectively disrupt the carbohydrate–lignin linkages and in turn, release hemicellulose from the lignocellulosic matrix and make it more accessible to xylanases. Another interesting effect of increasing temperature during the IL pretreatment process is that lignin polymers are susceptible to self-condensation reactions. Self-condensation results in increased molecular weight of lignin which can precipitate lignin. By disabling precipitated lignin from reentering lignocellulosic structures, it decreases the heterogeneity of the structure, and limits the recalcitrant effects that lignin usually applies (El Hage et al., 2010). However, these effects are observed at temperatures usually >150°C- well beyond the unfolding temperature of known lignocellulose degrading enzymes.
Some physicochemical properties of ILs such as viscosity are temperature dependent and have the ability to affect the reaction kinetics by causing changes in mass transfer and diffusivity, which plays a role in both the activity and stability of enzymes (Naushad et al., 2012). By extension, one might expect that viscosity of the IL solvent may play a deterministic role in process efficiency and cost. Indeed, some authors, such as Bose et al. attributed lower observed activities of cellulase in HEMA to the solvent’s high viscosity (Bose et al., 2010). Other authors, such as Zhao et al., investigated over 25 ILs and the relation between their viscosity and enzymatic reaction and suggested that IL viscosity played only a minor factor in enzymatic reaction rates (Zhao et al., 2009). For instance, some highly viscous ILs such as quaternary ammonium chloride, which has a viscosity of 512mPas at 25°C (roughly 500 times more viscous than water), did not have significantly lower reaction rates than other tested ILs. It should also be noted that ILs often appear at low concentrations mixed with water or organic solvents. These results, although by no means exhaustive, suggest that temperature dependent effects on IL viscosity do not play a completely deterministic role on enzyme function.
4.3 Effect of pH on enzyme activity
The acidity of the medium containing an enzyme not only affects the activity and shape of the enzyme, but also the shape and charge of the substrate. Specifically, pH can affect the ionization state of both acidic and alkaline amino acids that are present in the enzyme. This includes carboxyl functional groups on the side chains of acidic amino acids and amine-containing functional groups of basic amino acids. Changes in the chemical state of these amino acids can substantially alter the 3-dimensional geometry of an enzyme, thus changing the shape of the active site to be more or less effective at binding with a substrate of interest, or altogether inactivating the enzyme. Additionally, for the enzymatic reaction to occur, these functional groups often must be in a particular protonation state (Koshland, 1953). Typically, there is a range of pH at which enzymatic activity with a particular substrate is optimal. However, broad evidence suggests that this optimal pH range is a function of other factors such as temperature, salinity, IL concentration, and is highly dependent on enzymes and substrates in the system. It should be noted that ILs can be mildly acidic or basic and are able to alter the acidity of their local environment. The acidity of non-aqueous systems can be measured in many different ways, but perhaps the most well-established of these is the Hammett method which uses a range of closely related UV-vis probes to generate the Hammett acidity (Gräsvik et al., 2014). In practice, most ILs are mixed with water whereby more conventional methods of measuring their effects on pH can be utilized.
Differences in pH caused by the addition of ILs may have several different effects on enzymes. Most cellulases and some xylanases are glycoside hydrolases (GH) which function using acid-base mechanisms. Most GHs use a two-step double displacement mechanism whereby acid/base residue on a carbohydrate and a nucleophilic residue on the enzyme are produced (Knott et al., 2014). The first step requires proton transfer from the acid base residue. This mechanism is illustrated in Figure 9. Changes in pH caused by addition of ILs should in theory have some effect on this step by affecting the availability of protons, although their availability competes with the structural changes they induce.
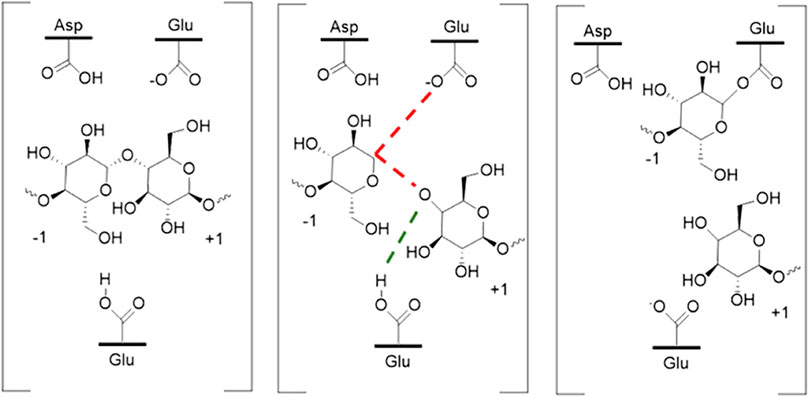
FIGURE 9. Schematic of one of the overall glycosyl hydrolysis reaction mechanisms highlighting proton exchange. Water is used to recycle the enzyme in this example.
The pH of a pretreatment process can also affect the way in which a substrate decomposes. In more acidic pretreatment environments, cellulose polymers have a tendency to stay intact, while hemicellulose hydrolyzes into monomeric sugars can form biotoxic compounds such as furfurals, HMF, and levulinic acid that inhibit downstream fermentation processes (Carvalheiro et al., 2008; Binder and Raines, 2010). At more neutral pHs, there tends to only be partial hydrolysis of the hemicelluloses. Often this is a result of autohydrolysis from organic acids within the lignocellulosic material. Since these conditions are not acidic enough, hemicelluloses tend to stay in polymeric or oligomeric form. In this regime, solubility of hemicelluloses is proportional to the concentration of organic acids available in biomass-meaning feedstocks such as softwoods typically have low solubilisation of hemicellulose. In more alkaline conditions lignin polymers tend to fractionalize and dissolve in solution, while hemicelluloses tend to remain in solid states. It may be of potential interest to factor inhibitory effects of biotoxic compound production during pretreatment on fermentation yields and cost.
Recent work by Pham et al. has reported that pH affects the ratio of products from the decomposition of lignin by lignin peroxidases isozyme H8 (Pham et al., 2021). This author suggests that pH is a key factor for efficient and selective depolymerization of lignin dimers (specifically guaiacyl glycerol beta-guaiacyl ether) and that engineering processes by altering pH could lead to improvements in bioprocessing in the future. How valuable this effect is for industrial processes is still to be determined.
4.4 Other factors (substrate, analysis method, quantification approach)
Varying origin or expression methods of the enzymes are also factors in potential IL-tolerance. The expression system of cell cultures has had an impact on enzyme quality, functionality, production speed, as well as yield. Natively expressed enzymes, enzymes produced in the organism of its origin, are often limited by demanding growth conditions to replicate the organisms’ native environment. For instance, fungal organisms are commonly cultured for cellulase production, but are limited by their need for special culturing and induction conditions. Additionally, enzyme yields are often limited and non-tailorable to different substrates due to substrate specificity of their metabolism. Heterologously produced enzymes currently suffer from their own drawbacks including low yields, poor secretion, and high costs (Lambertz et al., 2014). Specific modifications have been made to overcome these limitations in order to lower production costs and optimize expression systems, which differ among research labs and protocols.
At present a standard procedure across literature to quantify lignocellulosic enzyme activity in ionic liquids has not been fully realized. This comes despite the Commission on Biotechnology of Pure and Applied chemistry proposed a number of standard procedures for measuring cellulase activity in 1984 and the National Renewable Energy Laboratory (NREL) developing standardized procedures for different measurements in biomass processing in the early 2000s (Dowe, 2001; Dashtban et al., 2010). Variability in factors such as enzyme type, conditions in which they are active, substrate of interest, desired product, access to equipment, improvement of sensor technology and computational abilities, and even the deconstruction process itself, have all engendered the need to conduct alternative methods of analyzing deconstruction metrics (Fapyane and Ferapontova, 2017; Kwon et al., 2018).
Some studies opt to use “real” substrates, as opposed to model compounds for processing. In this case, real substrates are naturally occurring samples of organic compounds that are taken from biomass. Model compounds by contrast are typically synthetically produced chemicals or polymers of high purity. Oftentimes carboxymethyl cellulose (CMC), p-nitrophenol based compounds, or varieties of highly crystalline cellulose such as Avicel® are used to measure cellulase activity (Dashtban et al., 2010). Model compounds provide a more chemically simple system that can be specific to a single type of enzyme and give better insight on individual enzyme reaction pathway kinetics (Dashtban et al., 2010). Model compounds also lack impurities such as lignin that would typically be present at different concentrations in different biomass sources and create variations in enzyme activity across substrates (Ladeira Ázar et al., 2020). However, their use does not come without valid concerns. For one, model compounds do not chemically represent real substrates, and their reaction kinetics are not always directly comparable (Dashtban et al., 2011; Monschein et al., 2013). Additionally, the results from some model compounds have been reported to be notoriously non-reproducible for a variety of reasons specific to each compound (Percival Zhang et al., 2006). Despite their drawbacks, they remain popular options as measuring individual reaction pathways in enzyme assays from more complex substrates has proven difficult.
Due to the complexity of cellulose–cellulase systems and differences between kinetic characteristics of hydrolysis reaction and time, cellulase activity assays are often expressed by either their initial hydrolysis rate or by their end point hydrolysis yield. Initial hydrolysis rate measurements are preferred when measuring individual cellulase activity over a short time, whereas hydrolysis yields are used to measure total enzyme activity over a longer duration (Wu et al., 2006). Initial hydrolysis reactions are relatively free from complications such as back-reaction and enzyme degradation and product inhibition. Specific to cellulose-cellulase systems it should be noted that the rate of enzymatic hydrolysis of amorphous regions of cellulose are significantly higher than crystalline regions (Hall et al., 2010). Initial hydrolysis reaction rates are measured when the bulk of amorphous cellulose is still present and before their availability significantly alters enzyme velocity. End point measurements on the other hand account for enzyme deactivation caused by ILs, and feedback inhibition that occurs temporally. Reactions will significantly slow down by the end of the experiment as amorphous regions become scarcer, and cellulase activity becomes primarily a function of crystalline cellulose hydrolysis. It is sometimes indeterminate to what extent IL based deactivation occurs relative to feedback inhibition, so half-life studies in ILs are also conducted and first order deactivation models are applied (Schindl et al., 2019). As such, enzyme studies utilizing different assay methods are not always easily comparable and care must be taken when building datasets from multiple papers.
5 Mechanisms for improving enzyme activity
There exist various mechanisms for improving enzyme activity and stability in pretreatment and saccharification processes. Of these mechanisms, immobilization of enzymes on carriers and other stabilizing materials are often utilized to amplify the activity of the lignocellulosic enzymes. Immobilization allows for enhancing the biocatalytic properties of enzymes, making them more robust and chemically stable (Grewal et al., 2017). Other methods of modifying enzymes for enhanced performance include directed evolution, protein engineering, and enzyme-mediator complexes.
