- 1Fraunhofer IEG—Fraunhofer Research Institution for Energy Infrastructures and Geothermal Systems, Bochum, Germany
- 2Institute of Sustainable Economic Development, University of Natural Resources and Life Sciences, Vienna, Austria
Hydrogen storage might be key to the success of the hydrogen economy, and hence the energy transition in Germany. One option for cost-effective storage of large quantities of hydrogen is the geological subsurface. However, previous experience with underground hydrogen storage is restricted to salt caverns, which are limited in size and space. In contrast, pore storage facilities in aquifers -and/or depleted hydrocarbon reservoirs- could play a vital role in meeting base load needs due to their wide availability and large storage capacity, but experiences are limited to past operations with hydrogen-bearing town gas. To overcome this barrier, here we investigate hydrogen storage in porous storage systems in a two-step process: 1) First, we investigate positive and cautionary indicators for safe operations of hydrogen storage in pore storage systems. 2) Second, we estimate hydrogen storage capacities of pore storage systems in (current and decommissioned) underground natural gas storage systems and saline aquifers. Our systematic review highlights that optimal storage conditions in terms of energy content and hydrogen quality are found in sandstone reservoirs in absence of carbonate and iron bearing accessory minerals at a depth of approx. 1,100 m and a temperature of at least 40°C. Porosity and permeability of the reservoir formation should be at least 20% and 5 × 10−13 m2 (∼500 mD), respectively. In addition, the pH of the brine should fall below 6 and the salinity should exceed 100 mg/L. Based on these estimates, the total hydrogen storage capacity in underground natural gas storages is estimated to be up to 8 billion cubic meters or (0.72 Mt at STP) corresponding to 29 TWh of energy equivalent of hydrogen. Saline aquifers may offer additional storage capacities of 81.6–691.8 Mt of hydrogen, which amounts to 3.2 to 27.3 PWh of energy equivalent of hydrogen, the majority of which is located in the North German basin. Pore storage systems could therefore become a crucial element of the future German hydrogen infrastructure, especially in regions with large industrial hydrogen (storage) demand and likely hydrogen imports via pipelines and ships.
1 Introduction
Global environmental changes and future energy needs are among the most fundamental challenges facing humanity in the near future. Green hydrogen produced by renewable energies has the capacity to overcome these obstacles by decarbonizing the industry, electricity generation, transport and heat. With its National Hydrogen Strategy (NWS), the German government has given a strong boost to the hydrogen economy in all sectors of the energy system (BMWi, 2020). But renewable energy sources needed for the production of green hydrogen are subjected to weather-related and seasonal fluctuations. Hence, measures are needed to stabilize and secure future energy supplies. Hydrogen storage could therefore be the decisive factor in the energy transition. Only then the divergent hydrogen demand and production will be balanced.
In addition to the storage of hydrogen in tanks or pipes, the geological subsurface also offers possibilities for the long-term storage of hydrogen. The hydrocarbon industry has been using the underground for decades in Germany and around the world to store large quantities of natural gas in the pore space of rocks or in salt caverns (e.g., American Gas Association, 1997; Sedlacek, 1999a; Sedlacek, 2002; Evans and Chadwick, 2009). More than 23 billion cubic meters of natural gas are stored underground in Germany (EEK Redaktion, 2021). This gives Germany the fourth-largest storage capacity in the world after the United States, Russia and the Ukraine (EEK Redaktion, 2015). About 36% (∼8.6 billion cubic meters) of the current technically usable working gas volume is stored in pore storage systems for seasonal base load coverage and 74% of the installed capacity are stored in salt caverns for peak load coverage with more efficient injection and withdrawal cycles (EEK Redaktion, 2021). Nevertheless, current research into underground hydrogen storage potential in Germany and Europe has mainly focused on salt caverns only (Iordache et al., 2014; Simón et al., 2015; Michalski et al., 2017; Tarkowski and Czapowski, 2018; Caglayan et al., 2020; Lankof, and Tarkowski, 2020; Williams et al., 2022). The total energy storage potential in salt caverns is estimated to be as high as 84.8 PWh in Europe and 35.7 PWh in Germany alone (Caglayan et al., 2020). It is therefore not surprising that first flagship projects for underground storage of hydrogen in salt caverns at the field scale have already been launched in Germany at the Bad Lauchstädt Energy Park (HYPOS), in Rüdersdorf (HyCavMobil) and in Epe (GetH2). However, suitable salt deposits are locally limited and only 9% of the total worldwide working gas capacity is attributed to salt caverns (Sedlacek, 1999b; Cedigaz, 2021). Pore storage facilities such as depleted gas fields and saline aquifers could therefore play an important role in the energy transition due to their wide spread and broad availability across Europe (e.g., Foh et al., 1979; Panfilov, 2016; Tarkowski, 2019; Heinemann et al., 2021a; Zivar et al., 2021; Hematpur et al., 2023).
Here, we investigate safety requirements and storage potentials in the light of future hydrogen needs. The aim is to formulate recommendations for future underground hydrogen storage in porous media. Our study starts with an overview of the past, present, and future of underground hydrogen storage (UHS) in Germany, discussing the experience with UHS and the prospects for hydrogen network structures. It highlights the progress that has been made and the challenges that need to be overcome for widespread deployment. In particular, there are critical factors that need to be considered in the design and operation of UHS facilities to ensure their safe and efficient operation. These critical factors or indicators, such as biotic and abiotic reactions, sealing capacity, cap rock integrity, cushion gas requirements, etc., are discussed before estimates of UHS storage capacity in Germany are made. Our estimates are made with respect to the discussion of technical hydrogen storage capacity in former and current underground gas storage facilities and the theoretical hydrogen storage capacity in saline aquifers. This section provides a quantitative assessment of the potential of UHS to support the energy transition in Germany. The fifth and final section discusses storage capacity and demand in Europe and assesses the costs and purity requirements of UHS. It builds on the findings of the previous sections and highlights the economic and technical challenges associated with UHS and the potential role of UHS in supporting the development of a sustainable hydrogen economy in Europe.
2 Past, present, and future of underground hydrogen storage in Germany
2.1 Experiences with underground hydrogen storage
Before the introduction of natural gas, town gas with a hydrogen content of more than 50% hydrogen was produced, transported and stored in Germany and Europe. Town gas was derived from coal, and its production still persists in China and Asia. When storage tanks could no longer meet the requirements of the gas industry, underground gas storage facilities were built in the porous geological subsurface starting from the 1950s. Pore storage systems were launched in Beynes (France), Lobodice (Czech Republic), Engelbostel (Hannover), Reitbrook (Hamburg), Hähnlein (Darmstadt), Kirchheiligen (Mühlhausen/Tühringen), Eschenfelden (Fürth/Oberpfalz), Ketzin (Brandenburg) even before the first salt caverns were put into operations in Bad Lauchstädt and Kiel in the 1970s (e.g., Jones and Machsen, 1963; Šmigáň et al., 1990; Sedlacek, 2002; Panfilov, 2016; Marcogaz, 2017). Today, these underground storage facilities have been converted to natural gas storage facilities or have since been abandoned. Currently, pure hydrogen (>95% H2) is solely stored in salt caverns at a few sites, namely, Teesside (United Kingdom) and Clemens Dome (United States), Spindletop (United States), and Moss Bloss (United States) (e.g., Panfilov, 2016; Zivar et al., 2021). Given this sparse and limited experience with hydrogen in pore storage systems, it is necessary to formulate recommendations for the safe handling of hydrogen in the subsurface.
