- 1Grassland Science and Renewable Plant Resources (GNR), Universität Kassel, Witzenhausen, Germany
- 2Institute for Integrative Ecological Transition (In2ET), Neu-Eichenberg, Germany
- 3Leibniz Institute for Agricultural Engineering and Bioeconomy (ATB), Potsdam, Germany
Biochar is an emerging biomaterial for managing residual biomass while simultaneously sequestering carbon. To extend the biochar value chain, applying biochar to enhance anaerobic digestion (AD) processes is gaining attention in the context of a circular economy and cascading use of biomass. However, the comparative effects of various biochar dosages under normal and severe AD conditions are still unclear. To further our understanding of its potential application, this work investigated the impact of adding various biochar dosages on AD processes under normal and high substrate loadings. Three inoculum-to-substrate ratios (ISRs): one representing normal substrate loading (ISR 2) and two representing substrate overloading (ISR 1 and 0.5) were investigated. Each substrate loading rate was tested with a biochar dosage of 0% (control), 10%, and 25% based on substrate volatile solids. The results revealed that under the severe condition of high substrate overload (ISR 0.5), a high biochar dosage of 25% significantly increased cumulative methane production by 5.6% (p = 0.06) when compared to the control. Under the same condition (ISR 0.5, 25%), the time required to achieve a particular extent of ultimate methane potential was significantly reduced (p = 0.04), indicating that the methane production rate was increased. At ISR 0.5, the increase of process stability was also significant with 25% biochar addition, while the control (0%) and 10% biochar addition exhibited high variance among replicates. However, biochar did not affect AD processes under normal substrate loading (ISR 2) and mild substrate overload (ISR 1). Thus, a positive effect of biochar on the AD process was only observed under severe conditions with the highest biochar dosage. Future works should consider optimising substrate loadings and biochar dosages under real conditions when testing the practical application of biochar addition in AD processes.
1 Introduction
Biochar (BC) is a carbon-rich product of the thermal breakdown of biomass in the absence of oxygen (Lehmann and Joseph, 2009). During pyrolysis, energy can be captured as syngas (Bachmann et al., 2023) and the produced biochar can be used for various applications, such as a soil amendment in agriculture (Asirifi et al., 2021; Heinrich et al., 2023a; Asirifi et al., 2023), for wastewater treatment (Kaetzl et al., 2019; Joseph et al., 2020), or an additive in anaerobic digestion processes (Mumme et al., 2014; Shen et al., 2015). Furthermore, the recalcitrant carbon remaining within biochar allows for CO2 sequestration (Spokas, 2010), and thus contributes to climate change mitigation. Additionally, several suitable biomass can be used as the feedstock for biochar production, especially residue woody biomass, that includes sawdust, wood chips, or forestry residues, which are available in large quantities. For example, Europe currently has an estimated annual production of wood residues ca. 500 million m3 with an energy equivalent of approximately 3 EJ (exajoules) (Thiffault et al., 2023). The cascading use of wood residues in the context of a circular economy becomes an attractive approach aiming to increase biomass value, carbon sequestration, and prioritize other beneficial uses of biomass over energy utilisation alone (Olsson et al., 2016; Fehrenbach et al., 2017). Hence, using wood residues to produce biochar has great potential to fill the gap of applications, by combining renewable energy production with carbon storage and material utilisation.
Anaerobic digestion (AD) has been widely applied for the treatment of organic wastes, such as agricultural residue, sewage sludge, and household biowaste, and for the production of biogas. Thus, AD contributes to waste management and renewable energy production. A variety of parameters, such as temperature, pH value, composition of substrate, and organic loading rates can have an impact on how well AD processes work (Rosenwinkel et al., 2015). For instance, organic overloading can induce acid stress in an AD process, consequently inhibiting methane production (Dai et al., 2015; Xu et al., 2018). In the batch mode, the organic loading rate can be expressed as the inoculum-to-substrate ratio (ISR). Inadequate ISRs result in a low substrate-to-methane conversion rate (Raposo et al., 2009; Jensen et al., 2011; Filer et al., 2019), and sometimes even in a complete inhibition of methane production (Dang et al., 2017; Wang et al., 2017). However, the extent of inhibition is dependent on substrate types and the used ISRs (Angelidaki et al., 2009; Holliger et al., 2016). As the hydrolysis process is considered the rate-limiting phase of AD processes, the hydrolysis rate of a substrate can also affect the methane production rate or kinetics (Pavlostathis and Giraldo-Gomez, 1991; Koch and Drewes, 2014). Through co-digestion, modified reactors, or the addition of certain additives, such as biochars, it is possible to overcome such inhibitions or disturbances in AD processes (Mao et al., 2015; Ye et al., 2018).
Several studies have investigated the use of biochars to enhance anaerobic digestion (AD) processes. Broadly, the existing studies can be grouped into two categories: 1) those that have investigated biochar dosages (Shen et al., 2015; Fagbohungbe et al., 2016; Wang et al., 2017; Ovi et al., 2022) and 2) others that have studied the effects of biochars under severe conditions that have resulted from various substrate types or loading rates (Luo et al., 2015; Fagbohungbe et al., 2016; Wang et al., 2017; Zhang et al., 2019; Pan et al., 2022). For example, Wang et al. (2017) investigated the effects of three biochar dosages on AD under a high organic overload of kitchen waste and animal manure. They found that digestion was only initiated with biochar addition and attributed their results to the acid-buffering capacity of biochar. However, the used biochar dosages were up to three times more than the substrate concentration, which is not achievable in practical applications. Fagbohungbe et al. (2016) also investigated various biochar dosages using citrus peel containing inhibitor limonene as the substrate. The results showed that methane yield increased slightly with biochar addition, and the methanogenic lag phases decreased with increasing biochar dosages from 100% to 300%. The biochar dosage was also significantly high for practical applications considering the production cost of biochar, which ranges from 100 to 1,000 US$ per tonne (Haubold-Rosar et al., 2016; Nematian et al., 2021). Other studies have investigated effects of using biochar under severe conditions. For example, Deng et al. (2021) investigated an anaerobically difficult degradable substrate draff or brewer’s spent grain containing a high content of lignocellulosic materials and found a slight increase in methane yield. In another study, Luo et al. (2015) investigated the effects of biochar under several high organic overloading rates in the batch mode and found that biochar reduced the methanogenic lag phase and boosted the maximum methane production rate. The same authors analysed the microbial consortium and found an enrichment of methanogens in biochar-amended systems, which could explain the improved AD processes via mechanisms such as direct interspecies electron transfer (DIET) mediated by methanogens (Liu et al., 2012; Chen et al., 2014).
However, the experimental conditions used in earlier studies vary considerably with respect to biochar dosages, substrate types, and loading rates. This makes the comparison of results among studies difficult. A detailed summary of recent studies on biochar and its application in AD process focussing on their experimental setups are found in Supplementary Table S1. Moreover, most existing studies have used a very high biochar dosage that was much more than the substrate concentration, which is unrealistic considering the cost of biochar and the working volume of digesters, which would be reduced by biochar and could thus result in a lower substrate throughput. The used substrate loadings in most previous studies have also been very high, thereby causing strong inhibitions, while those that had applied low substrate loading used anaerobically difficult degradable substrate leading to challenges in AD processes. In summary, previous studies that investigated the effects of biochar on AD processes applied 1) unrealistic biochar dosages which were higher than those achievable under practical conditions and 2) severe conditions resulting from substrate loading rates or types that occurred rarely in practical applications. Thus, most of these earlier studies reported significant biochar effects on AD processes, but these findings are not transferable to real field applications. To address this gap, the present study used batch experiments to understand the effects of biochar on AD using practical applicable dosages to mimic realistic conditions. Therefore, the aim of the present study was to examine the effects of adding biochar in anaerobic digestion processes under the influences of 1) practical applicable biochar dosages, 2) normal substrate loadings, and 3) high substrate overloading. We used a biochar derived from oak wood and a commercial dog food as a substitute for food waste, which can be obtained with comparable quality worldwide. This enables the experiments to be replicated in international laboratories. In addition, the results of the present study were compared with those in the literature on biochar dosages, substrate types, and loading rates. This critical evaluation provides further insights on the sources of inconsistencies between the present results and those reported in earlier studies.
2 Materials and methods
2.1 Biochar production
Oak sawdust from a local sawmill was used as feedstock for biochar production. This was adjusted to a water content of approximately 12% and then pelletized with additive—2% potato starch on a dry weight basis (PP230UG, qteck GmbH, Bergen, Germany). The obtained pellets had a diameter of 6 mm and length of 10 mm with a water content of approximately 8%. The pellets were then pyrolyzed via a continuously operated auger reactor (PYREKA, PYREG GmbH, Dörth, Germany) at a temperature of 750°C (w750) and an average solid retention time of 30 min, mimicking a full-scale pyrolysis plant and enabling high transferability in practical applications (Joseph et al., 2020). The reactor was flushed with nitrogen (7 L/min) to avoid uncontrolled oxidation of biochar. The used high pyrolysis temperature and moderate retention time allowed high graphitization (Lehmann and Joseph, 2009; Zhang et al., 2015), which was supposed to improve methane production processes (Sun et al., 2017; Johnravindar et al., 2021). The biochar was ground to less than 1 mm and dried at 105°C overnight before using it in batch experiments.
2.2 Inoculum and substrate
The inoculum source was a digested sludge obtained from an anaerobic mesophilic sewage sludge digester of a municipal wastewater treatment plant (Kassel, Germany, population equivalent (PE): 340,000). Fresh inoculum was obtained before the dewatering process and contained no large fibrous material. According to Holliger et al. (2016), no pre-treatment or degassing of the used inoculum was required. Furthermore, the residue methane yield of the blank (inoculum only) was determined as 38 NmL/gVS, which is lower than 20% of the total methane production and thus no degassing was required (Holliger et al., 2016). According to the German standard methods for the examination of water, wastewater, and sludge (DEV, 2020), the inoculum prior to AD tests had a total solids (TS) content of 1.9% of fresh matter (FM) and volatile solids (VS) content of 58.9% of TS. Electrical conductivity and pH values measured prior to the AD tests were 5.7 mS/cm and 7.4, respectively.