Numerous studies note that activity is drastically different, and oftentimes better for modified cellulases. Trichoderma reesei cellulases immobilized on magnetic and silica nanoparticles retain up to 95% activity in 10%–20% [EMIM][OAc]. Even in higher concentrations of the ionic liquid, the enzymes consistently retain above 50% activity (Grewal et al., 2017). Other T. reesei cellulases immobilized onto a modified ReliZyme HA403 support and Sepabeads EC-EP support possessed high reusability after 5 uses, and retained 57% and 32% activity, respectively (Bilgin et al., 2016). Crosslinking enzymes are an alternative method of immobilization compared to covalently bound immobilized cellulases. Crosslinked cellulase aggregates performed well in ∼43% [BMIM][OAc] and retained roughly 40% higher activity than in water, and also possessed high reusability, at 12 cycles with cellulose hydrolysis rates maintained above 50% relative to control (Jamwal et al., 2016). Xu et al. investigate cellulase activity in 25% [EMIM][DMP] and report around 50% relative activity of free cellulase, which increased to nearly 100% activity when immobilized on PEGylated graphene oxide nanosheets (Xu et al., 2016b). In a different study, it was found that while free cellulases lost nearly 95% activity in 40% [EMIM][DMP], modified cellulases by means of encapsulation in alginate beads retained up to 76% activity (Xu et al., 2015a).
Chemically modified laccases by various means often display higher levels of stability in increased retention of activity compared to free enzymes. Aspergillus laccase immobilized on modified silica carriers demonstrated greater stability in 50% [EMIM][EtSO4] after 2 days of incubation, maintaining 60% of its original activity compared to 50% maintained by the free enzyme (Tavares et al., 2013). Myceliophthora thermophilia laccase immobilized on glyoxyl-agarose beads by means of covalent binding allowed laccases to withstand stability of over 50% in 75% [EMIM][EtSO4] while free laccases were completely inactivated (Fernández-Fernández et al., 2014). No single means of modification is expected to produce exceptional results, but methods of immobilization and evolution generally support the enzyme in tolerating ionic liquid solvent media.
Directed evolution is a technique successfully used to improve enzyme activity in ionic liquids, which directly evolves biomolecules in desired conditions. More specifically, mimicking natural evolution in the lab with a gene library for diversification, followed by screening for certain mutated variants that function under the conditions desired. This process continues over a number of cycles until the biomolecule variant tests successfully to desired traits and conditions at a faster rate than what would naturally occur (Packer and Liu, 2015). Directed evolution has successfully been used for developing ionic liquid tolerant cellulases (Wolski et al., 2016), laccases (Liu et al., 2013), and peroxidases (Cherry et al., 1999; Ryu et al., 2008; Gonzalez-Perez and Alcalde, 2017). For instance, after 2 generations of directed evolution, Trametes versicolor laccases expressed in Saccharomyces cerevisiae resulted in a lcc2 variant with improved tolerance to [EMIM][EtSO4], with a 3.5 to 4.5-fold increase in activity compared to the lcc2 wild type in 15% IL (Liu et al., 2013). Protein engineered laccase variants demonstrated 3.4-fold–8.4-fold activity increase in 5%–35% [EMIM][EtSO4] compared to the wild type lcc2 enzyme (Wallraf et al., 2018). A cellulase from Talaromyces emersonii was also directly evolved over 2 generations to improve ionic liquid tolerance, yielding an increase in activity and stability within aqueous-IL solutions of 43% (w/w) [MMIM][DMP] and 20% (w/w) [EMIM][OAc] (Wolski et al., 2016). Despite the numerous instances in which directed evolution has been successful in making ionic liquid-tolerant enzymes, there are limitations to this method. In using directed evolution, evolving against a model compound substrate such as model lignin or cellulose would not always necessarily be an adequate representation of lignocellulosic biomass. The actual composition and structure of lignocellulosic biomass is unpredictable and has a lot of variability, which cannot be predicted or considered with the model biomass substrates used to screen for enzyme variants.
Enzyme-mediator complexes are also another method with potential in improving enzyme activity. Mediators are artificial electron acceptors, also often referred to as “redox mediators,” which carry the radical from the enzyme to the substrate in redox reactions. This helps the oxidative reaction take place without the enzyme needing to be directly touching the substrate. Working in conjunction with oxidative enzymes, mediator systems are especially of interest for laccases and peroxidases and have been extensively studied. These mediators can be added to a reaction but can also occur in nature. These naturally occurring mediators may be metabolites of certain organisms. Some lignin-consuming organisms, for instance, have some phenolic metabolites that act as naturally occurring mediators such as syringaldehyde, acetosyringone, vanillin, acetovanillone, methyl vanillate and p-coumaric acid (Cañas and Camarero, 2010). Several articles within literature found 2,2′-azino-bis(3-ethylbenzothiazoline-6-sulfonic acid (ABTS) and 1-hydroxybenzotriazole (HBT) to be popular and effective mediators for lignin-degrading enzymes such as laccase and peroxidases. Although not directly tested with ionic liquids in particular, unsaturated fatty acids and HBT were shown to be effective mediators for manganese peroxidase oxidative conservation of several environmental pollutants in organic solvents, increasing conversion rates 3-fold (Michizoe et al., 2004). However, their effectiveness in ILs may possibly be difficult to determine, as the ions of the IL have the potential to react with the radicals carried by the mediators due to their charge, which could also contribute to why enzyme-mediator complexes have mostly been investigated within organic solvents rather than ILs.
6 Conclusion
Ionic liquids (ILs) are popular solvents used for the pretreatment and dissolution of lignocellulosic biomass. As an eco-friendly solution, IL pretreatment processes are commonly utilized and have demonstrated high sugar yields and efficient recovery of desired material. Because of the notably harsh conditions that enzymes undergo during the IL pretreatment process, enzymatic activity and stability is often inhibited or notably reduced, and for this reason the pretreatment and enzyme hydrolysis steps are kept separate. The need to engineer IL-tolerant enzymes is increasingly prevalent for more efficient biofuel hydrolysis. We have reviewed a number of the factors that influence enzyme stability and activity in ILs and outline trends that allow for better understanding of which areas to target to improve these processes. While there is no blueprint or platform approach to apply uniform conditions for biofuel production processes due to the vast variety of ILs and solvents available, identifying such trends will play a hand in taking a step forward towards developing more productive techniques.
Experimental studies investigating potential enzyme cocktails to produce synergistic effects is a promising next step. Classifying enzymes by the optimum conditions under which they work and running computational analysis or designing a machine learning problem may be a more efficient method of optimizing enzyme activity and biomass dissolution. While this review provides a comprehensive understanding of the fundamentals of pretreatment and enzymatic activity, more work needs to be done to design robust enzymes and develop optimal processes.
Author contributions
BB, AU, MC contributed to the Conceptualization, Methodology, Validation, Formal analysis, Investigation Writing—Original Draft, Writing—Review and Editing and Visualization. PW and EA contributed to the Conceptualization, Methodology, Validation, Formal analysis, Investigation Writing—Original Draft, Writing—Review and Editing and Visualization, Resources, Data Curation, Supervision, Project administration. BS and KS contributed to Writing—Review and Editing, Supervision, Project administration, Funding acquisition. All authors contributed to the article and approved the submitted version.
Funding
This work was part of the DOE Joint BioEnergy Institute (http://www.jbei.org) supported by the US Department of Energy, Office of Science, Office of Biological and Environmental Research, through contract DE-AC02-05CH11231 between Lawrence Berkeley National Laboratory and the US Department of Energy. The United States Government retains and the publisher, by accepting the article for publication, acknowledges that the United States Government retains a non-exclusive, paid-up, irrevocable, world-wide license to publish or reproduce the published form of this manuscript, or allow others to do so, for United States Government purposes.
Conflict of interest
The authors declare that the research was conducted in the absence of any commercial or financial relationships that could be construed as a potential conflict of interest.
Publisher’s note
All claims expressed in this article are solely those of the authors and do not necessarily represent those of their affiliated organizations, or those of the publisher, the editors and the reviewers. Any product that may be evaluated in this article, or claim that may be made by its manufacturer, is not guaranteed or endorsed by the publisher.