2.2 Prospects for hydrogen network structures
The design of future hydrogen infrastructure and storage requirements in Germany remains uncertain and complex (Lux et al., 2022; Neuwirth et al., 2022). It is challenging to analyze underground storage requirements and infrastructures in such a highly complex and swiftly evolving environment. While the German National Hydrogen Strategy was published in 2020 to promote the use of hydrogen in all sectors of the hydrogen economy, it didn't specify the design of the hydrogen transport and storage infrastructure (BMWi, 2020). To examine the potential use of underground hydrogen storage in porous media, three inter-dependent variables that could impact future storage requirements in Germany have to be considered: 1) spatial distribution of hydrogen production via electrolysis, 2) hydrogen demand centres, and 3) hydrogen pipelines and import terminals.
Lux et al. (2022) investigated the positioning of electrolysers, underground storage and transport infrastructure in a greenhouse gas-neutral German energy system using five scenarios. Regardless of the scenario, their calculations indicate that 71% of the total hydrogen production in Germany will be located in the North and Baltic Seas, with a combined hydrogen production of 83–129 TWh by 2050 and total electrolyzer capacities of 37–55 GWel (ibid.). The concentration in these regions is due to the high potential for wind power, and it does not conflict with the alternative of expanding the electricity grid. Sens et al. (2022) report similar findings, as they calculate the second-lowest hydrogen supply cost at the North Sea, while Husarek et al. (2021) also support the idea of regional concentration, which contrasts with the results of Gils et al. (2021). Lux et al. (2022) demonstrate that hydrogen can serve as a seasonal and long-term storage medium, with a working gas volume of 42–104 TWh of hydrogen in 2050. In contrast, Gils et al. (2021) report a required storage capacity of 53 TWh in the same year. In the modelled energy systems, hydrogen storage levels decrease during winter when renewable electricity generation is lower and increase in summer with higher renewable electricity feed-in (Lux et al., 2022). This seasonal profile for hydrogen storage has been identified in previous studies by Gils et al. (2021) and Welder et al. (2018) for Germany, and Cárdenas et al. (2021), Samsatli and Samsatli (2019), Gabrielli et al. (2020), and Hernandez and Gençer (2021) for different regions or countries. Large-scale storage directly on site or at the end of a transport pipeline at the demand location can reduce hydrogen costs by decreasing excess electricity (Welder et al., 2018; Gils et al., 2021; Sens et al., 2022).
In order to establish a cost-effective hydrogen economy and a greenhouse-gas neutral energy system in the long run, a German-wide transport network is necessary (Gils et al., 2021). However, the design of such a network and the routing of its pipelines is still up for debate. Although detailed expansion plans differ, there is consensus in the literature on the need to connect production hubs and import terminals in the North with demand centers in the South of Germany (ibid.; Lux et al., 2022; Husarek et al., 2021). The European gas network operators have proposed a European-wide hydrogen pipeline vision (hydrogen backbone), which focuses on the repurposing of existing natural gas infrastructures (Wang et al., 2020; Wang et al., 2021). For example, in Germany, a North-South pipeline from Rostock to Lake Constance is planned to be established from 2035 onwards, repurposing the existing natural gas infrastructure, which is considered more cost effective than building new pipelines. The repurposing of pipelines and port infrastructure is also a part of the IPCEI Hydrogen initiative (important projects of common European interests). The association of the supra-regional gas transmission companies in Germany (FNB Gas) develops a joint network development plan every 2 years, which is then submitted to the German Federal Network Agency. The 2022 plan (FNB Gas, 2022) models a national hydrogen network in Germany, based on a survey of transportation needs. However, due to the current regulatory framework, this network is not legally binding. The 2020 plan (FNB Gas, 2020) only contained a rough outline of a visionary hydrogen network, in contrast to the more detailed model presented in the 2022 plan.
Assessing hydrogen storage requirements is a multi-faceted endeavour that includes an evaluation of potential future hydrogen demand and its regional distribution. Future hydrogen demand is still uncertain, according to Neuwirth et al. (2022), while Lux et al. (2022) indicate a range of hydrogen demand scenarios between 34 and 667 TWh (final energy demand in 2050), attributable to different usage categories such as industry, transport, and heating. To derive a more comprehensive estimate, Neuwirth et al. (2022) conducted a site- and process-specific bottom-up analysis of hydrogen demand from the energy-intensive sectors alone, revealing a potential hydrogen demand of 326 TWh/a. The spatial distribution of this demand is heterogeneous, with concentration in a few regions. Consequently, we posit that underground hydrogen storage is more likely in these regions, given that they are probable endpoints for pipelines, and industry clusters require an economic and steady hydrogen supply, which can be secured through storage.
3 Indicators for safe operations of hydrogen in pore storage systems
There are recommendations and evaluation schemes for the geotechnical and energetic use of the subsurface on a German and European level based on the operation of hydrocarbon reservoirs, underground storage facilities for both natural gas and CO2, the search for radioactive repositories and the utilization of geothermal resources (e.g., Sedlacek, 1999a; Sedlacek, 1999b; Chadwick et al., 2008; Reinhold et al., 2011; Alfarra et al., 2020; Stober and Bucher, 2021). Here we report positive and cautionary indicators for safe operations with hydrogen in underground pore storage systems based on a literature survey. The results are summarized in Table 1.
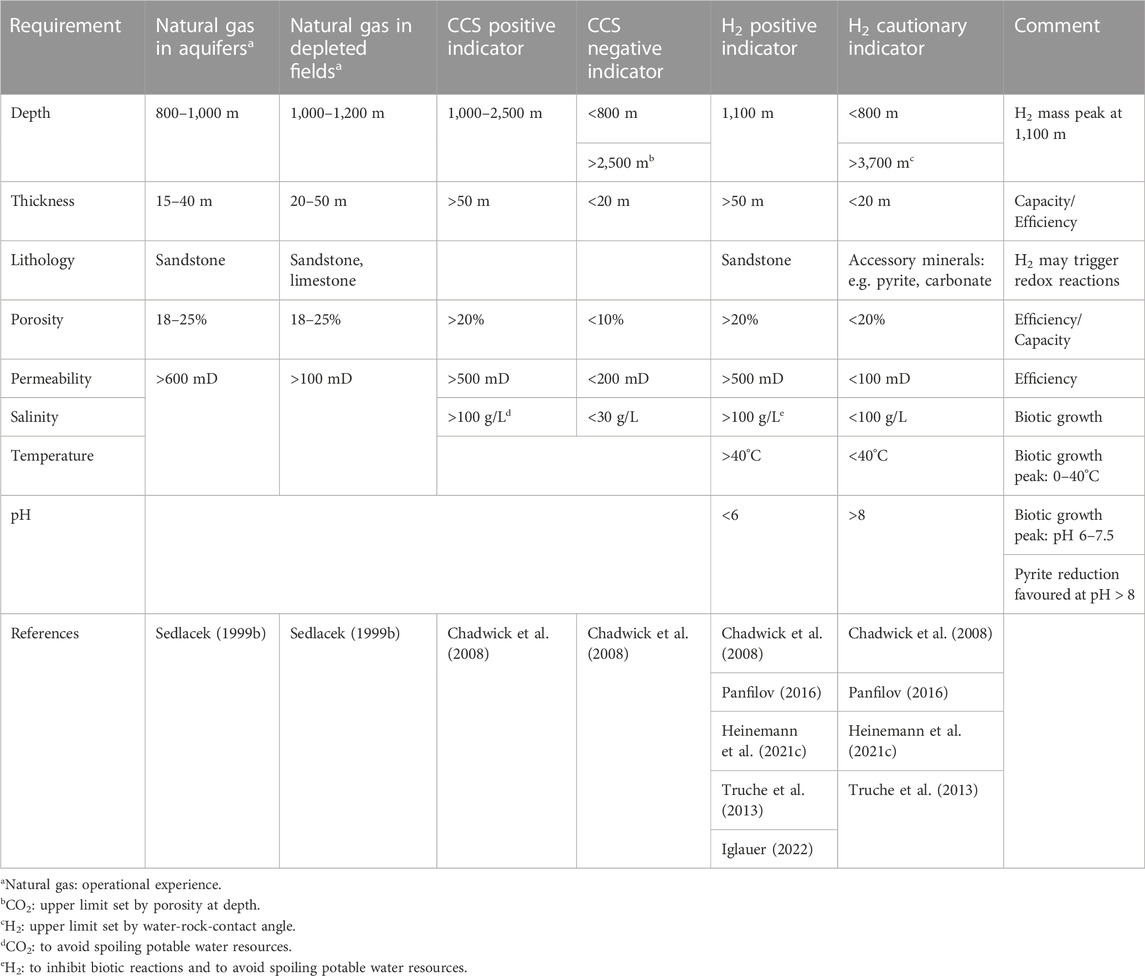
TABLE 1. Listing of requirements and positive and negative CCS and H2 indicators for underground gas storage in aquifers and depleted gas fields.