Commercial dog food pellets (Orlando Gourmet, Germany) were used as a substitute for food waste (Nakasaki et al., 2004; Dang et al., 2017; Koch et al., 2017), which can be obtained with comparable quality worldwide. This enables the experiments to be replicated in international laboratories. According to the producer, dog food comprised 25.0% crude protein, 16.0% crude lipid, 2.5% crude fibre, 6.5% ash, and the remaining (that included carbohydrates) accounted for 50.0% on a dry weight basis. The TS and VS content of the substrate were 91.7% of FM and 93.3% of TS, respectively. The substrate contained 44.6% carbon and 3.9% nitrogen on a dry weight basis, giving a C/N ratio of 11.5.
2.3 Batch experiments
Batch experiments were conducted to examine the short-term effects of using biochar in AD processes and thus did not include a microbiological analysis. This is because a steady state cannot be achieved in the batch mode. The experiments comprised three groups with different inoculum-to-substrate ratios (ISRs) based on VS. The three ISRs were 1) 2:1 (ISR 2) testing normal substrate loading, 2) 1:1 (ISR 1) representing a mild substrate overloading, and 3) 1:2 (ISR 0.5) representing a high substrate overloading. Each group consisted of one control treatment with only a substrate and inoculum, two biochar addition treatments with a substrate, and an inoculum and a biochar dosage of 10% and 25%, respectively. The biochar addition ratios were calculated based on the mass ratio of biochar total solids to substrate VS. The choice of biochar dosages was based on the amount of activated carbon that could land in the digester in wastewater treatment plants (Hollender et al., 2009; Joseph et al., 2020). This occurs when the activated carbon is either 1) directly added at the biological treatment stage, or 2) indirectly discharged at the biological stage after being used for the adsorption of micropollutants. Both cases lead to a carbon-enriched sewage sludge into the digester. The experiments were conducted in triplicate in two Gas Endeavour systems (Bioprocess Control Sweden AB) with a capacity of 30 bottles in total. The treatments used 27 bottles and the remaining 3 bottles were used to run two blank tests with the inoculum only and one positive test with cellulose and the inoculum. The details of the batch experimental conditions are presented in Table 1. All tests were conducted under a mesophilic condition (37°C) until the daily biogas production during three consecutive days was less than 0.5% of the total biogas production (VDI, 2016). The samples were stirred in cycles, that included 5 min mixing and 25 min resting (Koch et al., 2015). The headspace of all bottles was flushed with nitrogen gas at a flow rate of 2.5 L/min for 1 minute.
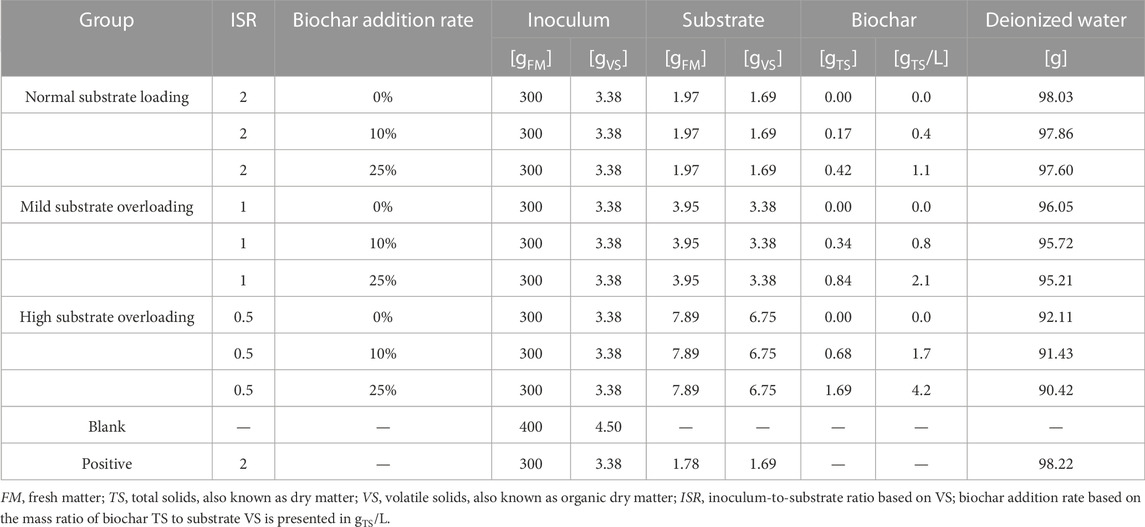
TABLE 1. Batch experimental conditions for different inoculum-to-substrate ratios without biochar addition (0%) and with biochar addition rates of 10% and 25%. All treatments were conducted in triplicate; a blank with only inoculum was conducted in duplicate, and a positive test with cellulose was conducted without replicates.
The used Gas Endeavour system (Bioprocess Control Sweden AB) consisted of 1) 15 glass bottles each of 500 mL, comprising a working volume of 400 mL and a headspace of approximately 100 mL per bottle; 2) two automatic gas detection units (Gas Endeavour with a resolution of 9 mL) with a working principle of liquid displacement and buoyancy; and 3) a CO2 and H2S absorption unit with a 3 M sodium hydroxide solution. The total biogas volume was measured with the first gas detection unit and then biogas was passed through the absorption unit, where CO2 and trace amounts of H2S were absorbed. The rest of the gas was then recorded as methane volume at the second gas detection unit. The difference between the first and second gas detection units was assumed to be the CO2 volume. The Gas Endeavour has a measuring precision of 99% and the absorption unit has a fixing efficiency greater than 98% according to the user manual. The Gas Endeavour’s embedded real-time data acquisition system recorded and automatically normalised the volume of biogas and methane to the standard temperature and pressure of 0°C and 1 atm. A more detailed description of the system can be found in the study proposed by Strömberg et al. (2014).
2.4 Analytical methods for biochar and biomass
The TS and ash content of biochar were measured following DIN EN 12902 (2005) at 105°C and 650°C, respectively. According to the European Biochar Certificate (EBC, 2023), the buffer capacity and pH that indicate the alkaline characteristics of biochar and electrical conductivity (EC) that indicates the soluble salts of biochar should be determined. Briefly, the pH was measured in suspension with 50 mL deionized water and 5 g air-dried biochar, and EC was also measured in the same liquid-to-solid ratio 10:1 (v/w) but in filtrate (Singh et al., 2017). Both pH and EC were measured using the WTW multiparameter metre 3420 (Xylem Analytics, Germany). The buffer capacity was determined by titrating with 0.5 M NaOH in a suspension with 10 mL 1 M HCL and 0.5 g air-dried biochar, and the results expressed as CaCO3 equivalents (Singh et al., 2017). The TS and ash content of biomass (oak wood) were measured following DIN EN ISO 18134-3 (2015) at 105°C and DIN EN ISO 18122 (2016) at 550°C, respectively. The C, H, N, and S contents of both biomass and biochar were determined in 150 mg of material on a weight basis using an elemental analyser (Elementar Analysensysteme GmbH, Hanau, Germany). The TS of biomass and biochar was also determined using the same sample. Oxygen (O) content was calculated based on the CHNS and ash content. The concentration of ash minerals (K, Ca, and Mg) was determined by an ICP-AES (SPECTRO Analytical Instruments GmbH, Kleve, Germany) analysis after microwave digestion with nitric acid. For biomass fibre analysis, neutral detergent fibre (NDF), acid detergent fibre (ADF), and acid detergent lignin (ADL) were determined by the method of Van Soest and Wine (1967), using an ANKOM200 Fibre Analyser (ANKOM Technologies, United States). The concentration of hemicellulose was calculated from the difference between NDF and ADF. The concentration of cellulose was calculated as the difference between ADF and ADL concentrations and expressed as dry weight percentage (Table 2). A more detailed description of the methods is found in the study by Nurk et al. (2016).
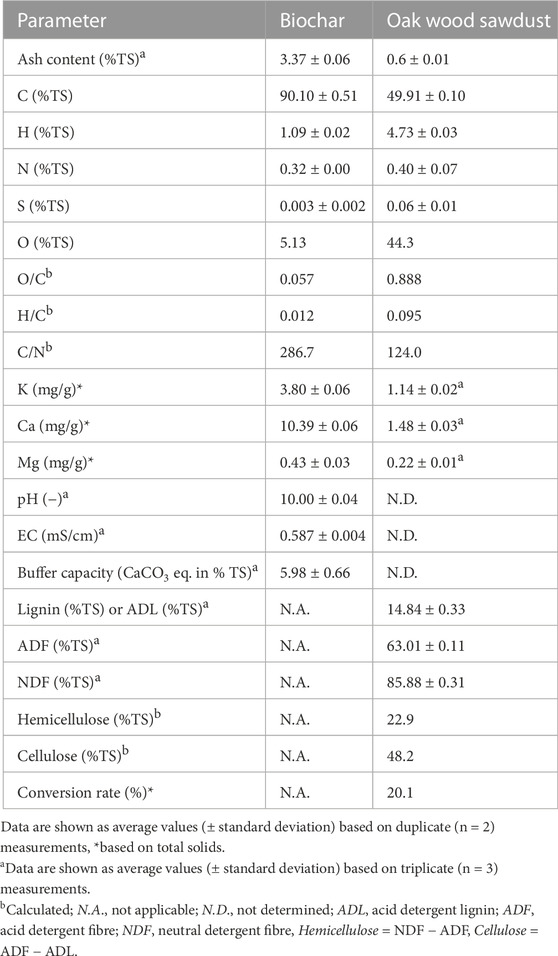
TABLE 2. Characteristics of biochar produced from oak sawdust at a pyrolysis temperature of 750°C and a retention time of 30 min and characteristics of the feedstock oak wood.