References
Abdulsattar, M. O., Abdulsattar, J. O., Greenway, G. M., Welham, K. M., and Zein, S. H. (2020). Optimization of pH as a strategy to improve enzymatic saccharification of wheat straw for enhancing bioethanol production. J. Anal. Sci. Technol. 11, 17. doi:10.1186/s40543-020-00217-7
Achinivu, E. C., Cabrera, M., Umar, A., Yang, M., Baral, N. R., Scown, C. D., et al. (2022). In situ synthesis of protic ionic liquids for biomass pretreatment. ACS Sustain. Chem. Eng. 10, 12090–12098. doi:10.1021/acssuschemeng.2c01211
Adewuyi, A. (2022). Underutilized lignocellulosic waste as sources of feedstock for biofuel production in developing countries. Front. Energy Res. 10. doi:10.3389/fenrg.2022.741570
Agbor, V. B., Cicek, N., Sparling, R., Berlin, A., and Levin, D. B. (2011). Biomass pretreatment: fundamentals toward application. Biotechnol. Adv. 29, 675–685. doi:10.1016/j.biotechadv.2011.05.005
Amaike Campen, S., Lynn, J., Sibert, S. J., Srikrishnan, S., Phatale, P., Feldman, T., et al. (2017). Expression of naturally ionic liquid-tolerant thermophilic cellulases in Aspergillus Niger. PLoS ONE 12, e0189604. doi:10.1371/journal.pone.0189604
Anbarasan, S., Wahlström, R., Hummel, M., Ojamo, H., Sixta, H., and Turunen, O. (2017). High stability and low competitive inhibition of thermophilic Thermopolyspora flexuosa GH10 xylanase in biomass-dissolving ionic liquids. Appl. Microbiol. Biotechnol. 101, 1487–1498. doi:10.1007/s00253-016-7922-9
Balakshin, M., Capanema, E., Gracz, H., Chang, H., and Jameel, H. (2011). Quantification of lignin-carbohydrate linkages with high-resolution NMR spectroscopy. Planta 233, 1097–1110. doi:10.1007/s00425-011-1359-2
Baral, N. R., and Shah, A. (2016). Techno-economic analysis of cellulose dissolving ionic liquid pretreatment of lignocellulosic biomass for fermentable sugars production. Biofuels, Bioprod. Bioref. 10, 70–88. doi:10.1002/bbb.1622
Barcelos, C. A., Oka, A. M., Yan, J., Das, L., Achinivu, E. C., Magurudeniya, H., et al. (2021). High-efficiency conversion of ionic liquid-pretreated woody biomass to ethanol at the pilot scale. ACS Sustain. Chem. Eng. 9, 4042–4053. doi:10.1021/acssuschemeng.0c07920
Ben Hmad, I., Boudabbous, M., Belghith, H., and Gargouri, A. (2017). A novel ionic liquid-stable halophilic endoglucanase from Stachybotrys microspora. Process Biochem. 54, 59–66. doi:10.1016/j.procbio.2017.01.007
Bhardwaj, N., Agrawal, K., Kumar, B., and Verma, P. (2021). “Role of enzymes in deconstruction of waste biomass for sustainable generation of value-added products,” in Bioprospecting of enzymes in industry, healthcare and sustainable environment. Editors H. Thatoi, S. Mohapatra, and S. K. Das (Singapore: Springer Singapore), 219–250. doi:10.1007/978-981-33-4195-1_11
Bhutto, A. W., Qureshi, K., Abro, R., Harijan, K., Zhao, Z., Bazmi, A. A., et al. (2016). Progress in the production of biomass-to-liquid biofuels to decarbonize the transport sector—prospects and challenges. RSC Adv. 6, 32140–32170. doi:10.1039/C5RA26459F
Bilgin, R., Yalcin, M. S., and Yildirim, D. (2016). Optimization of covalent immobilization of Trichoderma reesei cellulase onto modified ReliZyme HA403 and Sepabeads EC-EP supports for cellulose hydrolysis, in buffer and ionic liquids/buffer media. Artif. Cells Nanomed. Biotechnol. 44, 1276–1284. doi:10.3109/21691401.2015.1024842
Binder, J. B., and Raines, R. T. (2010). Fermentable sugars by chemical hydrolysis of biomass. Proc. Natl. Acad. Sci. U. S. A. 107, 4516–4521. doi:10.1073/pnas.0912073107
Birikh, K., Michine, A., Heikkilä, M., and Ihalainen, P. (2022). “Enzymes—key elements of the future biorefineries,” in Biorefineries—Selected Processes. Editors K. Biernat (IntechOpen). doi:10.5772/intechopen.99415
Bose, S., Armstrong, D. W., and Petrich, J. W. (2010). Enzyme-catalyzed hydrolysis of cellulose in ionic liquids: a green approach toward the production of biofuels. J. Phys. Chem. B 114, 8221–8227. doi:10.1021/jp9120518
Bose, S., Barnes, C. A., and Petrich, J. W. (2012). Enhanced stability and activity of cellulase in an ionic liquid and the effect of pretreatment on cellulose hydrolysis. Biotechnol. Bioeng. 109, 434–443. doi:10.1002/bit.23352
Brennan, T. C. R., Datta, S., Blanch, H. W., Simmons, B. A., and Holmes, B. M. (2010). Recovery of sugars from ionic liquid biomass liquor by solvent extraction. Bioenerg. Res. 3, 123–133. doi:10.1007/s12155-010-9091-5
Brogan, A. P. S., Bui-Le, L., and Hallett, J. P. (2018). Non-aqueous homogenous biocatalytic conversion of polysaccharides in ionic liquids using chemically modified glucosidase. Nat. Chem. 10, 859–865. doi:10.1038/s41557-018-0088-6
Bussemaker, M. J., and Zhang, D. (2013). Effect of ultrasound on lignocellulosic biomass as a pretreatment for biorefinery and biofuel applications. Ind. Eng. Chem. Res. 52, 3563–3580. doi:10.1021/ie3022785
Cañas, A. I., and Camarero, S. (2010). Laccases and their natural mediators: biotechnological tools for sustainable eco-friendly processes. Biotechnol. Adv. 28, 694–705. doi:10.1016/j.biotechadv.2010.05.002
Cantone, S., Hanefeld, U., and Basso, A. (2007). Biocatalysis in non-conventional media—Ionic liquids, supercritical fluids and the gas phase. Green Chem. 9, 954. doi:10.1039/b618893a
Carneiro, A. P., Rodríguez, O., Mota, F. L., Tavares, A. P. M., and Macedo, E. A. (2009). Kinetic and stability study of the peroxidase inhibition in ionic liquids. Ind. Eng. Chem. Res. 48, 10810–10815. doi:10.1021/ie9007612
Carvalheiro, F., Duarte, L. C., and Girio, F. M. (2008). Hemicellulose biorefineries: a review on biomass pretreatments. J. Sci. Industrial Res.
Casas, A., Palomar, J., Alonso, M. V., Oliet, M., Omar, S., and Rodriguez, F. (2012). Comparison of lignin and cellulose solubilities in ionic liquids by COSMO-RS analysis and experimental validation. Industrial Crops Prod. 37, 155–163. doi:10.1016/j.indcrop.2011.11.032
Chan, J. C., Paice, M., and Zhang, X. (2020). Enzymatic oxidation of lignin: challenges and barriers toward practical applications. ChemCatChem 12, 401–425. doi:10.1002/cctc.201901480
Chandel, A. K., Garlapati, V. K., Singh, A. K., Antunes, F. A. F., and da Silva, S. S. (2018). The path forward for lignocellulose biorefineries: bottlenecks, solutions, and perspective on commercialization. Bioresour. Technol. 264, 370–381. doi:10.1016/j.biortech.2018.06.004
Chauhan, A. K., and Choudhury, B. (2020). Suitability of organic solvent and cholinium based ionic liquid activated novel lignolytic enzymes of H. aswanensis for enhanced Kalson lignin degradation. Int. J. Biol. Macromol. 165, 107–117. doi:10.1016/j.ijbiomac.2020.09.137
Chauhan, P. S., Goradia, B., and Jha, B. (2018). Optimization and up scaling of ionic liquid tolerant and thermo-alkali stable laccase from a marine Staphylococcus arlettae S1-20 using tea waste. J. Taiwan Inst. Chem. Eng. 86, 1–8. doi:10.1016/j.jtice.2018.02.032
Chawachart, N., Anbarasan, S., Turunen, S., Li, H., Khanongnuch, C., Hummel, M., et al. (2014). Thermal behaviour and tolerance to ionic liquid [emim]OAc in GH10 xylanase from Thermoascus aurantiacus SL16W. Extremophiles 18, 1023–1034. doi:10.1007/s00792-014-0679-0
Chen, H., and Wang, L. (2017). “Enzymatic hydrolysis of pretreated biomass,” in Technologies for biochemical conversion of biomass (Elsevier), 65–99. doi:10.1016/B978-0-12-802417-1.00004-1
Chen, Y., and Mu, T. (2019). Application of deep eutectic solvents in biomass pretreatment and conversion. Green Energy and Environ. 4, 95–115. doi:10.1016/j.gee.2019.01.012
Cherry, J. R., Lamsa, M. H., Schneider, P., Vind, J., Svendsen, A., Jones, A., et al. (1999). Directed evolution of a fungal peroxidase. Nat. Biotechnol. 17, 379–384. doi:10.1038/7939
Chiappe, C., and Pieraccini, D. (2005). Ionic liquids: solvent properties and organic reactivity. J. Phys. Org. Chem. 18, 275–297. doi:10.1002/poc.863
Climent Barba, F., Grasham, O., Puri, D. J., and Blacker, A. J. (2022). A simple techno-economic assessment for scaling-up the enzymatic hydrolysis of MSW pulp. Front. Energy Res. 10. doi:10.3389/fenrg.2022.788534
Costa, T. H. F., Kadic’, A., Chylenski, P., Várnai, A., Bengtsson, O., Lidén, G., et al. (2020). Demonstration-scale enzymatic saccharification of sulfite-pulped spruce with addition of hydrogen peroxide forLPMO activation. Biofuels, Bioprod. Bioref. 14, 734–745. doi:10.1002/bbb.2103
Cuissinat, C., Navard, P., and Heinze, T. (2008). Swelling and dissolution of cellulose. Part IV: free floating cotton and wood fibres in ionic liquids. Carbohydr. Polym. 72, 590–596. doi:10.1016/j.carbpol.2007.09.029
Dabirmanesh, B., Khajeh, K., Ghazi, F., Ranjbar, B., and Etezad, S.-M. (2015). A semi-rational approach to obtain an ionic liquid tolerant bacterial laccase through π-type interactions. Int. J. Biol. Macromol. 79, 822–829. doi:10.1016/j.ijbiomac.2015.06.002
Das, D., Dasgupta, A., and Das, P. K. (2007). Improved activity of horseradish peroxidase (HRP) in ‘specifically designed’ ionic liquid. Tetrahedron Lett. 48, 5635–5639. doi:10.1016/j.tetlet.2007.06.022
Das, L., Achinivu, E. C., Barcelos, C. A., Sundstrom, E., Amer, B., Baidoo, E. E. K., et al. (2021). Deconstruction of woody biomass via protic and aprotic ionic liquid pretreatment for ethanol production. ACS Sustain. Chem. Eng. 9, 4422–4432. doi:10.1021/acssuschemeng.0c07925
Dashtban, M., Buchkowski, R., and Qin, W. (2011). Effect of different carbon sources on cellulase production by Hypocrea jecorina (Trichoderma reesei) strains. Int. J. Biochem. Mol. Biol. 2, 274–286.