3.1 Biotic and abiotic reactions
Depleted fields and aquifers are composed of either sandstones or limestones. Sandstones, consist primarily of stable, non-reactive silicate minerals, namely, quartz and feldspar that will not react with hydrogen at reservoir conditions (Flesch, et al., 2018; Yekta et al., 2018). Major components in limestone reservoirs and mineral accessories in sandstones or in the caprock, however, may form reactions with hydrogen forming toxic gases such as hydrogen sulphide. Possible reactants include carbonate [CaCO3, MgCO3, (Ca, Mg) CO3], sulfate (CuSO4, CuSO4H2O), sulphide (S, FeS, FeS2, PbS, HgS, ZnS, Cu2S, CuFeS2, CS2), and oxide (Fe2O3, Fe3O4, FeO, MgO) minerals and reservoir gases such as CO, CO2, and hydrocarbons (Foh et al., 1979). However most of these reactions are insignificant at reservoir conditions, because hydrogen redox reactivity is kinetically limited due to its high bonding energy and thus most of the possible redox reactions remain insignificant at low temperature (Truche et al., 2013; Panfilov, 2016). However, experimental data by Truche et al. (2013) demonstrates that hydrogen induced reduction of pyrite into pyrrhotite is likely to produce sulphide at reservoir conditions and that the pH of the media is a critical parameter controlling the extent of the reaction at low temperature. This is consistent with field observations in the town gas storage field in Beynes, France, where pyrite reduction is believed to be responsible for the observed rise in hydrogen sulphide within the reservoir (Bourgeois et al., 1979). Although this represents a potential risk to underground hydrogen storage, the study by Truche et al. (2013) also shows that pyrite reduction could be prevented at acidic reservoir conditions.
In contrast to abiotic reactions, the extent and impact of which is discussed in the literature (e.g., Foh et al., 1979; Truche et al., 2010; 2013; Yekta et al., 2018), it is widely agreed that biotic processes may endanger hydrogen quality and/or lead to hydrogen consumption (Šmigáň, et al., 1990; Buzek, et al., 1994; Reitenbach et al., 1995; Panfilov, 2016; Heinemann et al., 2021c; Zivar et al., 2021). Several classes of microorganisms present at reservoir conditions are known for consuming hydrogen, which are methanogens, hydrogenotrophic sulfate reducers, homoacetogens and hydrogenotrophic iron (III)-reducing bacteria (Reitenbach et al., 1995; Panfilov, 2016; Heinemann et al., 2021c). Possible implications might include the production of methane (CH4), acetic acid (CH3COOH), and hydrogen sulphide (H2S) in addition to hydrogen loss (Reitenbach et al., 1995; Panfilov, 2016; Heinemann et al., 2021c). A well-known example for such biotic hydrogen loss is the Czech underground storage facility Lobodice in which methanogens were shown to transform hydrogen and carbon dioxide to methane, resulting in significant hydrogen reduction from 54 vol% (input) to 37 vol% (output) over a time period of 7 months (Šmigáň et al., 1990). Optimum conditions where growth peaks and critical conditions beyond which no growth is possible were summarized for several classes of microorganisms by Heinemann et al. (2021c) showing that most bacteria favour moderate temperatures (20–40°C), salinity (<100 g/L) and pH (6–7.5) [Heinemann et al. (2021c) and references therein].
3.2 Petrophysical properties of prospective pore storage systems
The petrophysical properties of prospective pore storage systems are important factors to consider when selecting a site for underground gas storage (e.g., Hematpur et al., 2023). It is noteworthy that the optimal porosity and intrinsic permeability values for rock formations utilized in gas storage may vary depending on the specific storage application and the type of gas being stored. For instance, high-pressure storage applications may necessitate higher permeability than low-pressure storage. Other factors such as effective stress state, temperature, and deformation state must also be considered when evaluating the suitability of a rock formation for underground storage.
Technically in UHS operations, a rock with high porosity and permeability is more desirable for hydrogen storage because it allows for higher storage volumes as well as higher injection and withdrawal rates representing the unloading and loading cycles, respectively. Higher energy efficiencies tend to be expected for high intrinsic permeability rock types when hydrogen is injected into them (Pan et al., 2021a). Furthermore, the hydraulic properties of the reservoir rock are a key factor influencing the interaction between fluid pressure and mechanical properties of the reservoir, known as hydro-mechanical coupling (e.g., Rutqvist and Stephansson, 2003; Bai and Tahmasebi, 2002). The permeability controls the fluid flow and thus the temporal and spatial distribution of fluid pressure, which in turn affects the effective stress state of the reservoir and its mechanical properties. This interaction must be considered and studied in the design and operation of UHS systems, as it can change the security and efficiency of the storage process to a significant degree. Zones of high permeability can reduce the risk of high fluid-pressure transients in the reservoir and around the wells, and thus the risk of inelastic rock deformation. Local high-pressure zones can form within low-permeability rocks, leading to aftershocks and induced seismicity in worst-case scenarios (e.g., Pawar et al., 2015; White and Foxall, 2016). In addition, low-pressure zones in the reservoir and around the wells can lead to a decrease in the production rate.
Although hydrogen storage has been extensively researched, to the authors’ knowledge, there is currently no recommendation as to which rock type, porosity, and permeability is best suited for UHS. Therefore, requirements for the effective porosity (i.e., connected porosity), intrinsic permeability and reservoir thickness for UHS operations are derived from the experience of the hydrocarbon and geothermal industry (Sedlacek, 1999b; May et al., 2004; Chadwick et al., 2008; Heidug, 2013; Stober and Bucher, 2021) as well as conceptual studies on potential international hydrogen storage in depleted reservoir settings: Chadwick et al. (2008) summarized that porosities and permeabilities of at least 0.20 and 500 mD (approx. 5 × 10−13 m2) are considered ideal geologic storage conditions. Porosities of less than 0.1 and permeabilities of less than 200 mD (approx. 2 × 10−13 m2) are considered cautionary indicators by these authors. Sedlacek (1999b) propounds that -from a geological and reservoir perspective-porosities and permeabilities within the range of 18 to 15% and greater than 100 mD are suitable for gas reservoirs, respectively. All these indicative limits are consistent with the study by Pfeiffer and Bauer (2015) for subsurface porous media hydrogen storage, which used on-site and off-site porosities and permeabilities of the Middle Rhaetian (main sandstone) of Northern Germany of 0.33 and 572 mD, respectively. Similar porosities (0.1–0.3) and permeabilities in the range of 20–2,500 mD are also used by other studies on UHS (Amid et al., 2016; Heinemann et al., 2018; Hemme and Berk, 2018; Zivar et al., 2021; Lysyy et al., 2021). On the basis of these literature values, we suggest permeabilities and porosities greater than 500 mD and 0.2, respectively, for optimal and safe operation of UHS systems (Table 1). However, the range of porosity and permeability depends on the specific properties of the rock formation, so a thorough petrophysical characterization of the rock is required in advance to determine its suitability for hydrogen storage. In addition to the consideration of intrinsic permeability, the characterization of relative permeability in wet porous rocks is crucial for optimizing storage capacity and recovery efficiency. The determination of the relative permeability of hydrogen-brine rock systems is very complex as it depends on the number of hydrogen injection and reproduction cycles as well as the capillary forces responsible for residual hydrogen trapping. Accordingly, these parameters should be determined based on experimental measurements to determine the intrinsic permeability.