2.5 Theoretical biomethane potential and experimental methane yield
The maximum theoretical biomethane potential (BMP) was calculated from Equation 1, using organic fraction compositions (Raposo et al., 2011). It was expressed as NmL/(gVS,added).
According to VDI 4630 (VDI, 2016), the maximum theoretical biomethane potential cannot be achieved because approximately 8% of the substrate is used for biomass formation and is not available for methane production. Therefore, following Equation 1, the actual achievable BMP was calculated as 454.24 ± 14.81 NmL/(gVS,added). In the following text, theoretical BMP always means actual achievable BMP if there is no additional explanation. In addition, the 50%, 60%, 70%, and 80% quantiles of theoretical BMP accounted for 227.1 NmL, 272.5 NmL, 318.0 NmL, and 363.4 NmL, respectively.
The experimental methane yield of the added organic substrate was calculated following Eq. 2:
where Bexp. is the experimental methane yield expressed in NmL/(gVS,added), VS [NmL] is the cumulated methane volume from bottles with a substrate, VB [NmL] is the cumulated methane volume from blank bottles with the inoculum only, mI,S [gVS] is the amount of inoculum in substrate bottles, mI,B [gVS] is the amount of inoculum in blank bottles, and mS,S [gVS] is the organic matter of the added substrate in substrate bottles.
2.6 Statistical analysis
The normal distribution of data was checked using the Shapiro–Wilk test and the homogeneity of data variance was tested using the Levene’s test; both were also visually checked for double verification. The significance of differences in the mean were analysed using the two-way analysis of variance (ANOVA) to examine the effects of the experimental factors: biochar dosages and substrate loading rates. The linear regression analysis was performed for the important parameters: ultimate methane yield, time to reach particular extents of BMP, the removal of both TS and VS of the substrate, to determine the relationship between their extents over biochar dosages, substrate loading rates, and the interaction of these two experimental factors. All statistical analyses were done at a probability level p
3 Results
3.1 Biochar and biomass characteristics
The oak wood–derived biochar with high pyrolysis temperature 750°C (w750) and a moderate retention time of 30 min has a very low ash content at 3.4% and a very high carbon content at 90% on the dry weight basis and conversion rate of 20.1% (Table 2). It also has an alkaline pH value of 10 and a mild buffer capacity of 6% categorized into class 1 (from 0 to 3) (Camps-Arbestain et al., 2015). The biochar had an EC of 0.59 mS/cm, indicating soluble salts in biochar suspension, which is comparable to typical values of 0.07–1 mS/cm reported for woody biochars produced at pyrolysis temperatures of 450°C–700oC (Lehmann and Joseph, 2009; Singh et al., 2010; Rajkovich et al., 2012). The loss of the O and H ratio of biomass was very high during the pyrolysis processes due to the high pyrolysis temperature and moderate retention time, which resulted in the w750 biochar having very low O/C and H/C ratios with 0.057 and 0.012, respectively, indicating a high graphitization level (Tan et al., 2015; Masebinu et al., 2019; Chiappero et al., 2020) (Table 2). The graphitic structure of biochar implies a high conductivity of electrons (Lovley, 2017; Johnravindar et al., 2021), which could enhance the AD processes (Gahlot et al., 2020). In summary, the biochar used in this study shows favourable characteristics for addition in AD processes based on the alkaline properties and high graphitization level.
3.2 Methane production
The simulation of substrate overloading demonstrated that the development of methane production was inhibited with mild substrate overloading ISR 1 (t(99) = 2.22, p = 0.03) and, in particular, with high substrate overloading ISR 0.5 (t(99) = 15.93, p < 0.001) when compared to normal substrate loading ISR 2 (Figure 1A). Consequently, the time required to reach 50%, 60%, 70%, and 80% of the theoretical achievable BMP increased significantly. For instance, the time to achieve half of the BMP increased from an average of 37 h (ISR 2) to an estimated 68 h during treatments under mild substrate overloading (ISR 1) and to approximately 212 h for treatments with high substrate overloading (ISR 0.5). However, in treatments operating under a high substrate overloading (ISR 0.5), the addition of biochar at a dosage of 25% was observed to have a positive effect in reducing the time for achieving the particular extents of BMP (t(99) = −2.12, p = 0.04) (Figure 1B). But, in general, biochar addition did not significantly affect the development of methane production over time at either the 10% (t(99) = −0.15, p = 0.88) or 25% (t(99) = 0.10, p = 0.93) dosage (Figure 1A).
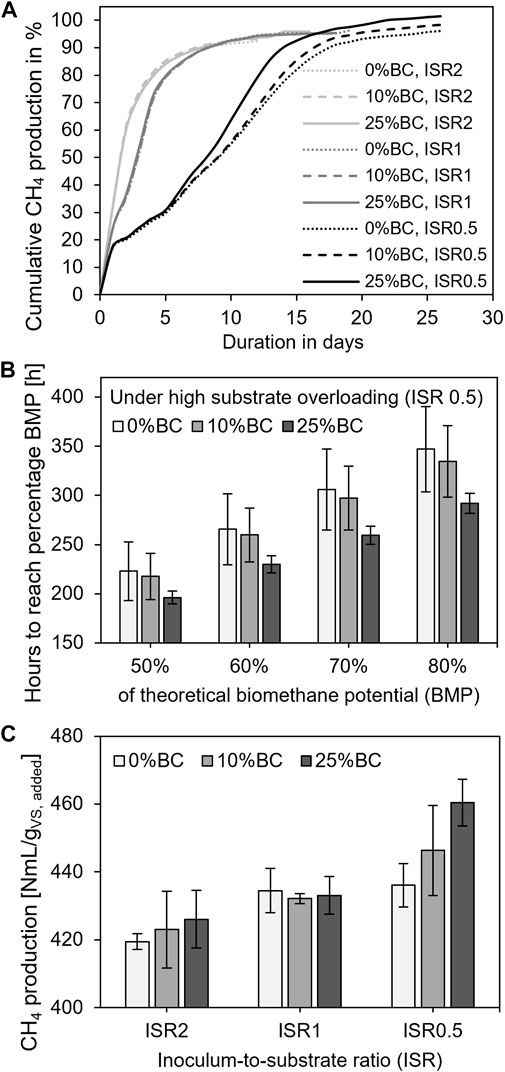
FIGURE 1. (A) Development of methane production of all nine treatments, where data are shown in the average of triplicate, (B) under high substrate overloading condition ISR 0.5, the time to reach different percentages of the actual achievable theoretical biomethane potential with different biochar dosages, where data are shown in the average of triplicate with standard deviation, and (C) methane yields (maximum cumulative methane production) are shown in the average of triplicate with standard deviation.
Under a high substrate overloading (ISR 0.5), the development of methane production was positively linked with the dosage of biochar that was added. Herein, by using a high biochar dosage of 25%, a rapid temporal development of methane production after day 7 was detectable (Figure 1A). A higher biochar dosage of 25% under ISR 0.5 further reduced the time required to reach 50%, 60%, 70%, and 80% of the BMP, whereas the addition of 10% biochar had no apparent influence on the time required to reach the particular extents of the BMP (Figure 1B). However, the addition of biochar did not result in a substantial reduction in the time required to reach a particular extent of BMP under conditions of normal substrate loading (ISR 2) and mild substrate overloading (ISR 1; Figure 1A). It implies that a significant promotion of methane production kinetics was obtained under high substrate overloading with a high biochar dosage.
The substrate loading rates had an significant effect on the ultimate methane yield, which was enhanced under both mild (ISR 1; t(18) = 2.36, p = 0.03) and high substrate overloading (ISR 0.5;t(18) = 2.62, p = 0.02) when compared to normal substrate loading (ISR 2; Figure 1C). While under high substrate overloading (ISR 0.5), the ultimate methane yield was improved significantly with the biochar dosage of 25% (t(18) = 1.97, p = 0.06) by 5.6% compared to its control, the ultimate methane yield was generally not affected by biochar addition at either 10% (t(18) = 0.57, p = 0.58) or 25% (t(18) = 1.04, p = 0.31) dosage when compared to the control without biochar addition.
3.3 Specific methane production rate
Multiple peaks demonstrated that under substrate overloading conditions (ISR 1 and 0.5), the methane production processes were inhibited, and the specific methane production rates decreased but could recover afterwards (Figure 2). The first peak of all curves illustrated that a maximum specific methane production rate was achieved at the beginning of the digestion tests and the maximum specific methane production rate decreased with an increasing substrate loading level (Figure 2). However, biochar had no impact on the maximum specific methane production rate. Additionally, under high substrate overloading (ISR 0.5), the specific methane production rate recovered rapidly with a high biochar dosage of 25% (Figure 2), and biochar did not affect the recovery of the specific methane production rate under mild substrate overloading (ISR 1).
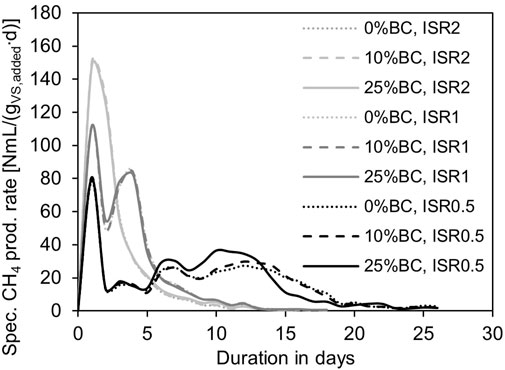
FIGURE 2. Development of specific methane production rates of each substrate loading rate and biochar addition, where data are shown in the average of triplicate.