Dashtban, M., Maki, M., Leung, K. T., Mao, C., and Qin, W. (2010). Cellulase activities in biomass conversion: measurement methods and comparison. Crit. Rev. Biotechnol. 30, 302–309. doi:10.3109/07388551.2010.490938
Datta, S., Holmes, B., Park, J. I., Chen, Z., Dibble, D. C., Hadi, M., et al. (2010). Ionic liquid tolerant hyperthermophilic cellulases for biomass pretreatment and hydrolysis. Green Chem. 12, 338. doi:10.1039/b916564a
Davis, R. E., Grundl, N. J., Tao, L., Biddy, M. J., Tan, E. C., Beckham, G. T., et al. (2018). Process design and economics for the conversion of lignocellulosic biomass to hydrocarbon fuels and coproducts: 2018 biochemical design case update; biochemical deconstruction and conversion of biomass to fuels and products via integrated biorefinery pathways. Golden, CO (United States): National Renewable Energy Laboratory NREL. doi:10.2172/1483234
Del Mar Contreras-Gámez, M., Galán-Martín, Á., Seixas, N., da Costa Lopes, A. M., Silvestre, A., and Castro, E. (2023). Deep eutectic solvents for improved biomass pretreatment: current status and future prospective towards sustainable processes. Bioresour. Technol. 369, 128396. doi:10.1016/j.biortech.2022.128396
Dhawane, S. H., Ghosh, A., Dwivedi, P., Dey, S., Kamila, B., and Halder, G. (2022). “Critical evaluation of the role of enzymes in the integrated biorefinery,”in. Thermochemical and catalytic conversion technologies for future biorefineries: volume 2 clean energy production technologies. Editor P. Verma (Singapore: Springer Nature Singapore), 77–105. doi:10.1007/978-981-19-4316-4_4
Dimarogona, M., Topakas, E., and Christakopoulos, P. (2012). Cellulose degradation by oxidative enzymes. Comput. Struct. Biotechnol. J. 2, e201209015. doi:10.5936/csbj.201209015
Domínguez, A., Rodríguez, O., Tavares, A. P. M., Macedo, E. A., Longo, M. A., and Sanromán, M. A. (2011). Studies of laccase from Trametes versicolor in aqueous solutions of several methylimidazolium ionic liquids. Bioresour. Technol. 102, 7494–7499. doi:10.1016/j.biortech.2011.05.063
Dong, S.-J., Zhang, B.-X. Z., Wang, F.-L., Xin, L., Gao, Y.-F., Ding, W., et al. (2019). Efficient lignin degradation of corn stalk by Trametes with high laccase activity and enzymatic stability in salt and ionic liquid. BioResources 14, 5339–5354. doi:10.15376/biores.14.3.5339-5354
Du, H., and Qian, X. (2011). The effects of acetate anion on cellulose dissolution and reaction in imidazolium ionic liquids. Carbohydr. Res. 346, 1985–1990. doi:10.1016/j.carres.2011.05.022
El Hage, R., Chrusciel, L., Desharnais, L., and Brosse, N. (2010). Effect of autohydrolysis of Miscanthus x giganteus on lignin structure and organosolv delignification. Bioresour. Technol. 101, 9321–9329. doi:10.1016/j.biortech.2010.06.143
Elgharbawy, A. A., Alam, Md. Z., Kabbashi, N. A., Moniruzzaman, M., and Jamal, P. (2016). Evaluation of several ionic liquids for in situ hydrolysis of empty fruit bunches by locally-produced cellulase. 3 Biotech. 6, 128. doi:10.1007/s13205-016-0440-8
Falade, A. O., Nwodo, U. U., Iweriebor, B. C., Green, E., Mabinya, L. V., and Okoh, A. I. (2017). Lignin peroxidase functionalities and prospective applications. Microbiologyopen 6, e00394. doi:10.1002/mbo3.394
Fapyane, D., and Ferapontova, E. E. (2017). Electrochemical assay for a total cellulase activity with improved sensitivity. Anal. Chem. 89, 3959–3965. doi:10.1021/acs.analchem.6b04391
Feder-Kubis, J., and Bryjak, J. (2013). Laccase activity and stability in the presence of menthol-based ionic liquids. Acta Biochim. Pol. 60, 741–745. doi:10.18388/abp.2013_2051
Fendt, S., Padmanabhan, S., Blanch, H. W., and Prausnitz, J. M. (2011). Viscosities of acetate or chloride-based ionic liquids and some of their mixtures with water or other common solvents. J. Chem. Eng. Data 56, 31–34. doi:10.1021/je1007235
Fernández-Fernández, M., Moldes, D., Domínguez, A., Sanromán, M. Á., Tavares, A. P. M., Rodríguez, O., et al. (2014). Stability and kinetic behavior of immobilized laccase from Myceliophthora thermophila in the presence of the ionic liquid 1-ethyl-3-methylimidazolium ethylsulfate. Biotechnol. Prog. 30, 790–796. doi:10.1002/btpr.1910
Flieger, J., and Flieger, M. (2020). Ionic liquids toxicity-benefits and threats. Int. J. Mol. Sci. 21, 6267. doi:10.3390/ijms21176267
Gladden, J. M., Park, J. I., Bergmann, J., Reyes-Ortiz, V., D’haeseleer, P., Quirino, B. F., et al. (2014). Discovery and characterization of ionic liquid-tolerant thermophilic cellulases from a switchgrass-adapted microbial community. Biotechnol. Biofuels 7, 15. doi:10.1186/1754-6834-7-15
Gonzalez-Perez, D., and Alcalde, M. (2017). The making of versatile peroxidase by directed evolution. Biocatal. Biotransformation 36, 1–11. doi:10.1080/10242422.2017.1363190
Gräsvik, J., Hallett, J. P., To, T. Q., and Welton, T. (2014). A quick, simple, robust method to measure the acidity of ionic liquids. Chem. Commun. 50, 7258–7261. doi:10.1039/c4cc02816c
Grewal, J., Ahmad, R., and Khare, S. K. (2017). Development of cellulase-nanoconjugates with enhanced ionic liquid and thermal stability for in situ lignocellulose saccharification. Bioresour. Technol. 242, 236–243. doi:10.1016/j.biortech.2017.04.007
Gunny, A. A. N., Arbain, D., Edwin Gumba, R., Jong, B. C., and Jamal, P. (2014). Potential halophilic cellulases for in situ enzymatic saccharification of ionic liquids pretreated lignocelluloses. Bioresour. Technol. 155, 177–181. doi:10.1016/j.biortech.2013.12.101
Haldar, D., Sen, D., and Gayen, K. (2016). A review on the production of fermentable sugars from lignocellulosic biomass through conventional and enzymatic route—A comparison. Int. J. Green Energy 13, 1232–1253. doi:10.1080/15435075.2016.1181075
Hall, M., Bansal, P., Lee, J. H., Realff, M. J., and Bommarius, A. S. (2010). Cellulose crystallinity--a key predictor of the enzymatic hydrolysis rate. FEBS J. 277, 1571–1582. doi:10.1111/j.1742-4658.2010.07585.x
Hallac, B. B., and Ragauskas, A. J. (2011). Analyzing cellulose degree of polymerization and its relevancy to cellulosic ethanol. Biofuels, Bioprod. Bioref. 5, 215–225. doi:10.1002/bbb.269
Harwardt, N., Stripling, N., Roth, S., Liu, H., Schwaneberg, U., and Spiess, A. C. (2014). Effects of ionic liquids on the reaction kinetics of a laccase–mediator system. RSC Adv. 4, 17097–17104. doi:10.1039/C4RA00733F
He, Y.-C., Liu, F., Gong, L., Di, J.-H., Ding, Y., Ma, C.-L., et al. (2016). Enzymatic in situ saccharification of chestnut shell with high ionic liquid-tolerant cellulases from Galactomyces sp. CCZU11-1 in a biocompatible ionic liquid-cellulase media. Bioresour. Technol. 201, 133–139. doi:10.1016/j.biortech.2015.11.034
Hebal, H., Parviainen, A., Anbarasan, S., Li, H., Makkonen, L., Bankar, S., et al. (2020). Inhibition of hyperthermostable xylanases by superbase ionic liquids. Process Biochem. 95, 148–156. doi:10.1016/j.procbio.2020.03.022
Hedegård, E. D., and Ryde, U. (2018). Molecular mechanism of lytic polysaccharide monooxygenases. Chem. Sci. 9, 3866–3880. doi:10.1039/c8sc00426a
Hemsworth, G. R., Henrissat, B., Davies, G. J., and Walton, P. H. (2014). Discovery and characterization of a new family of lytic polysaccharide monooxygenases. Nat. Chem. Biol. 10, 122–126. doi:10.1038/nchembio.1417
Hong, E. S., Kwon, O. Y., and Ryu, K. (2008). Strong substrate-stabilizing effect of a water-miscible ionic liquid [BMIM] [BF(4)] in the catalysis of horseradish peroxidase. Biotechnol. Lett. 30, 529–533. doi:10.1007/s10529-007-9570-8
Hou, Q., Ju, M., Li, W., Liu, L., Chen, Y., and Yang, Q. (2017). Pretreatment of lignocellulosic biomass with ionic liquids and ionic liquid-based solvent systems. Molecules 22, 490. doi:10.3390/molecules22030490
Hu, D., Ju, X., Li, L., Hu, C., Yan, L., Wu, T., et al. (2016a). Improved in situ saccharification of cellulose pretreated by dimethyl sulfoxide/ionic liquid using cellulase from a newly isolated Paenibacillus sp. LLZ1. Bioresour. Technol. 201, 8–14. doi:10.1016/j.biortech.2015.11.039
Hu, D., Xiao, L., Li, L., Zhong, C., Ju, X., Yan, L., et al. (2016b). Effects of ionic liquid 1-ethyl-3-methylimidazolium diethylphosphate on cellulase produced by Paenibacillus sp. LLZ1. ACS Sustain. Chem. Eng. 4, 4922–4926. doi:10.1021/acssuschemeng.6b01229
Hu, J., and Saddler, J. N. (2018). Why does GH10 xylanase have better performance than GH11 xylanase for the deconstruction of pretreated biomass? Biomass Bioenergy 110, 13–16. doi:10.1016/j.biombioe.2018.01.007
Ilmberger, N., Pottkämper, J., and Streit, W. (2013). Cellulases in ionic liquids—the long term stability of Aspergillus sp. cellulase. Catalysts 3, 584–587. doi:10.3390/catal3020584
Isikgor, F. H., and Becer, C. R. (2015). Lignocellulosic biomass: A sustainable platform for the production of bio-based chemicals and polymers. Polym. Chem. 6, 4497–4559. doi:10.1039/C5PY00263J