3.3 Storage depth, sealing capacity, and integrity of caprock
The seal capacity of a reservoir refers to the ability of the rock layers above the reservoir (seals) to prevent the upward migration of fluids or gases out of the reservoir. The seal capacity is therefore critical for the effective trapping and storage of hydrogen in a reservoir, allowing for the efficient extraction and utilization of the resource. The dominant trapping mechanism is the capillary properties of the cap rock that will trap a hydrogen column until the net buoyancy (i.e., difference between hydrogen and brine) exceeds the capillary displacement pressure of the seal (Watts, 1987). The properties that define the capillary entry pressure are the size of the largest interconnected pore throat of the cap rock, the hydrogen-brine interfacial tension (IFT) and wetting behavior of the cap rock with respect to hydrogen and brine, expressed by the brine-rock-hydrogen contact angle
The hydrogen-water and hydrogen-brine interfacial tension was measured experimentally by Chow et al. (2018) and Hosseini et al. (2022) and was shown to decline with depth, but increase with salinity. However, under storage conditions (1,000 m and 50°C), the IFT remains relatively high (∼70 mN/m2) and hydrogen and brine remain immiscible (Chow et al., 2018; Hosseini et al., 2022; Iglauer, 2022). Hydrogen wettability of selected minerals were also measured and calculated in a few studies. Hydrogen wettability of quartz (pure and aged with stearic acid) were reported to increase with organic acid surface concentration, pressure and temperature (Iglauer et al., 2021). While pure quartz remained always strongly water-wet (brine contact angles <50°) for all conditions tested, aged quartz samples were weakly water-wet (brine contact angles 50–70°) for most scenarios examined (Iglauer et al., 2021). Al-Yaseri et al. (2021) and Al-Yaseri et al. (2022) reported contact angles for the clay brine and shale brine system based on empirical correlations as a function of pressure and temperature. All clays showed water wetting (
3.4 Cushion gas requirements
The capacity of any gas reservoir consists of two components: cushion gas (also known as base gas), which is used to maintain the minimum reservoir pressure and hence desired production levels, and working gas, which refers to the portion of gas that can be withdrawn and used during a storage cycle. In underground gas storage the composition of the working and cushion gas is generally the same. The total amount of cushion gas required depends mainly on the type of storage, with pore storages requiring higher cushion gas shares than salt caverns. However, no general recommendations can be made because the proportion of cushion gas in pore storage facilities depends on many reservoir parameters, such as storage volume, permeability and porosity, reservoir geometry, depth and the resulting pressure and temperature conditions. It also depends on technical configurations, such as well distributions, shut-in periods between injection and production, and the respective injection and production rates. In Germany, for example, the cushion gas share in natural gas pore storage facilities active and inactive ranges from 21 to 93% (Table 2).
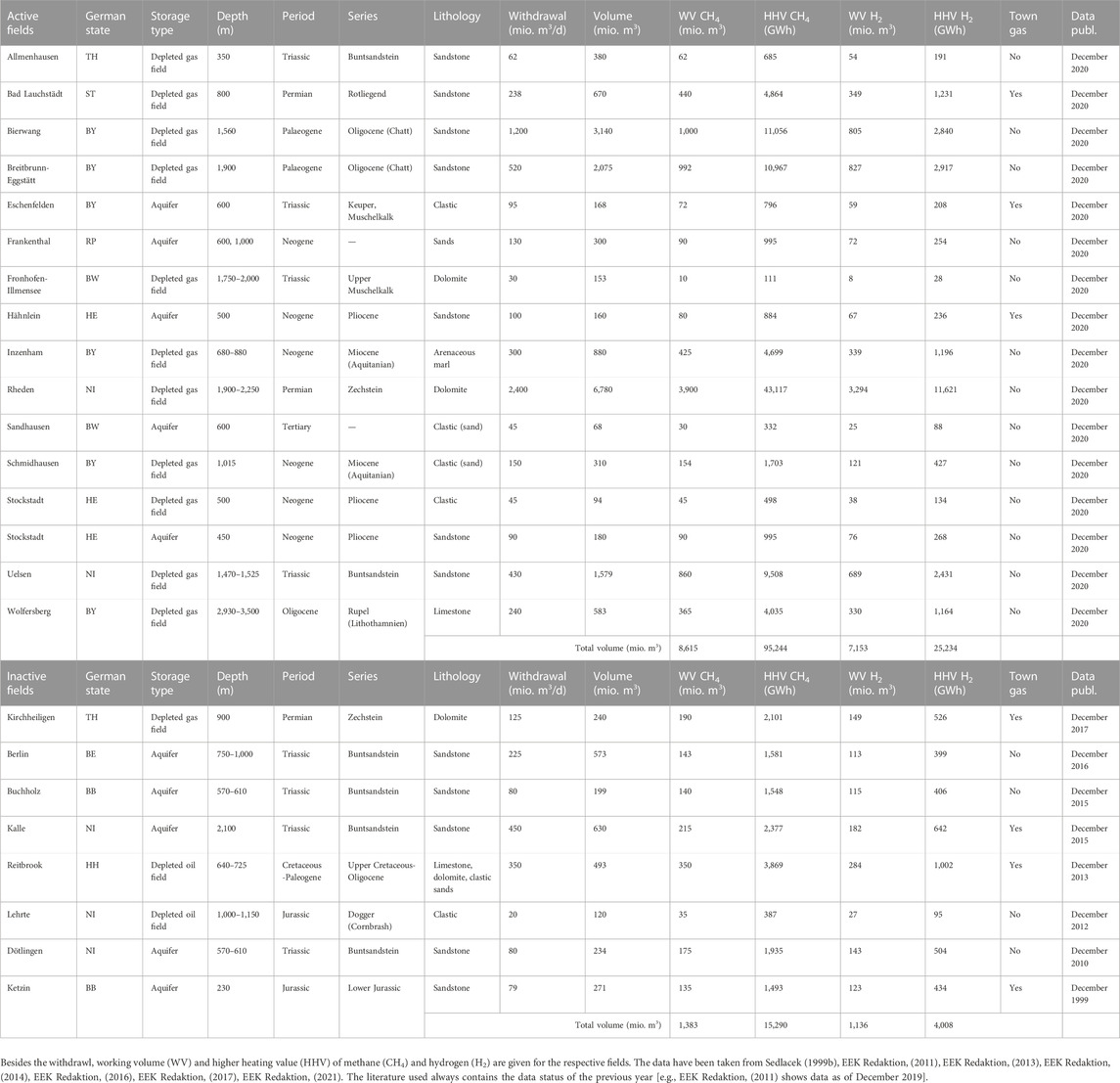
TABLE 2. Comparison of geological conditions and volumetric characteristics of existing active and inactive underground storage fields in Germany (see also Figure 3).