3.4 Process stability
The variance among replicates of the same treatment demonstrated the process stability (Figures 3A–C). Under the condition of high substrate overloading (ISR 0.5), the methane production curves of the control group (without biochar) showed significantly higher variability during the process (Figure 3A) than those treatments that received 10% or 25% biochar (Figures 3B,C). As the biochar dosage increased, the variance among methane production curves decreased, i.e., process stability increased. The lowest variance among replicates was obtained in the treatment which received a biochar dosage of 25%. This indicates that biochar improves the process stability of the AD process under a substrate overloading condition and the process stability increases with increasing biochar dosage.
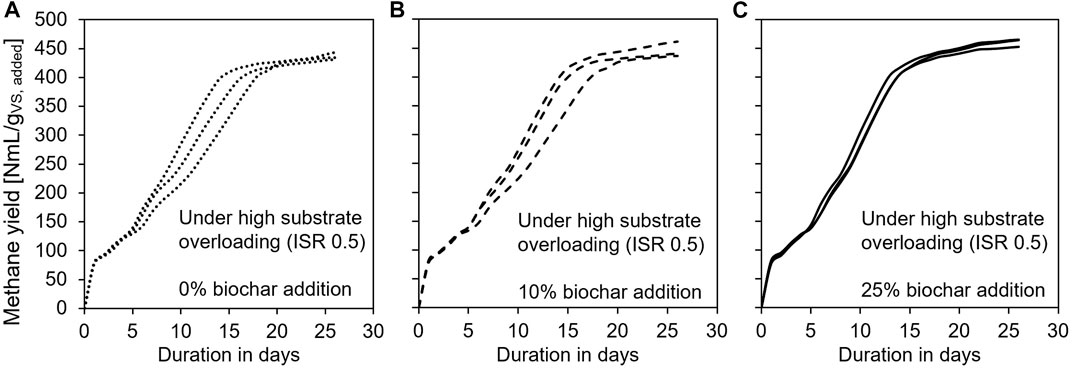
FIGURE 3. Methane production yield curves at substrate overloading condition ISR 0.5; all triplicate are illustrated (A) with 0% BC addition, (B) with 10% BC addition, and (C) with 25% BC addition.
3.5 Removal of volatile solids and total solids
The removal of TS of the substrate was not affected by either substrate loading rates (two-way ANOVA, F(2,18) = 2.54, p = 0.11) or biochar dosages (two-way ANOVA, F(2,18) = 2.94, p = 0.08; Figure 4A). By contrast, both ISRs (two-way ANOVA, F(2,18) = 8.55, p = 0.002) and biochar dosages (two-way ANOVA, F(2,18) = 8.19, p = 0.003) were found to have a significant effect on the removal of the substrate’s VS (Figure 4B). The removal of VS under high substrate overloading condition (ISR 0.5) was significantly smaller (t(18) = −3.03, p = 0.007) than that under normal substrate loading (ISR 2; Figure 4B). Generally, the highest VS removal rates were achieved at a biochar dosage of 25% (Figure 4B).
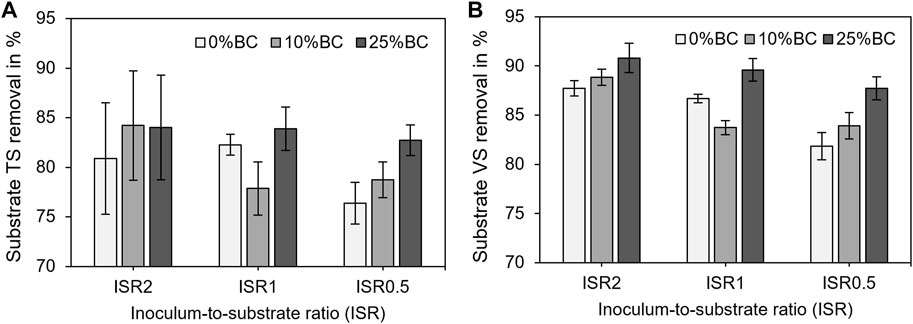
FIGURE 4. (A) Total solid removal rates of a substrate in the average of the triplicate with standard deviation, in which the TS part of biochar was subtracted by calculation and (B) volatile solid removal rates of a substrate in the average of triplicate with standard deviation and the VS part of biochar was subtracted by calculation.
4 Discussion
4.1 Effect of biochar on methane production
We studied the effects of biochar addition on methane production in a laboratory batch fermentation system by employing different biochar dosages and substrate loading conditions. The ultimate cumulative methane yield, i.e., BMP, is substrate dependent, and the batch test is a capable and cost-effective tool to determine it (Jensen et al., 2011; Koch et al., 2020). Usually, an inoculum-to-substrate ratio (ISR) between 2 and 4 is recommended based on the VS to conduct a standard BMP test (Holliger et al., 2016; VDI, 2016). As revealed in this study, an ISR of 1 induced a mild substrate overloading, whereas the chosen ISR of 0.5 led to conditions of high substrate overloading.
Irrespective of the applied biochar dosage, the addition of biochar produced from oak wood did not have boosting effects on methane yield under normal loading (ISR 2) and mild overloading (ISR 1) conditions. By contrast, Fagbohungbe et al. (2016) and Deng et al. (2021) reported a slight increase in methane yield by up to 12% and 5%, respectively, under normal loading, which may have resulted from difficult degradable substrates containing an inhibitor and a high content of lignocellulosic material. In this study, dog food was used as a food waste substitute, which is easily degradable due to its high carbohydrate content. Additionally, the C/N ratio of the used food waste substitute was 11.5, which was below the optimal range of 20–35 for AD in the batch mode (TG et al., 2022). However, the potential inhibition of methane production owing to the insufficient C/N ratio is considered negligible. This is because the average methane yield of approximately 430 NmL/(gVS,added) at all the ISRs tested in the present study is close to the actual achievable BMP of 454 ± 15 NmL/(gVS,added). In addition, other researchers have also used a very similar dog food as the food waste substitute in batch tests and did not observe any inhibitions in methane yield (Koch et al., 2017). Moreover, an enhancement in methane yield under conditions of a mild substrate overloading (ISR 1) was also observed in previous studies by up to 22% (Chiappero et al., 2021) and 37% (Ovi et al., 2022), in which also easily degradable substrate sewage sludge and food waste containing high content of carbohydrate was used. However, both the results had shown a removal of chemical oxygen demand (COD) of approximately 60% in control treatments, which indicates an inhibition under this mild substrate overloading. Because the removal of COD of the substrate in the AD processes suggests anaerobic biodegradability of the substrate (Guwy, 2004), which can theoretically be achieved to 92% (VDI, 2016), less than 70% should be considered to be the presence of inhibitions in the AD processes (Raposo et al., 2011). Thus, their results suggest that high biochar dosages ranging from 5 to 10 gTS/L alleviate the inhibition under mild substrate overloading equivalent to ISR 1 in the present study. However, the comparatively lower maximum biochar dosage of 2 gTS/L (25%) used in our study at ISR 1 may be insufficient to boost methane production. On the contrary, the biodegradability based on the substrate removal of our results at ISR 1 was determined as 88% (Figure 4B), which implies that no inhibition could occur during the AD experiments. Hence, the biochar did not affect the BMP and increased the methane yield only when the BMP could not be achieved under some inhibitions.
The amount of substrate was increased to an ISR of 0.5 and generated a high substrate overloading condition, and as our results have shown the methane production process was inhibited. The inhibition resulted from an imbalance of metabolic intermediates and an accumulation of volatile fatty acids (VFAs), i.e., acid stress (VDI, 2016), because the used substrate food waste containing a high content of carbohydrate allows rapid hydrolysis and acidogenesis procedures for converting substrates to VFAs (Pavlostathis and Giraldo-Gomez, 1991; Xu et al., 2018). Under this condition, an enhancement of methane yield was observed, suggesting that biochar could mitigate acid stress. Pan et al. (2022) and Zhang et al. (2019) reported that using biochar at an ISR of 0.3 with easily degradable substrates such as cow manure and sewage sludge, resulted in a considerable enhancement in methane yield by up to 80%. However, the methane yield from the control treatments of these two studies was strongly inhibited and much lower than their typical BMP values. Pan et al. (2022) reported a methane yield of 80 NmL/(gVS,added) without biochar addition, but for cow manure, the typical BMP was 210 NmL/(gVS,added) (Amon, 2015). Zhang et al. (2019) reported a methane yield of sewage sludge of 120 NmL/(gVS,added) without biochar addition, which is usually 300 NmL/(gVS,added) (DWA-A198, 2003). This is consistent with the results at ISR 1 from the literature (Chiappero et al., 2021; Ovi et al., 2022) that only under inhibition conditions, the methane yield increases. The more severe the inhibition is, the more enhanced is the methane yield or, in another words, the more the recovery of methane production. However, the biochar dosages used in these two earlier studies were either higher than (8 gTS/L, Zhang et al., 2019) or similar to (4 gTS/L, Pan et al., 2022) the 25% biochar added under ISR 0.5 condition, where an enhanced methane yield was observed in the present study. Hence, biochar can mitigate acid stress leading to the recovery of methane production but not increase the BMP, and an excessive addition was not only economically unfavourable but also reported to inhibit the AD processes (Zhang et al., 2019; Ovi et al., 2022).