Jaeger, V. W., and Pfaendtner, J. (2013). Structure, dynamics, and activity of xylanase solvated in binary mixtures of ionic liquid and water. ACS Chem. Biol. 8, 1179–1186. doi:10.1021/cb3006837
Jamwal, S., Chauhan, G. S., Ahn, J.-H., and Reddy, N. S. (2016). Cellulase stabilization by crosslinking with ethylene glycol dimethacrylate and evaluation of its activity including in a water–ionic liquid mixture. RSC Adv. 6, 25485–25491. doi:10.1039/C5RA19571C
Jeswani, H. K., Chilvers, A., and Azapagic, A. (2020). Environmental sustainability of biofuels: A review. Proc. Math. Phys. Eng. Sci. 476, 20200351. doi:10.1098/rspa.2020.0351
Johnson, L. B., Park, S., Gintner, L. P., and Snow, C. D. (2016). Characterization of supercharged cellulase activity and stability in ionic liquids. J. Mol. Catal. B Enzym. 132, 84–90. doi:10.1016/j.molcatb.2016.05.008
Jørgensen, H., and Pinelo, M. (2017). Enzyme recycling in lignocellulosic biorefineries. Biofuels, Bioprod. Bioref. 11, 150–167. doi:10.1002/bbb.1724
Kainthola, J., Podder, A., Fechner, M., and Goel, R. (2021). An overview of fungal pretreatment processes for anaerobic digestion: applications, bottlenecks and future needs. Bioresour. Technol. 321, 124397. doi:10.1016/j.biortech.2020.124397
Kamiya, N., Matsushita, Y., Hanaki, M., Nakashima, K., Narita, M., Goto, M., et al. (2008). Enzymatic in situ saccharification of cellulose in aqueous-ionic liquid media. Biotechnol. Lett. 30, 1037–1040. doi:10.1007/s10529-008-9638-0
Kim, J. S., Lee, Y. Y., and Kim, T. H. (2016). A review on alkaline pretreatment technology for bioconversion of lignocellulosic biomass. Bioresour. Technol. 199, 42–48. doi:10.1016/j.biortech.2015.08.085
Klein-Marcuschamer, D., Oleskowicz-Popiel, P., Simmons, B. A., and Blanch, H. W. (2012). The challenge of enzyme cost in the production of lignocellulosic biofuels. Biotechnol. Bioeng. 109, 1083–1087. doi:10.1002/bit.24370
Klein-Marcuschamer, D., Simmons, B. A., and Blanch, H. W. (2011). Techno-economic analysis of a lignocellulosic ethanol biorefinery with ionic liquid pre-treatment. Biofuels, Bioprod. Bioref. 5, 562–569. doi:10.1002/bbb.303
Knott, B. C., Haddad Momeni, M., Crowley, M. F., Mackenzie, L. F., Götz, A. W., Sandgren, M., et al. (2014). The mechanism of cellulose hydrolysis by a two-step, retaining cellobiohydrolase elucidated by structural and transition path sampling studies. J. Am. Chem. Soc. 136, 321–329. doi:10.1021/ja410291u
Konda, N. M., Shi, J., Singh, S., Blanch, H. W., Simmons, B. A., and Klein-Marcuschamer, D. (2014). Understanding cost drivers and economic potential of two variants of ionic liquid pretreatment for cellulosic biofuel production. Biotechnol. Biofuels 7, 86. doi:10.1186/1754-6834-7-86
Koshland, D. E. (1953). Stereochemistry and the mechanism of enzymatic reactions. Biol. Rev. 28, 416–436. doi:10.1111/j.1469-185X.1953.tb01386.x
Kudou, M., Kubota, Y., Nakashima, N., Okazaki, F., Nakashima, K., Ogino, C., et al. (2014). Improvement of enzymatic activity of β-glucosidase from Thermotoga maritima by 1-butyl-3-methylimidazolium acetate. J. Mol. Catal. B Enzym. 104, 17–22. doi:10.1016/j.molcatb.2014.02.013
Kwon, K. K., Yeom, S.-J., Lee, D.-H., Jeong, K. J., and Lee, S.-G. (2018). Development of a novel cellulase biosensor that detects crystalline cellulose hydrolysis using a transcriptional regulator. Biochem. Biophys. Res. Commun. 495, 1328–1334. doi:10.1016/j.bbrc.2017.11.157
Labbé, N., Kline, L. M., Moens, L., Kim, K., Kim, P. C., and Hayes, D. G. (2012). Activation of lignocellulosic biomass by ionic liquid for biorefinery fractionation. Bioresour. Technol. 104, 701–707. doi:10.1016/j.biortech.2011.10.062
Ladeira Ázar, R. I. S., Bordignon-Junior, S. E., Laufer, C., Specht, J., Ferrier, D., and Kim, D. (2020). Effect of lignin content on cellulolytic saccharification of liquid hot water pretreated sugarcane bagasse. Molecules 25, 623. doi:10.3390/molecules25030623
Lambertz, C., Garvey, M., Klinger, J., Heesel, D., Klose, H., Fischer, R., et al. (2014). Challenges and advances in the heterologous expression of cellulolytic enzymes: A review. Biotechnol. Biofuels 7, 135. doi:10.1186/s13068-014-0135-5
Lange, H., Decina, S., and Crestini, C. (2013). Oxidative upgrade of lignin – recent routes reviewed. Eur. Polym. J. 49, 1151–1173. doi:10.1016/j.eurpolymj.2013.03.002
Lee, S., Kang, M., Bae, J.-H., Sohn, J.-H., and Sung, B. H. (2019). Bacterial valorization of lignin: strains, enzymes, conversion pathways, biosensors, and perspectives. Front. Bioeng. Biotechnol. 7, 209. doi:10.3389/fbioe.2019.00209
Lei, Z., Chen, B., Koo, Y.-M., and MacFarlane, D. R. (2017). Introduction: ionic liquids. Chem. Rev. 117, 6633–6635. doi:10.1021/acs.chemrev.7b00246
Leynaud Kieffer Curran, L. M. C., Pham, L. T. M., Sale, K. L., and Simmons, B. A. (2022). Review of advances in the development of laccases for the valorization of lignin to enable the production of lignocellulosic biofuels and bioproducts. Biotechnol. Adv. 54, 107809. doi:10.1016/j.biotechadv.2021.107809
Li, C., Knierim, B., Manisseri, C., Arora, R., Scheller, H. V., Auer, M., et al. (2010). Comparison of dilute acid and ionic liquid pretreatment of switchgrass: biomass recalcitrance, delignification and enzymatic saccharification. Bioresour. Technol. 101, 4900–4906. doi:10.1016/j.biortech.2009.10.066
Li, H., Kankaanpää, A., Xiong, H., Hummel, M., Sixta, H., Ojamo, H., et al. (2013). Thermostabilization of extremophilic Dictyoglomus thermophilum GH11 xylanase by an N-terminal disulfide bridge and the effect of ionic liquid [emim]OAc on the enzymatic performance. Enzyme Microb. Technol. 53, 414–419. doi:10.1016/j.enzmictec.2013.09.004
Li, L., Xie, J., Yu, S., Su, Z., Liu, S., Liu, F., et al. (2012). Novel compatible system of [C2OHmim] [OAc]-cellulases for the in situ hydrolysis of lignocellulosic biomass. RSC Adv. 2, 11712. doi:10.1039/c2ra22128d
Li, L., Zhou, W., Wu, H., Yu, Y., Liu, F., and Zhu, D. (2014). Relationship between crystallinity index and enzymatic hydrolysis performance of celluloses separated from aquatic and terrestrial plant materials. Bioresources 9, 3993–4005. doi:10.15376/biores.9.3.3993-4005
Ling, S., Kaplan, D. L., and Buehler, M. J. (2018). Nanofibrils in nature and materials engineering. Nat. Rev. Mat. 3, 18016. doi:10.1038/natrevmats.2018.16
Ling, Z., Chen, S., Zhang, X., Takabe, K., and Xu, F. (2017). Unraveling variations of crystalline cellulose induced by ionic liquid and their effects on enzymatic hydrolysis. Sci. Rep. 7, 10230. doi:10.1038/s41598-017-09885-9
Liu, G., and Qu, Y. (2021). Integrated engineering of enzymes and microorganisms for improving the efficiency of industrial lignocellulose deconstruction. Eng. Microbiol. 1, 100005. doi:10.1016/j.engmic.2021.100005
Liu, H., Zhu, L., Bocola, M., Chen, N., Spiess, A. C., and Schwaneberg, U. (2013). Directed laccase evolution for improved ionic liquid resistance. Green Chem. 15, 1348. doi:10.1039/c3gc36899h
Liu, Y., Tang, Y., Gao, H., Zhang, W., Jiang, Y., Xin, F., et al. (2021). Challenges and future perspectives of promising biotechnologies for lignocellulosic biorefinery. Molecules 26, 5411. doi:10.3390/molecules26175411
Liu, Z., Li, L., Liu, C., and Xu, A. (2017). Saccharification of cellulose in the ionic liquids and glucose recovery. Renew. Energy 106, 99–102. doi:10.1016/j.renene.2017.01.023
Longe, L. F., Couvreur, J., Leriche Grandchamp, M., Garnier, G., Allais, F., and Saito, K. (2018). Importance of mediators for lignin degradation by fungal laccase. ACS Sustain. Chem. Eng. 6, 10097–10107. doi:10.1021/acssuschemeng.8b01426
Lozano, P., Bernal, B., Bernal, J. M., Pucheault, M., and Vaultier, M. (2011). Stabilizing immobilized cellulase by ionic liquids for saccharification of cellulose solutions in 1-butyl-3-methylimidazolium chloride. Green Chem. 13, 1406. doi:10.1039/c1gc15294g
Machado, M. F., and Saraiva, J. M. (2005). Thermal stability and activity regain of horseradish peroxidase in aqueous mixtures of imidazolium-based ionic liquids. Biotechnol. Lett. 27, 1233–1239. doi:10.1007/s10529-005-0023-y
Madeira Lau, R., Sorgedrager, M. J., Carrea, G., van Rantwijk, F., Secundo, F., and Sheldon, R. A. (2004). Dissolution of Candida Antarctica lipase B in ionic liquids: effects on structure and activity. Green Chem. 6, 483. doi:10.1039/b405693k
Mai, N. L., Ahn, K., and Koo, Y.-M. (2014). Methods for recovery of ionic liquids—a review. Process Biochem. 49, 872–881. doi:10.1016/j.procbio.2014.01.016
Manna, B., and Ghosh, A. (2020). Structure and dynamics of ionic liquid tolerant hyperthermophilic endoglucanase Cel12A from Rhodothermus marinus. RSC Adv. 10, 7933–7947. doi:10.1039/C9RA09612D
Martínez, A. T., Speranza, M., Ruiz-Dueñas, F. J., Ferreira, P., Camarero, S., Guillén, F., et al. (2005). Biodegradation of lignocellulosics: microbial, chemical, and enzymatic aspects of the fungal attack of lignin. Int. Microbiol. 8, 195–204.