Unfortunately, there are no direct experiences with pure hydrogen in pore storages we can draw on, but numerical reservoir simulations demonstrate that aquifers and depleted hydrocarbon fields can be operated with reasonable hydrogen recovery rates of 78–93% (Pfeiffer and Bauer, 2015; Feldmann et al., 2016; Sáinz-García et al., 2017; Heinemann et al., 2021b; Kanaani et al., 2022). Simulations of saline aquifers show that the injected cushion gas can efficiently displace formation water from injection and production wells, creating the conditions for subsequent hydrogen injection and withdrawal with significant improvements in UHS performance (Sáinz-García et al., 2017; Heinemann et al., 2021b; Kanaani et al., 2022). This also results in a lower demand for cushion gas in deeper structures and reservoirs with higher permeabilities (Heinemann et al., 2021b). Since hydrogen in particular involves considerable upfront costs, alternative cushion gas compositions such as nitrogen, CO2 or natural gas have been discussed in the literature to cut costs and/or CO2 emissions (Foh et al., 1979; Pfeiffer and Bauer, 2015; Feldmann, et al., 2016; Kanaani et al., 2022; Rhouma et al., 2022). Hence, the possibility of mixing with an inert cushion gas that is present in the reservoir must be considered (Foh et al., 1979). Gas mixing depends on viscosity and density contrasts (see Figures 1, 2), and is governed by diffusion and dispersion (Tek, 1989; Feldmann et al., 2016). A process that is particularly important to consider when converting depleted hydrocarbon fields with high gas saturation and low reservoir pressures (Feldmann et al., 2016). However numerical simulations of depleted gas reservoirs indicate that undesired physical phenomena such as viscous fingering caused by mobility and density contrasts are of minor importance in gas saturated reservoirs (Feldmann et al., 2016). Numerical simulations in saline reservoirs with hydrogen and nitrogen as working and cushion gas, respectively, demonstrate likewise the overall feasibility, but stress the importance of optimized injections schemes as the volume-weighted average hydrogen fraction of the produced gas increased from 52% during the first cycle to 85% in the fourth cycle (Pfeiffer and Bauer, 2015). In a most recent study of aquifer storage and withdrawal capacity, Kanaani et al. (2022) demonstrated likewise the operational feasibility and indicated that methane outperforms CO2 and nitrogen in terms of hydrogen recovery. The general feasibility has also been demonstrated in converted town gas storages across Europe (Foh et al., 1979; Foh, 1991). At Beynes, for example, natural gas was being injected on one side of the reservoir from 1972 while town gas had been withdrawn from the other side of the reservoir, replacing all of the working and 40% of the cushion gas with natural gas (Foh et al., 1979; Foh, 1991). Despite the fact that 60% of town gas resided in the reservoir, only minimal mixing of gases occurred during conversion, and less than 1% of the withdrawn gas consisted of the original town gas after conversion was completed in 1973 (Foh, 1991). It follows that detailed reservoir models are required to draw accurate conclusions about the demand and cushion gas requirements for any pore storage system, despite its general feasibility.
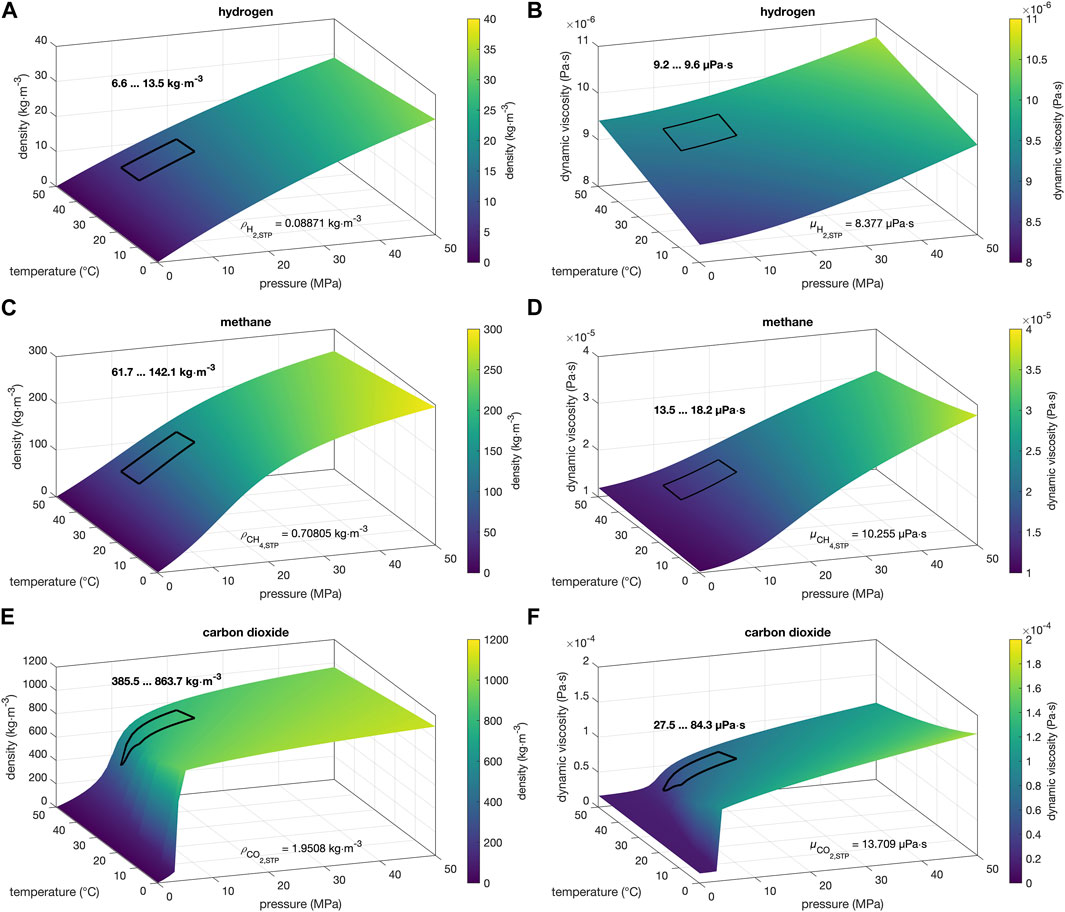
FIGURE 1. Pressure and temperature dependence of (A,C,E) density and (B,D,E) dynamic viscosity of (A,B) hydrogen, (C,D) methane, and (E,F) carbon dioxide under conditions for UHS systems. All density and dynamic viscosity values shown are from the NIST Chemistry web book database (Lemmon et al., 2023) and were calculated according to Leachman et al. (2009) (hydrogen), Setzmann and Wagner (1991) (methane), and Span and Wagner (1996) (carbon dioxide). The areas marked by black rectangles indicate the ranges of density and dynamic viscosity expected at the proposed optimal reservoir depths (see text). The values indicated with the STP indices refer to the corresponding values at standard pressure and temperature conditions.
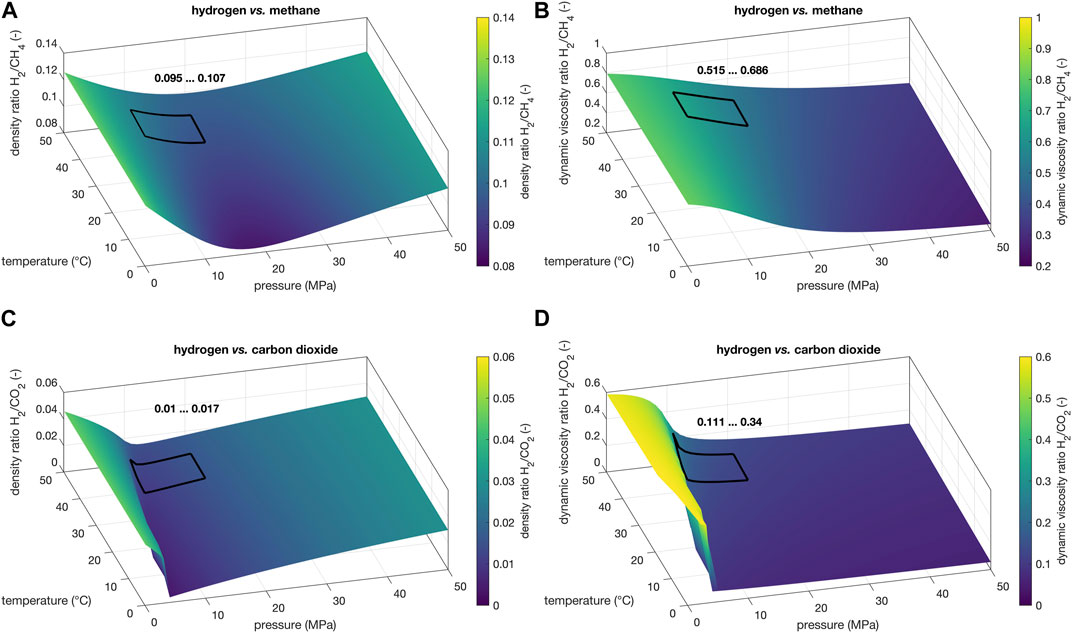
FIGURE 2. Pressure and temperature dependence of (A,C) density ratio and (B,D) dynamic viscosity ratio of hydrogen relative to (A,B) methane and (C,D) carbon dioxide under conditions for UHS systems. All density ratios and dynamic viscosity ratios shown are from the NIST Chemistry web book database (Lemmon et al., 2023) and were calculated according to Leachman et al. (2009) (hydrogen), Setzmann and Wagner (1991) (methane), and Span and Wagner (1996) (carbon dioxide). The areas marked by black rectangles indicate the ranges of density ratios and dynamic viscosity ratios expected at the proposed optimal reservoir depths (see text).