Considering our results that the biochar dosage of 25% enhanced the removal of VS of a substrate, the increase in methane yield with 25% biochar addition at ISR 0.5 should have resulted from the better degradation of the substrate, as well as better conversion to methane. This can be explained by the results of previous studies that biochar stimulates both the production and degradation of metabolic intermediates, i.e., VFAs (Luo et al., 2015; Kaur et al., 2020; Deng et al., 2021; Johnravindar et al., 2021), which has been proved through their reported VFAs profiles. In our study, we found the curves of specific methane production rates at ISR 0.5 showed multiple peaks or bottoms, which indicates inhibition at the bottom of the curves, and it could recover (peaks). This is similar to previous studies, where the authors also observed multiple peaks and bottoms in the specific methane production rates’ curves (Kaur et al., 2020; Wang et al., 2020; Johnravindar et al., 2021), and the bottoms were correlated with the VFA peaks shown in their VFA profiles. These provide evidence that VFAs inhibit methane production. Our results showed that with biochar addition, the recovery of specific methane production rates occurred prior to control treatments. This is consistent with previous studies, in which biochar also stimulated a rapid recovery of methane production when compared to the control (Luo et al., 2015; Kaur et al., 2020; Johnravindar et al., 2021). This could be attributed to the alkaline properties of the used biochar types, all with a pH value above 8.5 (Luo et al., 2015; Kaur et al., 2020; Johnravindar et al., 2021), which can mitigate acid stress resulting from VFA accumulations, and may consequently provide a neutral environment which favours methanogens (Williams and Crawford, 1984; Garcia et al., 2000; Megonigal et al., 2004). The oak wood biochar used in the present study also had a high pH of 10. In addition, we determined that the oak wood biochar had a mild buffer capacity with CaCO3 equivalent to 6%, whereby the buffer capacity may have resulted from alkali and alkaline earth metals (i.e., K, Ca, and Mg) in the biochar (Shen et al., 2015; Wang et al., 2017). The K, Ca, and Mg contents of our oak wood biochar (Table 2) are very similar to the biochar used by Wang et al. (2017), who investigated the acid-buffering capacity of biochar in AD with contents of K, Ca, and Mg at 2.53 g/kg, 15.8 g/kg, and 0.44 g/kg, respectively, and also an alkaline pH of 9. The alkaline properties in biochar may lead them to conclude that biochar can mitigate acid stress through the provision of an adequate acid-buffer capacity, and is hence of benefit to methane production.
Nevertheless, the methane yield demonstrated a reverse trend against VS removal regarding ISRs, which implies a high methane production linked to a low VS removal. The unexpected correlation could have resulted from the incubation time that all the treatments ended together after 26 days. However, the methane production at ISR 2 and ISR 1 was already terminated by day 12 and day 18, respectively. The long incubation results in further degradation of the digestate which may explain the higher VS removal of ISR 2 and ISR 1 than of ISR 0.5. In addition, the lower methane yield could have been caused by the resolution of the gas detection device, which might have failed to detect and record the lower residual gas potential from the ISR 2 and ISR 1. This finding suggests that in future work, the bottles should be terminated individually following the 0.5% criteria of VDI 4630 (2016) to ensure an accurate substrate removal rate.
Due to the experimental configuration, biogas was assumed to only comprise CO2 and CH4. The CO2 content was almost identical in all treatments (Supplementary Figure S1). This indicates that the CH4 content was unaffected by biochar addition. However, Shen et al. (2015) observed that biochar can remove CO2 significantly and explained this by the high ash content in their biochar, which was 45.2% with high concentrations of K, Ca, and Mg (14.2%, 3.9%, and 4.2% of the ash content, respectively). By contrast, the oak wood biochar that we used contained very low ash content of only 3.4% and a K, Ca, and Mg content of 3.8 mg/g, 10.4 mg/g, and 0.4 mg/g, respectively, which shows a different percentage of alkaline metals containing ash than was observed by Shen et al. (2015). Thus, the divergent outcomes of the experiments are attributed to the use of biochar types with varying characteristics.
4.2 Effect of biochar on methane production rate and stability
We demonstrated a rapid production of methane at ISR 0.5 with 25% biochar, while at other ISRs, no significant improvement of the production rate was observed. This could be attributed to the alkaline pH of biochar (Luo et al., 2015; Wang et al., 2017), which can mitigate the acid stress, since the improvement of the production rate was only observed under high substrate overloading ISR 0.5. It was also reported that the lag-phase of the AD process can be shortened with biochar addition (Fagbohungbe et al., 2016; Jang et al., 2018), and consequently, the incubation time decreased. However, an increase in the maximum methane production rate because of biochar addition (Luo et al., 2015; Shen et al., 2015) can also contribute to rapid methane production. Luo et al. (2015) conducted the experiments under high substrate overloading (ISR 1/2, 1/4, 1/6, and 1/8) with easily degradable glucose as the substrate and a biochar dosage of 10 gTS/L. It was observed that under extreme high overloading of ISR 1/4, 1/6, and 1/8, the lag-phase was shortened and the maximum methane production rate was increased. While Jang et al. (2018) carried out experiments at ISR 1, as well as with 10 gTS/L biochar and easily degradable dairy manure as the substrate, the effects were tested at psychrophilic, mesophilic, and thermophilic temperatures and all treatments showed a shortened lag-phase. Fagbohungbe et al. (2016) used difficult degradable citrus peel as the substrate containing an inhibitor at ISR 3 and reported a shortened lag-phase that decreased with increasing biochar dosage from 6 to 35 gTS/L. All the used biochar types had the same alkaline characteristics, with high pH or high ash content and rich in alkali and alkaline earth metals. In the present study, no increase in the maximum methane production rate and no lag-phase were observed, presumably because of the use of easily degradable substrate. Nevertheless, the average methane production rate at ISR 0.5 with 25% biochar was increased considerably. Regardless of ISRs and substrate types, the used biochar amount in the previous studies were much higher than in our study, with only 4 gTS/L at ISR 0.5 and with 25% biochar. It was also reported that excessive biochar addition resulted in inhibitions of the AD process (Zhang et al., 2019; Ovi et al., 2022). Thus, the average methane production rate can be accelerated by adequate biochar addition to shorten the lag-phase or increase the maximum methane production rate due to the alkaline characteristics of biochar.
Nevertheless, the applied oak wood biochar in this study had O/C and H/C ratios of 0.057 and 0.012, respectively, which suggests a high degree of carbonization and hydrophobicity (Tan et al., 2015; Masebinu et al., 2019; Chiappero et al., 2020). In this context, biochar with lower H/C and O/C ratios exhibits a more graphitic structure. Luo et al. (2015) and Shen et al. (2015) used similar biochars as in our study, with also very low O/C and H/C ratios (Supplementary Table S1). Shen et al. (2015) reported increased process stability, but under thermophilic condition, whereas Luo et al. (2015) reported an enhancement in both degradation and production of intermediate acids, which could also contribute to the process stability. These could be explained by stimulating methanogenesis (Lü et al., 2016; Zhang et al., 2019; Deng et al., 2021) due to the graphitic structure with reduced resistance to the electron transfer process (Lovley, 2017; Johnravindar et al., 2021). By contrast, increasing process stability regarding the degradation of intermediate acids was investigated by Johnravindar et al. (2021), but the O/C and H/C ratios of their used biochars were an order of magnitude greater than that presented in our study. Despite this, other studies have shown that both granular and monolithic biochars are electrically conductive materials, and thus act as electron mediators or shuttles that facilitate direct interspecies electron transfer (DIET) (Gabhi et al., 2017; Gabhi et al., 2020; Gahlot et al., 2020). Several studies, which include reviews, have attributed increased AD and methane production after biochar addition to the DIET mechanism (Gahlot et al., 2020; Wang et al., 2021; Zhang et al., 2023). For example, one comprehensive review investigating the role of conductive material in AD showed that DIET was a major mechanism accounting for improved AD processes when using biochar (Gahlot et al., 2020). Although the DIET mechanism was not directly investigated in the present study, as discussed earlier, the H/C and O/C ratios of 0.012 and 0.057, respectively, that were measured for the biochar used in the present study point towards the possibility for such mechanisms to contribute and enhance AD. Other studies report that the H/C ratio below 0.35 and O/C ratio below 0.09 are indicative of a more graphitic structure of biochar (Sun et al., 2017). Furthermore, powdered or granular biochars with electrical conductivity for electrons ranging from 2.11 μS/cm to 4.41 μS/cm were reported to promote DIET (Chen et al., 2014). However, the relative contributions of various mechanisms (i.e., pH buffering versus DIET) to the enhancement of the AD processes require further investigation. Additionally, some researchers have suggested that biochar facilitates methanogenesis via selective enrichment of microbes due to the specific surface area (SSA) of biochar (Luo et al., 2015; Zhang et al., 2019). However, one other researcher suggested no link between the SSA of biochar and enhanced AD processes (Pan et al., 2022). Thus, well-designed long-term experiments are required to examine the interactions of biochar and microbial growth. However, according to Luo et al. (2015) and Johnravindar et al. (2021), the biochars that they used had an alkaline pH with 9 on average, and their experiments were conducted under very high substrate overloading conditions (up to ISR 0.1), with a high biochar dosage of 10 gTS/L added, which are consistent with our study in that only under high substrate overloading and high biochar dosage does the process stability increase. This may imply that the alkaline properties of biochar can mitigate acid stress resulting from the high substrate overloading, and thus can stabilize AD processes in a favourable environment of methanogens. However, the increased process stability cannot be explained completely only based on the evidence of molar ratios and alkaline pH values of biochar. Future work should consider conducting long-term trials, checking pH profiles during AD processes and providing microbiological evidence to explain the improved process stability. Additionally, further research should also focus on 1) the effects of conductive biochar materials in AD to understand the role of various mechanisms better, such as in DIET and 2) systematic optimization of the key factors controlling the AD process in order to determine optimal values and improve the transferability and practical application of biochar in AD.