Meine, N., Benedito, F., and Rinaldi, R. (2010). Thermal stability of ionic liquids assessed by potentiometric titration. Green Chem. 12, 1711. doi:10.1039/c0gc00091d
Meng, X., Pu, Y., Yoo, C. G., Li, M., Bali, G., Park, D.-Y., et al. (2017). An in-depth understanding of biomass recalcitrance using natural poplar variants as the feedstock. ChemSusChem 10, 139–150. doi:10.1002/cssc.201601303
Messali, M. (2014). An efficient and green sonochemical synthesis of some new eco-friendly functionalized ionic liquids. Arabian J. Chem. 7, 63–70. doi:10.1016/j.arabjc.2013.08.023
Michizoe, J., Uchimura, Y., Ichinose, H., Maruyama, T., Kamiya, N., Wariishi, H., et al. (2004). Activation of manganese peroxidase in an organic medium using a mediator. Biochem. Eng. J. 19, 43–46. doi:10.1016/j.bej.2003.09.008
Monschein, M., Reisinger, C., and Nidetzky, B. (2013). Enzymatic hydrolysis of microcrystalline cellulose and pretreated wheat straw: A detailed comparison using convenient kinetic analysis. Bioresour. Technol. 128, 679–687. doi:10.1016/j.biortech.2012.10.129
Morais, A. R. C., da Costa Lopes, A. M., and Bogel-Łukasik, R. (2015). Carbon dioxide in biomass processing: contributions to the green biorefinery concept. Chem. Rev. 115, 3–27. doi:10.1021/cr500330z
Nanda, S., Mohammad, J., Reddy, S. N., Kozinski, J. A., and Dalai, A. K. (2014). Pathways of lignocellulosic biomass conversion to renewable fuels. Biomass Conv. bioref. 4, 157–191. doi:10.1007/s13399-013-0097-z
Nascimento, P. A. M., Picheli, F. P., Lopes, A. M., Pereira, J. F. B., and Santos-Ebinuma, V. C. (2019). Effects of cholinium-based ionic liquids on Aspergillus Niger lipase: stabilizers or inhibitors. Biotechnol. Prog. 35, e2838. doi:10.1002/btpr.2838
Naushad, M., Alothman, Z. A., Khan, A. B., and Ali, M. (2012). Effect of ionic liquid on activity, stability, and structure of enzymes: A review. Int. J. Biol. Macromol. 51, 555–560. doi:10.1016/j.ijbiomac.2012.06.020
Nordwald, E. M., Brunecky, R., Himmel, M. E., Beckham, G. T., and Kaar, J. L. (2014). Charge engineering of cellulases improves ionic liquid tolerance and reduces lignin inhibition. Biotechnol. Bioeng. 111, 1541–1549. doi:10.1002/bit.25216
Norrrahim, M. N. F., Huzaifah, M. R. M., Farid, M. A. A., Shazleen, S. S., Misenan, M. S. M., Yasim-Anuar, T. A. T., et al. (2021). Greener pretreatment approaches for the valorisation of natural fibre biomass into bioproducts. Polym. (Basel) 13, 2971. doi:10.3390/polym13172971
OECD (2019). “Global mitigation potential of biofuels in the transport sector,” in Enhancing the Mitigation of Climate Change though Agriculture: Policies, Economic Consequences, and Trade-offs (OECD), 107–132. doi:10.1787/dce06785-en
Østby, H., Hansen, L. D., Horn, S. J., Eijsink, V. G. H., and Várnai, A. (2020). Enzymatic processing of lignocellulosic biomass: principles, recent advances and perspectives. J. Ind. Microbiol. Biotechnol. 47, 623–657. doi:10.1007/s10295-020-02301-8
Ovejero-Pérez, A., Ayuso, M., Rigual, V., Domínguez, J. C., García, J., Alonso, M. V., et al. (2021). Technoeconomic assessment of a biomass pretreatment + ionic liquid recovery process with aprotic and choline derived ionic liquids. ACS Sustain. Chem. Eng. 9, 8467–8476. doi:10.1021/acssuschemeng.1c01361
Packer, M. S., and Liu, D. R. (2015). Methods for the directed evolution of proteins. Nat. Rev. Genet. 16, 379–394. doi:10.1038/nrg3927
Park, J. H., Yoo, I. K., Kwon, O. Y., and Ryu, K. (2011). Partial uncompetitive inhibition of horseradish peroxidase by a water-miscible ionic liquid [BMIM] [MeSO4]. Biotechnol. Lett. 33, 1657–1662. doi:10.1007/s10529-011-0618-4
Pecha, M. B., and Garcia-Perez, M. (2020). “Pyrolysis of lignocellulosic biomass: oil, char, and gas,” in Bioenergy (Elsevier), 581–619. doi:10.1016/B978-0-12-815497-7.00029-4
Pedersen, J. N., Pérez, B., and Guo, Z. (2019). Stability of cellulase in ionic liquids: correlations between enzyme activity and COSMO-RS descriptors. Sci. Rep. 9, 17479. doi:10.1038/s41598-019-53523-5
Peng, X., Masai, E., Kitayama, H., Harada, K., Katayama, Y., and Fukuda, M. (2002). Characterization of the 5-carboxyvanillate decarboxylase gene and its role in lignin-related biphenyl catabolism in Sphingomonas paucimobilis SYK-6. Appl. Environ. Microbiol. 68, 4407–4415. doi:10.1128/AEM.68.9.4407-4415.2002
Percival Zhang, Y. H., Himmel, M. E., and Mielenz, J. R. (2006). Outlook for cellulase improvement: screening and selection strategies. Biotechnol. Adv. 24, 452–481. doi:10.1016/j.biotechadv.2006.03.003
Peterson, M. E., Daniel, R. M., Danson, M. J., and Eisenthal, R. (2007). The dependence of enzyme activity on temperature: determination and validation of parameters. Biochem. J. 402, 331–337. doi:10.1042/BJ20061143
Pham, L. T. M., Deng, K., Northen, T. R., Singer, S. W., Adams, P. D., Simmons, B. A., et al. (2021). Experimental and theoretical insights into the effects of pH on catalysis of bond-cleavage by the lignin peroxidase isozyme H8 from Phanerochaete chrysosporium. Biotechnol. Biofuels 14, 108. doi:10.1186/s13068-021-01953-7
Pottkämper, J., Barthen, P., Ilmberger, N., Schwaneberg, U., Schenk, A., Schulte, M., et al. (2009). Applying metagenomics for the identification of bacterial cellulases that are stable in ionic liquids. Green Chem. 11, 957. doi:10.1039/b820157a
Putrino, F. M., Tedesco, M., Bodini, R. B., and Oliveira, A. L. de (2020). Study of supercritical carbon dioxide pretreatment processes on green coconut fiber to enhance enzymatic hydrolysis of cellulose. Bioresour. Technol. 309, 123387. doi:10.1016/j.biortech.2020.123387
Qiao, J., Cui, H., Wang, M., Fu, X., Wang, X., Li, X., et al. (2022). Integrated biorefinery approaches for the industrialization of cellulosic ethanol fuel. Bioresour. Technol. 360, 127516. doi:10.1016/j.biortech.2022.127516
Raddadi, N., Cherif, A., Daffonchio, D., and Fava, F. (2013). Halo-alkalitolerant and thermostable cellulases with improved tolerance to ionic liquids and organic solvents from Paenibacillus tarimensis isolated from the Chott El Fejej, Sahara desert, Tunisia. Bioresour. Technol. 150, 121–128. doi:10.1016/j.biortech.2013.09.089
Ragauskas, A. J., Beckham, G. T., Biddy, M. J., Chandra, R., Chen, F., Davis, M. F., et al. (2014). Lignin valorization: improving lignin processing in the biorefinery. Science 344, 1246843. doi:10.1126/science.1246843
Ramos, L. P., Suota, M. J., Fockink, D. H., Pavaneli, G., da Silva, T. A., and Łukasik, R. M. (2020). “Enzymes and biomass pretreatment,” in Recent advances in bioconversion of lignocellulose to biofuels and value-added chemicals within the biorefinery concept (Elsevier), 61–100. doi:10.1016/B978-0-12-818223-9.00004-7
Remsing, R. C., Hernandez, G., Swatloski, R. P., Massefski, W. W., Rogers, R. D., and Moyna, G. (2008). Solvation of carbohydrates in n,n’-dialkylimidazolium ionic liquids: A multinuclear NMR spectroscopy study. J. Phys. Chem. B 112, 11071–11078. doi:10.1021/jp8042895
Robak, K., and Balcerek, M. (2018). Review of second generation bioethanol production from residual biomass. Food Technol. Biotechnol. 56, 174–187. doi:10.17113/ftb.56.02.18.5428
Rodrigues Mota, T., Matias de Oliveira, D., Marchiosi, R., Ferrarese-Filho, O., and Dantas dos Santos, W., (2018). Plant cell wall composition and enzymatic deconstruction. AIMS Bioeng. 5, 63–77. doi:10.3934/bioeng.2018.1.63
Rodríguez, O., Cristóvão, R. O., Tavares, A. P. M., and Macedo, E. A. (2011). Study of the alkyl chain length on laccase stability and enzymatic kinetic with imidazolium ionic liquids. Appl. Biochem. Biotechnol. 164, 524–533. doi:10.1007/s12010-010-9154-2
Ryu, K., Hwang, S. Y., Kim, K. H., Kang, J. H., and Lee, E. K. (2008). Functionality improvement of fungal lignin peroxidase by DNA shuffling for 2,4-dichlorophenol degradability and H2O2 stability. J. Biotechnol. 133, 110–115. doi:10.1016/j.jbiotec.2007.09.008
Salvador, Â. C., Santos, M. da C., and Saraiva, J. A. (2010). Effect of the ionic liquid [bmim]Cl and high pressure on the activity of cellulase. Green Chem. 12, 632. doi:10.1039/b918879g
Sanfilippo, C., D’Antona, N., and Nicolosi, G. (2004). Chloroperoxidase from Caldariomyces fumago is active in the presence of an ionic liquid as co-solvent. Biotechnol. Lett. 26, 1815–1819. doi:10.1007/s10529-004-5087-6
Santos, R. B., Lee, J. M., Jameel, H., Chang, H.-M., and Lucia, L. A. (2012). Effects of hardwood structural and chemical characteristics on enzymatic hydrolysis for biofuel production. Bioresour. Technol. 110, 232–238. doi:10.1016/j.biortech.2012.01.085
Sathendra, E. R., Praveenkumar, R., Gurunathan, B., Chozhavendhan, S., and Jayakumar, M. (2022). “Refining lignocellulose of second-generation biomass waste for bioethanol production,” in Biofuels and bioenergy (Elsevier), 87–110. doi:10.1016/B978-0-323-85269-2.00016-2
Schindl, A., Hagen, M. L., Muzammal, S., Gunasekera, H. A. D., and Croft, A. K. (2019). Proteins in ionic liquids: reactions, applications, and futures. Front. Chem. 7, 347. doi:10.3389/fchem.2019.00347
Sharma, H. K., Xu, C., and Qin, W. (2017). Biological pretreatment of lignocellulosic biomass for biofuels and bioproducts: an overview. Waste Biomass Valor 10, 235–251. doi:10.1007/s12649-017-0059-y