3.5 Hydrogen solubility and losses
The solubility of hydrogen in brine water depends on a number of factors, including temperature, pressure, and salinity, but it is generally lower compared to other gases such as CO2 or CH4 (e.g., Zivar et al., 2021). Hence, less loss due to dissolution is expected compared to other gases. Experimental and modelled data suggest that hydrogen solubility increases with increasing pressure, but decreases with increasing salinity resulting in less than 0.001 of mole fraction of hydrogen at storage conditions (Wiebe and Gaddy, 1934; Li et al., 2018; Chabab et al., 2020). This is important, because dissolved hydrogen may diffuse and leak out of the reservoir resulting in hydrogen loss. The rate of diffusion will likewise depend on a number of factors, including the temperature and pressure. Diffusion rates of hydrogen in brine water can be estimated using theoretical and empirical relationships (Einstein, 1906; Wilke and Chang, 1955), but have also been examined experimentally (Akgerman and Gainer, 1972), showing that at storage conditions, the rate of hydrogen diffusion is approximately twice as high when compared to methane and three times as high when compared to carbon dioxide (Akgerman and Gainer, 1972).
3.6 Thermophysical parameters of hydrogen at underground storage conditions
Comprehension of the thermophysical properties of hydrogen is essential for a detailed consideration of its storage potential in UHS systems. In particular, with regard to the storage of hydrogen in aquifers and depleted hydrocarbon reservoirs, a detailed consideration of its two thermophysical properties, density and viscosity, is essential under pressure and temperature conditions prevailing in the storage system. For this application, it is also useful to compare these properties of hydrogen with those of methane (CH4) and carbon dioxide (CO2) (Figures 1, 2). All thermophysical properties of H2, CH4, and CO2 presented here were obtained from the NIST chemistry web book database (Lemmon et al., 2023) and are intended to serve as a reference for upcoming UHS systems.
One of the most notable thermophysical characteristics of hydrogen is its low density, which dictates that it must be compressed or liquefied for any practical application (Osman et al., 2021). At standard temperature (0°C or 273.15 K) and pressure (0.1 MPa or 1 bar), the density of hydrogen is 0.08871 kg/m3 according to the equation of state for normal hydrogen (Leachman et al., 2009). Due to the low critical temperature (−240.005°C or 33.145 K) and low critical pressure (1.2964 MPa or 12.964 bar) of hydrogen (Leachman et al., 2009), it would be stored in the gaseous phase in porous storage systems (Züttel, 2004). Hydrogen is a highly compressible gas, and its density increases significantly with increasing pressure and barely with increasing temperature (Figure 1A). Consequently, hydrogen storage efficiency increases with increasing reservoir depth. But, because CH4 (Setzmann and Wagner, 1991) and CO2 (Span and Wagner, 1996) is much denser than hydrogen (Figures 2A, C), storing hydrogen gas of the same mass requires higher pressure (Tarkowski et al., 2021) and less hydrogen can be stored in the same reservoir volume compared to CH4 and CO2 (e.g., Lanz et al., 2001; Lankof and Tarkowski, 2020; Zivar et al., 2021; Epelle et al., 2022). This underlines the importance of storage capacity in hydrogen storage. In this context, depleted hydrocarbon storage facilities represent a more interesting storage option compared to salt caverns and aquifers due to their large storage space and high availability (e.g., Reitenbach et al., 2015). Moreover, the large density difference between H2 and dense formation water and cushion gas (e.g., CH4) can cause a strong gravity segregation effect (Ide et al., 2007; Jamshidnezhad et al., 2010; Rossen et al., 2010; Han et al., 2016; Rabinovich and Cheng, 2020), resulting in the accumulation of a hydrogen cap beneath the cap rock (Ruith and Meiburg, 2000; Heinemann et al., 2021c; Muhammed et al., 2022), which could ensure that H2 will remain in the reservoir (Iglauer, 2022). Conversely, Osman et al. (2021) emphasized that an H2 plume is susceptible to formidable buoyancy forces, with the magnitude of said forces directly correlating to the potential for hydrogen leakage. Literature by Heinemann et al. (2021c) and Sáinz-García et al. (2017) suggests that water may ascend towards the production well under these conditions, making seasonal storage of hydrogen in porous storage systems challenging.
While the hydrogen density and thus the compressibility in the depth range of UHS systems mainly increases with increasing pressure (Figure 1A), the dynamic viscosity increases significantly with increasing temperature (Figure 1B). In the range of standard conditions up to pressures of 50 MPa and temperatures of 50°C, the viscosity shows a comparable temperature and pressure dependence. For example, hydrogen viscosity increases by about 15% from standard temperature and pressure conditions to an elevated temperature of 50°C (at standard pressure; Leachman et al., 2009). At standard temperature, viscosity increases by about 18% in the pressure range from 0.1 to 50 MPa (Leachman et al., 2009). In direct comparison, the viscosities of CH4 and CO2 are larger than those of H2, and both show a dominant pressure dependence that far exceeds the behavior of H2 viscosity (Figures 2B, D). Hence, hydrogen has a much higher mobility than CH4 and CO2. The viscosities of H2 and CH4 are still most similar here in comparison and are in the same order of magnitude. As elaborated by Muhammed et al. (2023), underground hydrogen storages could thus be used as a direct substitute for CH4 storage. Due to their viscosities, according to Edlmann et al. (2016) and Heinemann et al. (2018), H2 and CH4 can be stored in a wide range of underground reservoirs because they are not limited by reservoir conditions or depth. Rather, reservoirs are determined based on the geologic characteristics of the reservoirs, as appropriate containment features are required to seal against these mobile gases (Muhammed et al., 2023). But, Paterson (1983) and numerous subsequent studies have shown that the low density, viscosity, and molecular size of H2 result in more viscous fingering and poorer conformation during injection, faster migration toward structurally high sites, and some amount of unrecoverable hydrogen (e.g., Hagemann et al., 2014; Feldmann et al., 2016). For this reason, Epelle et al. (2022), for example, recommend adjusting the flow rate of hydrogen and the size of the wellbore (Sørensen, 2007; Zivar et al., 2021) compared to operation with natural gas to minimize diffusion in porous media.