4.3 Critical evaluation towards achieving comparable effects using biochar under practical realistic conditions
The batch approach utilised in this study allows some assumptions regarding the short-term effect of biochar on AD processes. In particular, the process conditions, such as the inoculum-to-substrate ratio (ISR) and the used biochar dosage, play an important role in AD processes. Our results showed that the beneficial effects of biochar, for example, higher methane yield, higher methane production rate, and process stability, can only be observed under high substrate overloading (ISR 0.5) with high biochar dosage (25%). Comparisons with other studies is however difficult because of the variety of used substrates, such as easily or difficultly degradable substrate, in addition to different biochar dosages or types and the various substrate loadings in the AD systems. Nevertheless, some studies have used very high ISRs with easily degradable substrate that leads to strong acid stress (Luo et al., 2015; Wang et al., 2017; Zhang et al., 2019), some used normal ISRs but with substrate containing inhibitors or difficult anaerobic degradable lignocellulosic materials (Fagbohungbe et al., 2016; Deng et al., 2021). Thus, both the previous studies and the current study demonstrate that biochar has effects only on AD processes when an inhibition condition occurs. To ensure detectable effects of biochar, very high dosages have been used in previous studies. Most of them have ranged from 5 gTS/L to 35 gTS/L or a ratio from 50% to 300% based on substrate VS (Supplementary Table S1). It should be noted that biochar is used only as an additive rather than as a competitor of the substrate to occupy the working volume of the digester. The low biochar dosage with 10% however did not affect the AD processes under all our tested ISRs. The high biochar dosage of 25% had positive effects on AD, but only under inhibitions (ISR 0.5). This ratio with 25% is already quite lower than it is in the literature but is still too high for some practical applications, for example, a large-scale agricultural biogas plant. The high amount of biochar might occupy the working volume of the digester, thereby reducing the amount of substrate in the reactor. As discussed earlier, the improved methane production rate could enhance the substrate throughput and partially compensate for the lost operation capacity. However, it is unlikely that such a scenario will be economically feasible under practical conditions. Despite this, if we include the energy use of syngas generated during pyrolysis, ranging usually from 1,000 MJ to 30,000 MJ per tonne of produced biochar (Sanna et al., 2011; Crombie and Mašek, 2015; Joseph et al., 2020; Heinrich et al., 2023b), it not only is sufficient to cover the self-energy demand but also provides surplus energy to decouple (Joseph et al., 2020; Heinrich et al., 2023b). Furthermore, we can also consider the CO2 sequestration potential of biochar, for example, our used oak wood biochar had a low O/C and H/C ratio indicating high carbon stability in the soil (Spokas, 2010), thus resulting in a high potential of carbon storage. Therefore, use of biochar in AD remains attractive, and our future work will focus on the life cycle assessment of this attractive approach. In summary, effects can only be observed using high biochar dosages and under inhibition conditions. When using a low biochar dosage or under normal conditions which are more common in practical applications, biochar does not affect the AD processes; at least in short-term batch trials, we cannot observe any comparable effects.
However, the individual characteristics of each biochar can make the comparison of effects and generalization of application conditions of using biochar in AD challenging. The biochar used in previous studies and the current study varies, and show different ash contents and ash compositions (K, Ca, and Mg), as well as different molar ratios, affecting the characteristics of biochar and further affecting the effects of biochar in AD. These characteristics are dependent on pyrolysis temperatures and feedstock (Lehmann and Joseph, 2009), which will be the focus of our future work, for example, to produce biochar at different pyrolysis temperatures and in using various feedstock, especially residue biomass. Nevertheless, the batch test is a powerful tool to determine the BMP of a substrate or mixture, substrate anaerobic biodegradability, and short-term effect of an inhibitor and qualitatively describe the kinetic of an AD process (Koch et al., 2020). It is widely used but limited in transferability. For example, typical digester design parameters, such as hydraulic retention time and organic loading rate, can only be determined through continuous experiments (VDI, 2016). Furthermore, beneficial long-term or synergistic effects of substrate–biochar mixtures cannot be determined in the batch mode either (Koch et al., 2020). In particular, due to the short incubation time and the feeding mode of batch tests, the adaption of beneficial microorganisms to biochar cannot be assessed. Because a steady state cannot be reached, we did not include a microbiological analysis in the current study. Future work that considers the long-term effects and includes microbiological assessments under a practical realistic condition with respect to both biochar dosages and normal substrate loadings is required. Despite the limitations, the use of biochar in AD processes has a great potential of sequestrating carbon when we use the biochar-enriched digestate for agricultural applications as soil amendments. However, there are some legal restrictions for this application concerning environmental impacts due to greenhouse emission, water contamination, and trace metals.
5 Conclusion
Contrary to previous findings, our results have shown that under practical realistic substrate loadings, the addition of biochar does not affect AD processes even at various biochar dosages. This is attributed to different substrates and biochar types, as well as different experimental conditions. A high dosage of biochar addition to AD processes can however mitigate acid stress caused by high substrate overloading. Under such a condition, biochar-assisted AD processes have further potentials to alter the methane production rate towards an improvement of the treatment capacity of the substrate, and consequently to enhance the cumulative methane production. Furthermore, biochar can stabilize the AD process under acid stress, which allows better resilience of the anaerobic digestion system against substrate overloading. However, we critically evaluated the effects of using various biochar dosages in AD processes under normal conditions and severe conditions and compared the effects under severe conditions with that found in the literature. To achieve comparable effects under severe conditions, we suggested that future work should investigate the effects in AD processes under practical realistic conditions and the long-term effects of using biochar in a practical applicable dosage.
Data availability statement
The raw data supporting the conclusions of this article will be made available by the authors, without undue reservation.
Author contributions
JH and KK contributed to the study conception. Design, material preparation, data collection, and analysis were performed by JH. The first draft of the manuscript was written by JH. KS commented on statistics, visualization, and previous revised versions of the manuscript. KK acquired funding and revised the manuscript. WG critically revised the manuscript and commented on the writing and interpretation of the data. MW acquired funding and commented on the manuscript. All authors contributed to the article and approved the submitted version.
Funding
This study was partly funded by Interreg NWE Three C project (grant number: NWE1010) and Interreg NWE RE-DIRECT project (grant number: NWE294).
Acknowledgments
WG thanks the following 1) Alexander von Humboldt Stiftung/Foundation for funding the Georg Forster Fellowship, and 2) the Universität Kassel and the Leibniz-Institut für Agrartechnik und Bioökonomie (ATB), Potsdam for co-hosting the research fellowship. The authors also wish to thank the support by the Open Access Publication Fund of the Universität Kassel.
Conflict of interest
The authors declare that the research was conducted in the absence of any commercial or financial relationships that could be construed as a potential conflict of interest.
Publisher’s note
All claims expressed in this article are solely those of the authors and do not necessarily represent those of their affiliated organizations, or those of the publisher, editors, and reviewers. Any product that may be evaluated in this article, or claim that may be made by its manufacturer, is not guaranteed or endorsed by the publisher.
Supplementary material
The Supplementary Material for this article can be found online at: https://www.frontiersin.org/articles/10.3389/fenrg.2023.1205818/full#supplementary-material
References
Angelidaki, I., Alves, M., Bolzonella, D., Borzacconi, L., Campos, J. L., Guwy, A. J., et al. (2009). Defining the biomethane potential (BMP) of solid organic wastes and energy crops: A proposed protocol for batch assays. Water Sci. Technol. 59, 927–934. doi:10.2166/wst.2009.040
Asirifi, I., Kaetzl, K., Werner, S., Heinze, S., Abagale, F. K., Wichern, M., et al. (2023). Biochar for wastewater treatment and soil improvement in irrigated urban agriculture: Single and combined effects on crop yields and soil fertility. J. Soil Sci. Plant Nutr. 23, 1408–1420. doi:10.1007/s42729-023-01132-7
Asirifi, I., Kaetzl, K., Werner, S., Saba, C. K. S., Abagale, F. K., Amoah, P., et al. (2021). Pathogen and heavy metal contamination in urban agroecosystems of northern Ghana: Influence of biochar application and wastewater irrigation. J. Environ. Qual. 50, 1097–1109. doi:10.1002/jeq2.20260
Bachmann, M., Völker, S., Kleinekorte, J., and Bardow, A. (2023). Syngas from what? Comparative life-cycle assessment for syngas production from biomass, CO 2, and steel mill off-gases. ACS Sustain. Chem. Eng. 11, 5356–5366. doi:10.1021/acssuschemeng.2c05390
Camps-Arbestain, M., Amonette, J. E., Singh, B., Wang, T., and Schmidt, H.-P. (2015). “A biochar classification system and associated test methods,” in Biochar for environmental management. Editors J. Lehmann, and S. Joseph (Oxfordshire: Routledge).
Chen, S., Rotaru, A.-E., Shrestha, P. M., Malvankar, N. S., Liu, F., Fan, W., et al. (2014). Promoting interspecies electron transfer with biochar. Sci. Rep. 4, 5019. doi:10.1038/srep05019
Chiappero, M., Cillerai, F., Berruti, F., Mašek, O., and Fiore, S. (2021). Addition of different biochars as Catalysts during the mesophilic anaerobic digestion of mixed wastewater sludge. Catalysts 11, 1094. doi:10.3390/catal11091094
Chiappero, M., Norouzi, O., Hu, M., Demichelis, F., Berruti, F., Di Maria, F., et al. (2020). Review of biochar role as additive in anaerobic digestion processes. Renew. Sustain. Energy Rev. 131, 110037. doi:10.1016/j.rser.2020.110037
Crombie, K., and Mašek, O. (2015). Pyrolysis biochar systems, balance between bioenergy and carbon sequestration. GCB Bioenergy 7, 349–361. doi:10.1111/gcbb.12137
Dai, B., Xu, J., He, Y., Xiong, P., Wang, X., Deng, Y., et al. (2015). Acid inhibition during anaerobic digestion of biodegradable kitchen waste. J. Renew. Sustain. Energy 7, 023118. doi:10.1063/1.4918281
Dang, Y., Sun, D., Woodard, T. L., Wang, L.-Y., Nevin, K. P., and Holmes, D. E. (2017). Stimulation of the anaerobic digestion of the dry organic fraction of municipal solid waste (OFMSW) with carbon-based conductive materials. Bioresour. Technol. 238, 30–38. doi:10.1016/j.biortech.2017.04.021
Deng, C., Lin, R., Kang, X., Wu, B., Wall, D. M., and Murphy, J. D. (2021). What physicochemical properties of biochar facilitate interspecies electron transfer in anaerobic digestion: A case study of digestion of whiskey by-products. Fuel 306, 121736. doi:10.1016/j.fuel.2021.121736
DEV (2020). Deutsche Einheitsverfahren zur Wasser-, Abwasser-und Schlamm-Untersuchung (German Standard Methods for Examination of Water, Wastewater and Sludge). Weinheim, Germany: VCH.