Shekiro, J., Kuhn, E. M., Nagle, N. J., Tucker, M. P., Elander, R. T., and Schell, D. J. (2014). Characterization of pilot-scale dilute acid pretreatment performance using deacetylated corn stover. Biotechnol. Biofuels 7, 23. doi:10.1186/1754-6834-7-23
Shipovskov, S., Gunaratne, H. Q. N., Seddon, K. R., and Stephens, G. (2008). Catalytic activity of laccases in aqueous solutions of ionic liquids. Green Chem. 10, 806. doi:10.1039/b716369j
Silveira, M. H. L., Morais, A. R. C., da Costa Lopes, A. M., Olekszyszen, D. N., Bogel-Łukasik, R., Andreaus, J., et al. (2015). Current pretreatment technologies for the development of cellulosic ethanol and biorefineries. ChemSusChem 8, 3366–3390. doi:10.1002/cssc.201500282
Sindhu, R., Pandey, A., and Binod, P. (2015). “Alkaline treatment,” in Pretreatment of biomass (Elsevier), 51–60. doi:10.1016/B978-0-12-800080-9.00004-9
Smiglak, M., Reichert, W. M., Holbrey, J. D., Wilkes, J. S., Sun, L., Thrasher, J. S., et al. (2006). Combustible ionic liquids by design: is laboratory safety another ionic liquid myth? Chem. Commun., 2554–2556. doi:10.1039/b602086k
Soltanian, S., Aghbashlo, M., Almasi, F., Hosseinzadeh-Bandbafha, H., Nizami, A.-S., Ok, Y. S., et al. (2020). A critical review of the effects of pretreatment methods on the exergetic aspects of lignocellulosic biofuels. Energy Convers. Manag. 212, 112792. doi:10.1016/j.enconman.2020.112792
Sorek, N., Yeats, T. H., Szemenyei, H., Youngs, H., and Somerville, C. R. (2014). The implications of lignocellulosic biomass chemical composition for the production of advanced biofuels. Bioscience 64, 192–201. doi:10.1093/biosci/bit037
Souza, T. V., Araujo, J. N., da Silva, V. M., Liberato, M. V., Pimentel, A. C., Alvarez, T. M., et al. (2016). Chemical stability of a cold-active cellulase with high tolerance toward surfactants and chaotropic agent. Biotechnol. Rep. (Amst) 9, 1–8. doi:10.1016/j.btre.2015.11.001
Sriariyanun, M., Tantayotai, P., Yasurin, P., Pornwongthong, P., and Cheenkachorn, K. (2016). Production, purification and characterization of an ionic liquid tolerant cellulase from Bacillus sp. isolated from rice paddy field soil. Electron. J. Biotechnol. 19, 23–28. doi:10.1016/j.ejbt.2015.11.002
Stevens, J. C., Das, L., Mobley, J. K., Asare, S. O., Lynn, B. C., Rodgers, D. W., et al. (2019). Understanding laccase-ionic liquid interactions toward biocatalytic lignin conversion in aqueous ionic liquids. ACS Sustain. Chem. Eng. 7, 15928–15938. doi:10.1021/acssuschemeng.9b02151
Stevens, J. C., Rodgers, D. W., C Dumon, C., and Shi, J. (2020a). Characterization and enzyme engineering of a hyperthermophilic laccase toward improving its activity in ionic liquid. Available at: http://apps.webofknowledge.com.libproxy.berkeley.edu/full_record.do?product=WOS&search_mode=GeneralSearch&qid=1&SID=6BZYE4sUTCIKzrygHLV&page=1&doc=4 (Accessed December 17, 2020).
Stevens, J. C., Rodgers, D. W., Dumon, C., and Shi, J. (2020b). Characterization and enzyme engineering of a hyperthermophilic laccase toward improving its activity in ionic liquid. Front. Energy Res. 8, 158. doi:10.3389/fenrg.2020.00158
Stock, F., Hoffmann, J., Ranke, J., Störmann, R., Ondruschka, B., and Jastorff, B. (2004). Effects of ionic liquids on the acetylcholinesterase – A structure–activity relationship consideration. Green Chem. 6, 286–290. doi:10.1039/B402348J
Sun, J., Konda, N. V. S. N. M., Parthasarathi, R., Dutta, T., Valiev, M., Xu, F., et al. (2017a). One-pot integrated biofuel production using low-cost biocompatible protic ionic liquids. Green Chem. 19, 3152–3163. doi:10.1039/C7GC01179B
Sun, J., Konda, N. V. S. N. M., Shi, J., Parthasarathi, R., Dutta, T., Xu, F., et al. (2016). CO2 enabled process integration for the production of cellulosic ethanol using bionic liquids. Energy Environ. Sci. 9, 2822–2834. doi:10.1039/C6EE00913A
Sun, J., Liu, H., Yang, W., Chen, S., and Fu, S. (2017b). Molecular mechanisms underlying inhibitory binding of alkylimidazolium ionic liquids to laccase. Molecules 22, 1353. doi:10.3390/molecules22081353
Sun, J., Shi, J., Murthy Konda, N. V. S. N., Campos, D., Liu, D., Nemser, S., et al. (2017c). Efficient dehydration and recovery of ionic liquid after lignocellulosic processing using pervaporation. Biotechnol. Biofuels 10, 154. doi:10.1186/s13068-017-0842-9
Sun, N., Parthasarathi, R., Socha, A. M., Shi, J., Zhang, S., Stavila, V., et al. (2014). Understanding pretreatment efficacy of four cholinium and imidazolium ionic liquids by chemistry and computation. Green Chem. 16, 2546–2557. doi:10.1039/C3GC42401D
Sun, N., Rahman, M., Qin, Y., Maxim, M. L., Rodríguez, H., and Rogers, R. D. (2009). Complete dissolution and partial delignification of wood in the ionic liquid 1-ethyl-3-methylimidazolium acetate. Green Chem. 11, 646. doi:10.1039/b822702k
Tantayotai, P., Rachmontree, P., Rodiahwati, W., Rattanaporn, K., and Sriariyanun, M. (2016). Production of ionic liquid-tolerant cellulase produced by microbial consortium and its application in biofuel production. Energy Procedia 100, 155–159. doi:10.1016/j.egypro.2016.10.158
Tavares, A. P. M., Rodríguez, O., Fernández-Fernández, M., Domínguez, A., Moldes, D., Sanromán, M. A., et al. (2013). Immobilization of laccase on modified silica: stabilization, thermal inactivation and kinetic behaviour in 1-ethyl-3-methylimidazolium ethylsulfate ionic liquid. Bioresour. Technol. 131, 405–412. doi:10.1016/j.biortech.2012.12.139
Tavares, A. P. M., Rodriguez, O., and Macedo, E. A. (2008). Ionic liquids as alternative co-solvents for laccase: study of enzyme activity and stability. Biotechnol. Bioeng. 101, 201–207. doi:10.1002/bit.21866
Thomas, M. F., Li, L.-L., Handley-Pendleton, J. M., van der Lelie, D., Dunn, J. J., and Wishart, J. F. (2011). Enzyme activity in dialkyl phosphate ionic liquids. Bioresour. Technol. 102, 11200–11203. doi:10.1016/j.biortech.2011.09.069
Toledo, M. L., Pereira, M. M., Freire, M. G., Silva, J. P. A., Coutinho, J. A. P., and Tavares, A. P. M. (2019). Laccase activation in deep eutectic solvents. ACS Sustain. Chem. Eng. 7, 11806–11814. doi:10.1021/acssuschemeng.9b02179
Trivedi, N., Gupta, V., Kumar, M., Kumari, P., Reddy, C. R. K., and Jha, B. (2011). Solvent tolerant marine bacterium Bacillus aquimaris secreting organic solvent stable alkaline cellulase. Chemosphere 83, 706–712. doi:10.1016/j.chemosphere.2011.02.006
Trivedi, N., Gupta, V., Reddy, C. R. K., and Jha, B. (2013). Detection of ionic liquid stable cellulase produced by the marine bacterium Pseudoalteromonas sp. isolated from brown alga Sargassum polycystum C. Agardh. Bioresour. Technol. 132, 313–319. doi:10.1016/j.biortech.2013.01.040
Turner, M. B., Spear, S. K., Huddleston, J. G., Holbrey, J. D., and Rogers, R. D. (2003). Ionic liquid salt-induced inactivation and unfolding of cellulase from Trichoderma reesei. Green Chem. 5, 443. doi:10.1039/b302570e
Usmani, Z., Sharma, M., Gupta, P., Karpichev, Y., Gathergood, N., Bhat, R., et al. (2020). Ionic liquid based pretreatment of lignocellulosic biomass for enhanced bioconversion. Bioresour. Technol. 304, 123003. doi:10.1016/j.biortech.2020.123003
van den Heuvel, R. H. H., Fraaije, M. W., Laane, C., and van Berkel, W. J. H. (2001). Enzymatic synthesis of vanillin. J. Agric. Food Chem. 49, 2954–2958. doi:10.1021/jf010093j
Vane, C. H., Drage, T. C., and Snape, C. E. (2006). Bark decay by the white-rot fungus lentinula edodes: polysaccharide loss, lignin resistance and the unmasking of suberin. Int. Biodeterior. Biodegrad. 57, 14–23. doi:10.1016/j.ibiod.2005.10.004
Vasconcelos, M. H., Mendes, F. M., Ramos, L., Dias, M. O. S., Bonomi, A., Jesus, C. D. F., et al. (2020). Techno-economic assessment of bioenergy and biofuel production in integrated sugarcane biorefinery: identification of technological bottlenecks and economic feasibility of dilute acid pretreatment. Energy 199, 117422. doi:10.1016/j.energy.2020.117422
Vitz, J., Erdmenger, T., Haensch, C., and Schubert, U. S. (2009). Extended dissolution studies of cellulose in imidazolium based ionic liquids. Green Chem. 11, 417. doi:10.1039/b818061j
Wahlström, R. M., and Suurnäkki, A. (2015). Enzymatic hydrolysis of lignocellulosic polysaccharides in the presence of ionic liquids. Green Chem. 17, 694–714. doi:10.1039/C4GC01649A
Wallraf, A.-M., Liu, H., Zhu, L., Khalfallah, G., Simons, C., Alibiglou, H., et al. (2018). A loop engineering strategy improves laccase lcc2 activity in ionic liquid and aqueous solution. Green Chem. 20, 2801–2812. doi:10.1039/C7GC03776G
Wan, C., and Li, Y. (2012). Fungal pretreatment of lignocellulosic biomass. Biotechnol. Adv. 30, 1447–1457. doi:10.1016/j.biotechadv.2012.03.003
Wang, X., Li, H., Cao, Y., and Tang, Q. (2011a). Cellulose extraction from wood chip in an ionic liquid 1-allyl-3-methylimidazolium chloride (AmimCl). Bioresour. Technol. 102, 7959–7965. doi:10.1016/j.biortech.2011.05.064
Wang, Y., Radosevich, M., Hayes, D., and Labbé, N. (2011b). Compatible ionic liquid-cellulases system for hydrolysis of lignocellulosic biomass. Biotechnol. Bioeng. 108, 1042–1048. doi:10.1002/bit.23045
Wolski, P. W., Dana, C. M., Clark, D. S., and Blanch, H. W. (2016). Engineering ionic liquid-tolerant cellulases for biofuels production. Protein Eng. Des. Sel. 29, 117–122. doi:10.1093/protein/gzv066
Wolski, P. W. (2013). Engineering and identification of ionic liquid-tolerant cellulases for biofuels production.