As previously described by us and other studies (e.g., Iglauer, 2022), the optimal depth, d, for UHS systems is in the range of 800–1,100 m depth. Assuming a mean surface temperature of 8.2°C and an average geothermal gradient of 32°C/km (Agemar et al., 2012), a temperature range of 33.8–43.4°C results for the target depth. For an average density, ρlith, of the overlying rock of 2,300–2,600 kg/m3 and a lithostatic factor, Flith, of 0.5–0.7 (Foh et al., 1979), the pressure at which hydrogen could be stored in UHS systems can be determined using the following simple relationship:
This temperature and pressure range corresponds to the optimal conditions for the geological storage of hydrogen in porous storage systems (black rectangles within Figures 1, 2). The so-called lithostatic factor was introduced here to represent the ratio of working pressure to lithostatic pressure in the reservoir. For this typical geological conditions for porous media storage, hydrogen has a low density and viscosity in the narrow ranges of 6.6–13.5 kg/m3 and 9.2–9.6 μPa·s, respectively (Figures 1A, B). The influence of temperature and pressure variations on the density and viscosity of highly compressible hydrogen is thus almost negligible at this reservoir depth. In contrast, the thermophysical properties of CO2 and CH4 behave in a much more pressure- and temperature-dependent manner, which is reflected in the relative density and viscosity ratios (Figure 2). Within the proposed reservoir range, the density and viscosity ratios of H2 and CH4 do not vary too much (max. ±25%; Figures 2A, B), whereas the corresponding ratios of H2 to CO2 can vary by up to a factor of about 2–3 (Figures 2C, D). This has to be considered when re-using former natural gas reservoirs with H2.
4 Capacity estimates for UHS in Germany
4.1 Technical hydrogen storage capacity in former and current UGS systems
Underground gas storage facilities can be adapted for hydrogen storage and some of the currently operating underground storage facilities have already stored town gas with a hydrogen content of about 50–60% in the past. Thus, in a first step, we focus on the installed capacity of underground pore storages in Germany. The hydrogen storage capacity is calculated based on the recoverable volume of natural gas as reported by the State Authority for Mining, Energy and Geology in Germany (Landesamt für Bergbau, Energie und Geologie, LBEG). These data are based on empirical values, which are derived from technical and economic considerations for natural gas and, thus, provide sound estimates of the dynamic recovery of hydrogen. The amount of energy stored as hydrogen in the working gas, which describes the portion of gas that is cycled in and out of the reservoir,
where
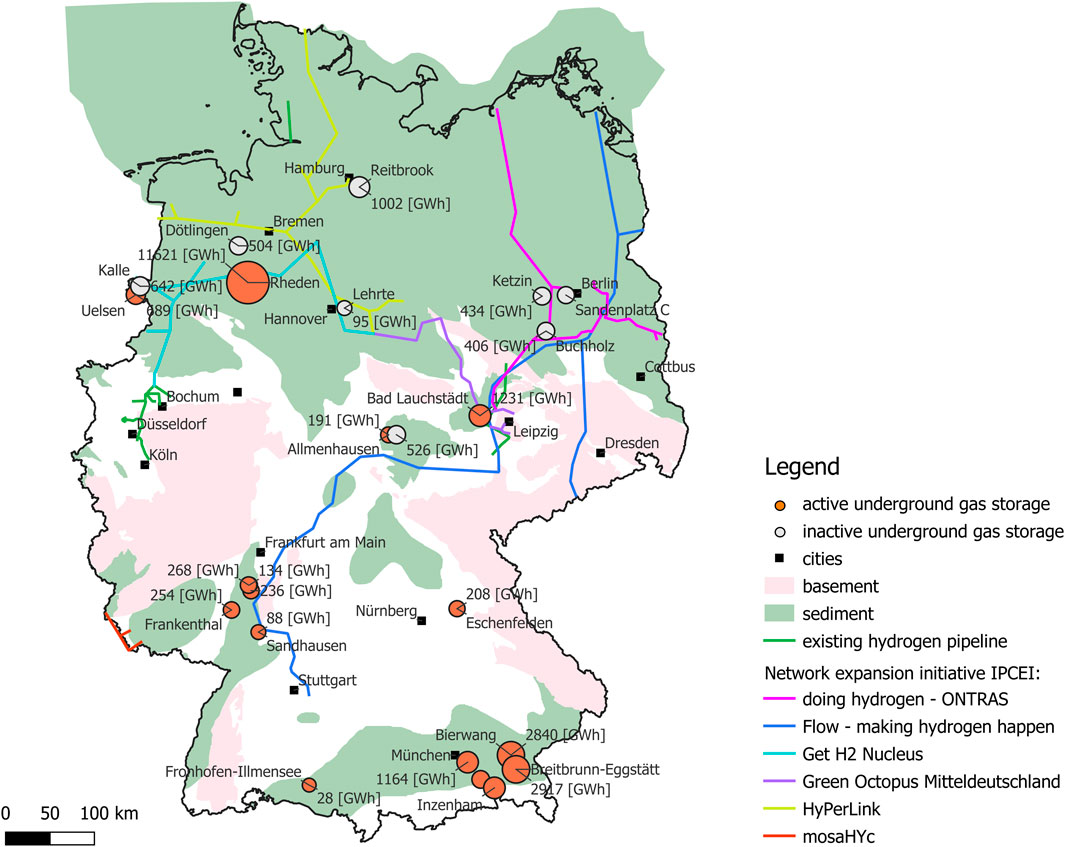
FIGURE 3. Simplified overview map of current hydrogen pipelines and future development plans (IPCEI projects) with potential underground hydrogen storage locations in Germany (green shaded areas). Red shaded areas represent basement rocks, where no pore storage facilities are possible. Green areas indicate the spread of sedimentary rocks and saline aquifers, in which a total of 3.2–27.3 PWh of hydrogen energy could be stored. Red dots indicate the storage location and storage potential of hydrogen (TWh) in UGS systems that could be converted to UHS facilities in the future. Grey shaded dots represent decommissioned UGS systems and their corresponding storage capacity in terms of hydrogen energy equivalent (TWh).
4.2 Theoretical hydrogen storage capacity in saline aquifers
Additional underground storage capacities may become available in saline aquifers that hold the highest storage potential due to their widespread occurrence. In order to assess the hydrogen storage capacity in saline aquifers, we employ the volumetric assessment of CO2 storage capacities in saline aquifers, as previously conducted by Knopf and May (2017). This probabilistic approach yields a proven, median, and possible CO2 storage capacity of 20.4 Gt (P10), 49.1 Gt (P50), and 115.3 Gt (P90) for all evaluated rock units (onshore and offshore; green shaded areas in Figure 3), respectively. Most of these resources are located in the North German Basin with estimated CO2 storage capacities ranging from 19.3 Gt (P10)–108.6 Gt (P90) (Knopf and May, 2017). By applying this data, we can estimate the thermal hydrogen energy content using
where
5 Discussion
5.1 Storage capacities and requirements in Europe
Existing and decommissioned underground pore storage facilities may account for up to 30 TWh of energy equivalent of hydrogen, but are unlikely to meet future storage requirements of 34–667 TWh (Lux et al., 2022), not least because an overall conversion of all storage facilities from natural gas to hydrogen is unlikely. To meet these future storage requirements, additional capacities may be needed in the form of depleted hydrocarbon fields and saline aquifers. Approximately 3.2–27.3 PWh of hydrogen energy equivalent could be stored in saline aquifers in Germany, which is comparable to the technical storage potential in salt caverns that has been estimated at 35.7 PWh (Caglayan et al., 2020) and similar to the hydrogen storage potential in saline aquifers in the UK with an estimated capacity of 2.1 PWh of energy equivalent of hydrogen (Scafidi et al., 2021). In practice, many of the storage sites may, however, prove unsuitable, given the technical, economic and social constraints or not least because of the increasing competition between different geotechnical applications, be it geothermal energy or storage of natural gas, CO2, or hydrogen (Suchi et al., 2014).