DWA-A198 (2003). Arbeitsblatt ATV-DVWK-A 198 - vereinheitlichung und Herleitung von Bemessungswerten für Abwasseranlagen. Hennef: Ges. zur Förderung der Abwassertechnik.
EBC (2023). European biochar certificate - guidelines for a sustainable production of biochar. Available at: https://www.european-biochar.org/en/ct/2-EBC-guidelines-documents (Accessed May 17, 2023).
Fagbohungbe, M. O., Herbert, B. M. J., Hurst, L., Li, H., Usmani, S. Q., and Semple, K. T. (2016). Impact of biochar on the anaerobic digestion of citrus peel waste. Bioresour. Technol. 216, 142–149. doi:10.1016/j.biortech.2016.04.106
Fehrenbach, H., Köppen, S., Kauertz, B., Detzel, A., Wellenreuther, F., Breitmayer, E., et al. (2017). BIOMASS CASCADES Increasing resource efficiency by cascading use of biomass — from theory to practice. Dessau-Roßlau, Germany: Umweltbundesamt.
Filer, J., Ding, H. H., and Chang, S. (2019). Biochemical methane potential (BMP) assay method for anaerobic digestion research. Water 11, 921. doi:10.3390/w11050921
Fox, J., and Weisberg, S. (2019). An R companion to applied regression. Thousand Oaks, Canada: Sage.
Gabhi, R., Basile, L., Kirk, D. W., Giorcelli, M., Tagliaferro, A., and Jia, C. Q. (2020). Electrical conductivity of wood biochar monoliths and its dependence on pyrolysis temperature. Biochar 2, 369–378. doi:10.1007/s42773-020-00056-0
Gabhi, R. S., Kirk, D. W., and Jia, C. Q. (2017). Preliminary investigation of electrical conductivity of monolithic biochar. Carbon 116, 435–442. doi:10.1016/j.carbon.2017.01.069
Gahlot, P., Ahmed, B., Tiwari, S. B., Aryal, N., Khursheed, A., Kazmi, A. A., et al. (2020). Conductive material engineered direct interspecies electron transfer (DIET) in anaerobic digestion: Mechanism and application. Environ. Technol. Innovation 20, 101056. doi:10.1016/j.eti.2020.101056
Garcia, J. L., Patel, B. K., and Ollivier, B. (2000). Taxonomic, phylogenetic, and ecological diversity of methanogenic Archaea. Anaerobe 6, 205–226. doi:10.1006/anae.2000.0345
Guwy, A. J. (2004). Equipment used for testing anaerobic biodegradability and activity. Rev. Environ. Sci. Bio/Technol. 3, 131–139. doi:10.1007/s11157-004-1290-0
Haubold-Rosar, M., Heinkele, T., Rademacher, A., Kern, J., Dicke, C., Funke, A., et al. (2016). Chancen und Risiken des Einsatzes von Biokohle und anderer „veränderter“ Biomasse als Bodenhilfsstoffe oder für die C-Sequestrierung in Böden. Dessau-Roßlau, Germany: Umweltbundesamt.
Heinrich, T., Kaetzl, K., Libra, J. A., and Hoffmann, T. (2023a). Influence of thermochemical conversion Technologies on biochar characteristics from extensive grassland for safe soil application. Energies (Basel) 16, 1896. doi:10.3390/en16041896
Heinrich, T., Park, H., Orozco, R., Ding, Z., Álvarez-López, V., Mosquera-Losada, M. R., et al. (2023b). Biochar production from late-harvest grass – challenges and potential for farm-scale implementation. Sustain. Prod. Consum. 37, 256–267. doi:10.1016/j.spc.2023.02.019
Hollender, J., Zimmermann, S. G., Koepke, S., Krauss, M., McArdell, C. S., Ort, C., et al. (2009). Elimination of organic micropollutants in a municipal wastewater treatment plant upgraded with a full-scale post-ozonation followed by sand filtration. Environ. Sci. Technol. 43, 7862–7869. doi:10.1021/es9014629
Holliger, C., Alves, M., Andrade, D., Angelidaki, I., Astals, S., Baier, U., et al. (2016). Towards a standardization of biomethane potential tests. Water Sci. Technol. 74, 2515–2522. doi:10.2166/wst.2016.336
Jang, H. M., Choi, Y.-K., and Kan, E. (2018). Effects of dairy manure-derived biochar on psychrophilic, mesophilic and thermophilic anaerobic digestions of dairy manure. Bioresour. Technol. 250, 927–931. doi:10.1016/j.biortech.2017.11.074
Jensen, P. D., Ge, H., and Batstone, D. J. (2011). Assessing the role of biochemical methane potential tests in determining anaerobic degradability rate and extent. Water Sci. Technol. 64, 880–886. doi:10.2166/wst.2011.662
Johnravindar, D., Wong, J. W. C., Chakraborty, D., Bodedla, G., and Kaur, G. (2021). Food waste and sewage sludge co-digestion amended with different biochars: VFA kinetics, methane yield and digestate quality assessment. J. Environ. Manage. 290, 112457. doi:10.1016/j.jenvman.2021.112457
Joseph, B., Kaetzl, K., Hensgen, F., Schäfer, B., and Wachendorf, M. (2020). Sustainability assessment of activated carbon from residual biomass used for micropollutant removal at a full-scale wastewater treatment plant. Environ. Res. Lett. 15, 064023. doi:10.1088/1748-9326/ab8330
Kaetzl, K., Lübken, M., Uzun, G., Gehring, T., Nettmann, E., Stenchly, K., et al. (2019). On-farm wastewater treatment using biochar from local agroresidues reduces pathogens from irrigation water for safer food production in developing countries. Sci. Total Environ. 682, 601–610. doi:10.1016/j.scitotenv.2019.05.142
Kaur, G., Johnravindar, D., and Wong, J. W. C. (2020). Enhanced volatile fatty acid degradation and methane production efficiency by biochar addition in food waste-sludge co-digestion: A step towards increased organic loading efficiency in co-digestion. Bioresour. Technol. 308, 123250. doi:10.1016/j.biortech.2020.123250
Koch, K., Bajón Fernández, Y., and Drewes, J. E. (2015). Influence of headspace flushing on methane production in Biochemical Methane Potential (BMP) tests. Bioresour. Technol. 186, 173–178. doi:10.1016/j.biortech.2015.03.071
Koch, K., and Drewes, J. E. (2014). Alternative approach to estimate the hydrolysis rate constant of particulate material from batch data. Appl. Energy 120, 11–15. doi:10.1016/j.apenergy.2014.01.050
Koch, K., Hafner, S. D., Weinrich, S., Astals, S., and Holliger, C. (2020). Power and limitations of biochemical methane potential (BMP) tests. Front. Energy Res. 8, 63. doi:10.3389/fenrg.2020.00063
Koch, K., Lippert, T., and Drewes, J. E. (2017). The role of inoculum's origin on the methane yield of different substrates in biochemical methane potential (BMP) tests. Bioresour. Technol. 243, 457–463. doi:10.1016/j.biortech.2017.06.142
Lehmann, J., and Joseph, S. (2009). Biochar for environmental management Science and technology. London, UK: Earthscan.
Liu, F., Rotaru, A.-E., Shrestha, P. M., Malvankar, N. S., Nevin, K. P., and Lovley, D. R. (2012). Promoting direct interspecies electron transfer with activated carbon. Energy Environ. Sci. 5, 8982. doi:10.1039/c2ee22459c
Lovley, D. R. (2017). Syntrophy goes electric: Direct interspecies electron transfer. Annu. Rev. Microbiol. 71, 643–664. doi:10.1146/annurev-micro-030117-020420
Lü, F., Luo, C., Shao, L., and He, P. (2016). Biochar alleviates combined stress of ammonium and acids by firstly enriching Methanosaeta and then Methanosarcina. Water Res. 90, 34–43. doi:10.1016/j.watres.2015.12.029
Luo, C., Lü, F., Shao, L., and He, P. (2015). Application of eco-compatible biochar in anaerobic digestion to relieve acid stress and promote the selective colonization of functional microbes. Water Res. 68, 710–718. doi:10.1016/j.watres.2014.10.052
Mao, C., Feng, Y., Wang, X., and Ren, G. (2015). Review on research achievements of biogas from anaerobic digestion. Renew. Sustain. Energy Rev. 45, 540–555. doi:10.1016/j.rser.2015.02.032
Masebinu, S. O., Akinlabi, E. T., Muzenda, E., and Aboyade, A. O. (2019). A review of biochar properties and their roles in mitigating challenges with anaerobic digestion. Renew. Sustain. Energy Rev. 103, 291–307. doi:10.1016/j.rser.2018.12.048
Megonigal, J. P., Hines, M. E., and Visscher, P. T. (2004). Anaerobic metabolism: Linkages to trace gases and aerobic processes. Oxford: Elsevier.