Wu, B., Zhao, Y., and Gao, P. J. (2006). A new approach to measurement of saccharifying capacities of crude cellulase. BioResources 1, 189–200. doi:10.15376/biores.1.2.189-200
Xia, M., Peng, M., Xue, D., Cheng, Y., Li, C., Wang, D., et al. (2020). Development of optimal steam explosion pretreatment and highly effective cell factory for bioconversion of grain vinegar residue to butanol. Biotechnol. Biofuels 13, 111. doi:10.1186/s13068-020-01751-7
Xu, F., Sun, J., Konda, N. V. S. N. M., Shi, J., Dutta, T., Scown, C. D., et al. (2016a). Transforming biomass conversion with ionic liquids: process intensification and the development of a high-gravity, one-pot process for the production of cellulosic ethanol. Energy Environ. Sci. 9, 1042–1049. doi:10.1039/C5EE02940F
Xu, J., He, B., Wu, B., Wang, B., Wang, C., and Hu, L. (2014). An ionic liquid tolerant cellulase derived from chemically polluted microhabitats and its application in in situ saccharification of rice straw. Bioresour. Technol. 157, 166–173. doi:10.1016/j.biortech.2014.01.102
Xu, J., Liu, X., He, J., Hu, L., Dai, B., and Wu, B. (2015a). Enzymatic in situ saccharification of rice straw in aqueous-ionic liquid media using encapsulated Trichoderma aureoviride cellulase. J. Chem. Technol. Biotechnol. 90, 57–63. doi:10.1002/jctb.4458
Xu, J. (2015). “Microwave pretreatment,” in Pretreatment of biomass (Elsevier), 157–172. doi:10.1016/B978-0-12-800080-9.00009-8
Xu, J., Sheng, Z., Wang, X., Liu, X., Xia, J., Xiong, P., et al. (2016b). Enhancement in ionic liquid tolerance of cellulase immobilized on PEGylated graphene oxide nanosheets: application in saccharification of lignocellulose. Bioresour. Technol. 200, 1060–1064. doi:10.1016/j.biortech.2015.10.070
Xu, J., Wang, X., Hu, L., Xia, J., Wu, Z., Xu, N., et al. (2015b). A novel ionic liquid-tolerant Fusarium oxysporum BN secreting ionic liquid-stable cellulase: consolidated bioprocessing of pretreated lignocellulose containing residual ionic liquid. Bioresour. Technol. 181, 18–25. doi:10.1016/j.biortech.2014.12.080
Xu, J., Wang, X., Liu, X., Xia, J., Zhang, T., and Xiong, P. (2016c). Enzymatic in situ saccharification of lignocellulosic biomass in ionic liquids using an ionic liquid-tolerant cellulases. Biomass Bioenergy 93, 180–186. doi:10.1016/j.biombioe.2016.07.019
Yadav, A., Sharma, V., Tsai, M.-L., Chen, C.-W., Sun, P.-P., Nargotra, P., et al. (2023). Development of lignocellulosic biorefineries for the sustainable production of biofuels: towards circular bioeconomy. Bioresour. Technol. 381, 129145. doi:10.1016/j.biortech.2023.129145
Yang, B., Dai, Z., Ding, S.-Y., and Wyman, C. E. (2011). Enzymatic hydrolysis of cellulosic biomass. Biofuels 2, 421–449. doi:10.4155/bfs.11.116
Yang, Z. (2009). Hofmeister effects: an explanation for the impact of ionic liquids on biocatalysis. J. Biotechnol. 144, 12–22. doi:10.1016/j.jbiotec.2009.04.011
Yoshida, M., Liu, Y., Uchida, S., Kawarada, K., Ukagami, Y., Ichinose, H., et al. (2008). Effects of cellulose crystallinity, hemicellulose, and lignin on the enzymatic hydrolysis of Miscanthus sinensis to monosaccharides. Biosci. Biotechnol. Biochem. 72, 805–810. doi:10.1271/bbb.70689
Youn, H.-D., Hah, Y. C., and Kang, S.-O. (1995). Role of laccase in lignin degradation by white-rot fungi. FEMS Microbiol. Lett. 132, 183–188. doi:10.1111/j.1574-6968.1995.tb07831.x
Yu, X., Zou, F., Li, Y., Lu, L., Huang, X., and Qu, Y. (2013). Effect of three trifluoromethanesulfonate ionic liquids on the activity, stability and conformation of laccase. Int. J. Biol. Macromol. 56, 62–68. doi:10.1016/j.ijbiomac.2013.02.005
Zang, G., Shah, A., and Wan, C. (2020). Techno-economic analysis of an integrated biorefinery strategy based on one-pot biomass fractionation and furfural production. J. Clean. Prod. 260, 120837. doi:10.1016/j.jclepro.2020.120837
Zavrel, M., Bross, D., Funke, M., Büchs, J., and Spiess, A. C. (2009). High-throughput screening for ionic liquids dissolving (ligno-)cellulose. Bioresour. Technol. 100, 2580–2587. doi:10.1016/j.biortech.2008.11.052
Zhang, F., Bunterngsook, B., Li, J.-X., Zhao, X.-Q., Champreda, V., Liu, C.-G., et al. (2019). “Regulation and production of lignocellulolytic enzymes from Trichoderma reesei for biofuels production,” in Advances in bioenergy (Elsevier), 79–119. doi:10.1016/bs.aibe.2019.03.001
Zhang, K., Pei, Z., and Wang, D. (2016). Organic solvent pretreatment of lignocellulosic biomass for biofuels and biochemicals: A review. Bioresour. Technol. 199, 21–33. doi:10.1016/j.biortech.2015.08.102
Zhang, T., Datta, S., Eichler, J., Ivanova, N., Axen, S. D., Kerfeld, C. A., et al. (2011). Identification of a haloalkaliphilic and thermostable cellulase with improved ionic liquid tolerance. Green Chem. 13, 2083. doi:10.1039/c1gc15193b
Zhao, D., Liao, Y., and Zhang, Z. (2007). Toxicity of ionic liquids. Clean. Soil Air Water 35, 42–48. doi:10.1002/clen.200600015
Zhao, H. (2006). Are ionic liquids kosmotropic or chaotropic? An evaluation of available thermodynamic parameters for quantifying the ion kosmotropicity of ionic liquids. J. Chem. Technol. Biotechnol. 81, 877–891. doi:10.1002/jctb.1449
Zhao, H., Baker, G. A., Song, Z., Olubajo, O., Crittle, T., and Peters, D. (2008). Designing enzyme-compatible ionic liquids that can dissolve carbohydrates. Green Chem. 10, 696. doi:10.1039/b801489b
Zhao, H., Baker, G. A., Song, Z., Olubajo, O., Zanders, L., and Campbell, S. M. (2009). Effect of ionic liquid properties on lipase stabilization under microwave irradiation. J. Mol. Catal. B Enzym. 57, 149–157. doi:10.1016/j.molcatb.2008.08.006
Zhao, H., Olubajo, O., Song, Z., Sims, A. L., Person, T. E., Lawal, R. A., et al. (2006). Effect of kosmotropicity of ionic liquids on the enzyme stability in aqueous solutions. Bioorg. Chem. 34, 15–25. doi:10.1016/j.bioorg.2005.10.004
Zhao, J., Wilkins, M. R., and Wang, D. (2022). A review on strategies to reduce ionic liquid pretreatment costs for biofuel production. Bioresour. Technol. 364, 128045. doi:10.1016/j.biortech.2022.128045
Zhao, L., Cao, G.-L., Wang, A.-J., Ren, H.-Y., Dong, D., Liu, Z.-N., et al. (2012). Fungal pretreatment of cornstalk with Phanerochaete chrysosporium for enhancing enzymatic saccharification and hydrogen production. Bioresour. Technol. 114, 365–369. doi:10.1016/j.biortech.2012.03.076
Zhou, J., Sui, H., Jia, Z., Yang, Z., He, L., and Li, X. (2018). Recovery and purification of ionic liquids from solutions: A review. RSC Adv. 8, 32832–32864. doi:10.1039/C8RA06384B
Zhu, D., Liang, N., Zhang, R., Ahmad, F., Zhang, W., Yang, B., et al. (2020). Insight into depolymerization mechanism of bacterial laccase for lignin. ACS Sustain. Chem. Eng. 8, 12920–12933. doi:10.1021/acssuschemeng.0c03457
Zoghlami, A., and Paës, G. (2019). Lignocellulosic biomass: understanding recalcitrance and predicting hydrolysis. Front. Chem. 7, 874. doi:10.3389/fchem.2019.00874
Glossary
Keywords: enzymes, ionic liquids, biorefineries, cellulose, lignin, pretreament, lignocellulose, biomass
Citation: Wolski P, Blankenship BW, Umar A, Cabrera M, Simmons BA, Sale KL and Achinivu EC (2023) Factors that influence the activity of biomass-degrading enzymes in the presence of ionic liquids—a review. Front. Energy Res. 11:1212719. doi: 10.3389/fenrg.2023.1212719
Received: 26 April 2023; Accepted: 09 August 2023;
Published: 24 August 2023.
Edited by:
Yunqiao Pu, Oak Ridge National Laboratory (DOE), United StatesReviewed by:
Sujit Jagtap, University of Illinois at Urbana-Champaign, United StatesRafal Lukasik, Łukasiewicz Research Network, Poland
Copyright © 2023 Wolski, Blankenship, Umar, Cabrera, Simmons, Sale and Achinivu. This is an open-access article distributed under the terms of the Creative Commons Attribution License (CC BY). The use, distribution or reproduction in other forums is permitted, provided the original author(s) and the copyright owner(s) are credited and that the original publication in this journal is cited, in accordance with accepted academic practice. No use, distribution or reproduction is permitted which does not comply with these terms.
*Correspondence: Ezinne C. Achinivu, ZWFjaGluaXZ1QGxibC5nb3Y=, YWNoaW5pdnVAdWljLmVkdQ==
†Present address: Ezinne C. Achinivu, Department of Chemical Engineering, University of Illinois at Chicago, Chicago, IL, United States
‡These authors have contributed equally to this work