Mumme, J., Srocke, F., Heeg, K., and Werner, M. (2014). Use of biochars in anaerobic digestion. Bioresour. Technol. 164, 189–197. doi:10.1016/j.biortech.2014.05.008
Nakasaki, K., Nagasaki, K., and Ariga, O. (2004). Degradation of fats during thermophilic composting of organic waste. Waste Manag. Res. 22, 276–282. doi:10.1177/0734242X04045430
Nematian, M., Keske, C., and Ng'ombe, J. N. (2021). A techno-economic analysis of biochar production and the bioeconomy for orchard biomass. Waste Manag. 135, 467–477. doi:10.1016/j.wasman.2021.09.014
Nurk, L., Bühle, L., and Wachendorf, M. (2016). Degradation of fibre and non-fibre fractions during anaerobic digestion in silages of maize, sunflower and sorghum-sudangrass of different maturities. Bioenerg. Res. 9, 720–730. doi:10.1007/s12155-016-9717-3
Olsson, O., Bruce, L., Roos, A., Hektor, B., Guisson, R., Lamers, P., et al. (2016). Cascading of woody biomass: Definitions, policies and effects on international trade. Paris: IEA Bioenergy.
Ovi, D., Chang, S. W., Wong, J. W. C., Johnravindar, D., Varjani, S., Hoon Jeung, J., et al. (2022). Effect of rice husk and palm tree-based biochar addition on the anaerobic digestion of food waste/sludge. Fuel 315, 123188. doi:10.1016/j.fuel.2022.123188
Pan, J., Sun, J., Ao, N., Xie, Y., Zhang, A., Chen, Z., et al. (2022). Factors influencing biochar-strengthened anaerobic digestion of cow manure. Bioenergy Res. doi:10.1007/s12155-022-10396-3
Pavlostathis, S. G., and Giraldo-Gomez, E. (1991). Kinetics of anaerobic treatment: A critical review. Crit. Rev. Environ. Control 21, 411–490. doi:10.1080/10643389109388424
R Core Team (2021). R: A language and environment for statistical computing. Vienna, Austria: R Foundation for Statistical Computing.
Rajkovich, S., Enders, A., Hanley, K., Hyland, C., Zimmerman, A. R., and Lehmann, J. (2012). Corn growth and nitrogen nutrition after additions of biochars with varying properties to a temperate soil. Biol. Fertil. Soils 48, 271–284. doi:10.1007/s00374-011-0624-7
Raposo, F., Borja, R., Martín, M. A., Martín, A., La Rubia, M. A., and Rincón, B. (2009). Influence of inoculum–substrate ratio on the anaerobic digestion of sunflower oil cake in batch mode: Process stability and kinetic evaluation. Chem. Eng. J. 149, 70–77. doi:10.1016/j.cej.2008.10.001
Raposo, F., Fernández-Cegrí, V., La Rubia, M. A., Borja, R., Béline, F., Cavinato, C., et al. (2011). Biochemical methane potential (BMP) of solid organic substrates: Evaluation of anaerobic biodegradability using data from an international interlaboratory study. J. Chem. Technol. Biotechnol. 86, 1088–1098. doi:10.1002/jctb.2622
Rosenwinkel, K. H., Kroiss, H., Dichtl, N., Seyfried, C. F., and Weiland, P. (2015). Anaerobtechnik: Abwasser-, Schlamm-und Reststoffbehandlung, Biogasgewinnung. Berlin, Germany: Springer Vieweg.
Sanna, A., Li, S., Linforth, R., Smart, K. A., and Andrésen, J. M. (2011). Bio-oil and bio-char from low temperature pyrolysis of spent grains using activated alumina. Bioresour. Technol. 102, 10695–10703. doi:10.1016/j.biortech.2011.08.092
Shen, Y., Linville, J. L., Urgun-Demirtas, M., Schoene, R. P., and Snyder, S. W. (2015). Producing pipeline-quality biomethane via anaerobic digestion of sludge amended with corn stover biochar with in-situ CO2 removal. Appl. Energy 158, 300–309. doi:10.1016/j.apenergy.2015.08.016
Singh, B., Camps-Arbestain, M., and Lehmann, J. (2017). Biochar: A guide to analytical methods. Clayton Victoria, Australia: CSIRO Publishing.
Singh, B., Singh, B. P., and Cowie, A. L. (2010). Characterisation and evaluation of biochars for their application as a soil amendment. Soil Res. 48, 516. doi:10.1071/SR10058
Spokas, K. A. (2010). Review of the stability of biochar in soils: Predictability of O:C molar ratios. Carbon Manag. 1, 289–303. doi:10.4155/cmt.10.32
Strömberg, S., Nistor, M., and Liu, J. (2014). Towards eliminating systematic errors caused by the experimental conditions in Biochemical Methane Potential (BMP) tests. Waste Manag. 34, 1939–1948. doi:10.1016/j.wasman.2014.07.018
Sun, T., Levin, B. D. A., Guzman, J. J. L., Enders, A., Muller, D. A., Angenent, L. T., et al. (2017). Rapid electron transfer by the carbon matrix in natural pyrogenic carbon. Nat. Commun. 8, 14873. doi:10.1038/ncomms14873
Tan, X., Liu, Y., Zeng, G., Wang, X., Hu, X., Gu, Y., et al. (2015). Application of biochar for the removal of pollutants from aqueous solutions. Chemosphere 125, 70–85. doi:10.1016/j.chemosphere.2014.12.058
Tg, I., Haq, I., and Kalamdhad, A. S. (2022). “14 - factors affecting anaerobic digestion for biogas production: A review,” in Advanced organic waste management: Sustainable practices and approaches. Editors C. Hussain, and S. Hait (Elsevier), 223–233.
Thiffault, E., Gianvenuti, A., Zuzhang, X., and Walter, S. (2023). The role of wood residues in the transition to sustainable bioenergy: Analysis of good practices and recommendations for the deployment of wood residues for energy. Rome, Italy: FAO.
van Soest, P. J., and Wine, R. H. (1967). Use of detergents in the analysis of fibrous feeds. IV. Determination of plant cell-wall constituents. J. Assoc. Off. Anal. Chem. 50, 50–55. doi:10.1093/jaoac/50.1.50
VDI (2016). Vdi 4630: 2016-11 vergärung organischer stoffe - substratcharakterisierung, probenahme, stoffdatenerhebung, gärversuche (fermentation of organic materials characterisation of the substrate, sampling, collection of material data, fermentation tests). Berlin, Germany: Beuth Verlag GmbH.
Wang, D., Ai, J., Shen, F., Yang, G., Zhang, Y., Deng, S., et al. (2017). Improving anaerobic digestion of easy-acidification substrates by promoting buffering capacity using biochar derived from vermicompost. Bioresour. Technol. 227, 286–296. doi:10.1016/j.biortech.2016.12.060
Wang, J., Zhao, Z., and Zhang, Y. (2021). Enhancing anaerobic digestion of kitchen wastes with biochar: Link between different properties and critical mechanisms of promoting interspecies electron transfer. Renew. Energy 167, 791–799. doi:10.1016/j.renene.2020.11.153
Wang, Z., Jiang, Y., Wang, S., Zhang, Y., Hu, Y., Hu, Z.-h., et al. (2020). Impact of total solids content on anaerobic co-digestion of pig manure and food waste: Insights into shifting of the methanogenic pathway. Waste Manag. 114, 96–106. doi:10.1016/j.wasman.2020.06.048
Williams, R. T., and Crawford, R. L. (1984). Methane production in Minnesota peatlands. Appl. Environ. Microbiol. 47, 1266–1271. doi:10.1128/aem.47.6.1266-1271.1984
Xu, F., Li, Y., Ge, X., Yang, L., and Li, Y. (2018). Anaerobic digestion of food waste - challenges and opportunities. Bioresour. Technol. 247, 1047–1058. doi:10.1016/j.biortech.2017.09.020
Ye, M., Liu, J., Ma, C., Li, Y.-Y., Zou, L., Qian, G., et al. (2018). Improving the stability and efficiency of anaerobic digestion of food waste using additives: A critical review. J. Clean. Prod. 192, 316–326. doi:10.1016/j.jclepro.2018.04.244
Zhang, J., Liu, J., and Liu, R. (2015). Effects of pyrolysis temperature and heating time on biochar obtained from the pyrolysis of straw and lignosulfonate. Bioresour. Technol. 176, 288–291. doi:10.1016/j.biortech.2014.11.011
Zhang, K., Deng, Y., Liu, Z., Feng, Y., Hu, C., and Wang, Z. (2023). Biochar facilitated direct interspecies electron transfer in anaerobic digestion to alleviate antibiotics inhibition and enhance methanogenesis: A review. Int. J. Environ. Res. Public Health 20, 2296. doi:10.3390/ijerph20032296
Keywords: acid stress, biochar dosage, cascading use of biomass, food waste, methane production rate, substrate loading rates, wood residue
Citation: Hu J, Stenchly K, Gwenzi W, Wachendorf M and Kaetzl K (2023) Critical evaluation of biochar effects on methane production and process stability in anaerobic digestion. Front. Energy Res. 11:1205818. doi: 10.3389/fenrg.2023.1205818
Received: 14 April 2023; Accepted: 30 June 2023;
Published: 18 July 2023.
Edited by:
Vandit Vijay, Sardar Swaran Singh National Institute of Renewable Energy, IndiaReviewed by:
Nabin Aryal, University of South-Eastern Norway (USN), NorwaySameer Khan, Indian Institute of Technology Delhi, India
Copyright © 2023 Hu, Stenchly, Gwenzi, Wachendorf and Kaetzl. This is an open-access article distributed under the terms of the Creative Commons Attribution License (CC BY). The use, distribution or reproduction in other forums is permitted, provided the original author(s) and the copyright owner(s) are credited and that the original publication in this journal is cited, in accordance with accepted academic practice. No use, distribution or reproduction is permitted which does not comply with these terms.
*Correspondence: Korbinian Kaetzl, a2FldHpsQHVuaS1rYXNzZWwuZGU=