Other additional storage options that have been discussed in the literature include depleted gas and oil fields, which are considered appropriate for hydrogen underground storage because of their proven containment of natural gas, well explored subsurface, and developed capacity and infrastructure (e.g., see reviews by Raza et al., 2022; Zivar et al., 2021; Heinemann et al., 2021c). Capacity estimates in the United Kingdom and the Netherlands show large potentials. Scafidi et al. (2021) identified 95 suitable depleted gas fields on the UK continental shelf with a total working gas capacity of 6.9 PWh energy equivalent of hydrogen. This estimate is slightly higher than the total hydrocarbon storage capacity in gas fields reported by Mouli-Castillo et al. (2021), which is based on the initial gas-in-place capacity of the respective hydrocarbon fields resulting in 2.7 PWh energy equivalent of hydrogen. Large storage potentials in depleted gas fields were also predicted for the Netherlands, one of the largest producers of natural gas in Europe. Estimates based on the original-gas-in-place analysis show that onshore capacities of 277 TWh and offshore capacities of 179 TWh could add up to a total of 456 TWh energy equivalent of hydrogen in the Netherlands, which is an order of magnitude higher than the estimated hydrogen storage potential in salt caverns of 43 TWh. In Germany, too, depleted gas and oil fields could offer large storage capacities, but estimates on the original gas in place capacity are not publicity available, only cumulative production numbers (LBEG, 2021). Cumulative gas production from all fields at STP is 1.058512 × 1012 m3 (current as of 1 January 2021; LBEG, 2021). Of this, 114.719 × 109 m3 of natural gas was produced from gas fields that are depleted today, most of it in Lower Saxony (LBEG, 2021).
However, more important than uncertain volume estimates, is the question what proportion of this total can be utilized for underground hydrogen storage under the parameters presented here. For example, as fields in the Weser-Ems and Elbe-Weser sector located in the Rotliegend formation may reach depths of 2,500–5,000 m (LBEG, 2021). From an economic point of view, storing gas at these depths is not profitable due to the high compression costs (e.g., Foh et al., 1979). Typical depths of natural gas storage facilities are 1,000–1,200 m (Sedlacek, 1999a). Physical constraints limit the storage of hydrogen to depth of 3,700 m below which hydrogen is thought to percolate through the cap rock and, hence, confinement loss (Iglauer et al., 2021). Details on the specific gas production, horizon and depth are, however, not listed. Former gas deposits in carbonates of the Stassfurt formation (Ca2), common in the eastern part of the North German Basin and the Thuringian Basin, may prove unsuitable as they contain significant amounts of hydrogen sulphur. However, former gas deposits in the Tertiary sandstones in the Upper Rhine Graben could again show suitable potential and supplement storage capacities in saline aquifers and the UGS facilities Stockstadt, Hähnlein, and Frankenthal. According to forecasts, this could be the end point of the North-South hydrogen pipeline. However, the nearby industrial clusters would depend on an economical and steady supply of hydrogen, which could be ensured by underground hydrogen storage facilities. For the Munich area, on the other hand, there are no pipeline plans according to the Gas Network Development Plan 2022–2032 or the IPCEI project plan. The question, therefore, arises as to how the supply will be ensured, and here, too, underground hydrogen storage could be of significance. Future research should therefore focus on the technical storage potential matching it with planned hydrogen infrastructures and industrial demands. This is of paramount importance in the South of Germany where storage potential is lower than in the North, making targeted planning necessary.
5.2 Assessment of underground hydrogen storage costs
Early economic analyses have shown that the cost of service for hydrogen is approximately equal to the costs of storing natural gas, but have stressed that the costs are very site specific and that a range of costs is possible for each type of underground storage (Foh et al., 1979). In a more recent life cycle cost analysis, Lord et al. (2011) conclude on a cost per kg of hydrogen basis that depleted hydrocarbon reservoirs and aquifers would be the economically attractive options, considering costs for pipelines and wells, compressors, cavern mining, and base gas. The overall costs for UHS amount to 1.6 $/kg for salt caverns, 0.04 $/kg for depleted hydrocarbon fields, 0.08 $/kg for aquifers, and 2.75 $/kg for hard rock caverns (Lord et al., 2011). This methodology has been adapted with most recent cost estimates as of 2019 in a review by Raza et al. (2022) for UHS to be 0.14–14.03 $/kg (0.004–0.421 $/kWh) for salt caverns, 1.42 $/kg (0.043 $/kg) for depleted hydrocarbon fields, 1.49 $/kg (0.045 $/kWh) for aquifers, and 0.36–3.20 $/kg (0.011–0.096 $/kWh) for hard rock caverns. However, a comparison of natural gas storage costs in Europe and the United States shows that storage costs in Europe could be twice as high, not least because of higher regulatory requirements (Sedlacek, 1999a). But unlike in the United States, where these figures are sometimes published, cost estimates in Europe are rather poor (Sedlacek, 1999a). Something that all cost estimates for hydrogen pore storages have in common is the substantial influence of cushion gas on the ultimate costs of service, similar to natural gas storage facilities (Foh et al., 1979; Lord et al., 2011). At the same time it is considered more economic to leave some gas in the reservoir than to invest in the additional pumps and compressors to empty it completely (Foh et al., 1979). Therefore, one way to reduce these costs is to use lower cost cushion gas, but further research is needed to investigate the possibility of mixing, viscous fingering and gravity override that needs to be considered (see Section 3.4; Feldmann et al., 2016). The economics will therefore depend on the quantity of the cushion gas, the quality of the recovered hydrogen gas, and costs of gas separation if required (Foh, 1991).
5.3 Hydrogen purity requirements
Probably the most important parameters influencing the economic development of underground hydrogen storage are biotic and abiotic reactions. Potential contaminations may result from biotic reactions, such as for instance in the town gas storage Lobodice (Czech Republic) in which methanogens were shown to transform hydrogen and carbon dioxide to methane (Šmigáň et al., 1990). Or abiotic reactions like in the in the town gas storage Beynes (France) where pyrite reduction is believed to be responsible for the observed rise in hydrogen sulphide within the reservoir (Bourgeois et al., 1979). There are several process engineering options for removing hydrogen sulphide from natural gas and the hydrocarbon industry has decades of experience. In Germany, too, a large proportion of all natural gas reserves contain varying amounts of hydrogen sulphur, which have been processed at the Großenkneten desulfurization plant (Lower Saxony) since the 1970s. Here the hydrogen sulphur is removed from the gas and converted to elemental Sulphur used as feedstock by the chemical industry. However, this is a costly process and the purity requirements of hydrogen extracted from the storage facility will ultimately depend on the end user. Fuel cells, for example, require hydrogen of 99.97% purity according to Beuth Verlag GmbH, (2019) because contaminants such as carbon monoxide and hydrogen sulfide can impede electrode charging, while ammonia causes irreversible effects on fuel cell performance. In contrast, fuel quality specification for applications other than PEM fuel cell, i.e., combustion processes in hydrogen boilers will tolerate higher concentrations of impurities of about 98% (ISO, 2019). In contrast, industrial combustion applications (e.g., for sintering processes, heating of furnaces) and domestic appliances (boilers, cookers, etc.) are more tolerant with respect to the hydrogen content and impurity levels resulting in fuel-types similar to “town gas” with hydrogen contents of around 50%. Generally, these higher impurity levels could facilitate hydrogen utilization by increasing the heating value but replacement by other components, e.g., oxygen or carbon monoxide have to be considered carefully with regards to safety, decarbonization and cost-effective level of purity for end user applications and quality measurements.
6 Summary
Hydrogen has the capacity to meet future emission targets by decarbonizing the industry, mobility, electricity, and heat generation. But, hydrogen utilization and the implementation of a hydrogen economy at large scale requires significant storage capacities. Underground hydrogen storage in porous geological structures has the potential to meet these storage requirements. Positive and cautionary indicators for safe hydrogen storage in porous structures were identified, and a comparison and linkage with existing and planned aboveground hydrogen infrastructures was established. We have identified porous sandstone formations located at depths ranging from 800 to 1,100 m and exhibiting temperatures exceeding 40°C as highly promising reservoirs for hydrogen storage. Specifically, porosities exceeding 20% and permeabilities greater than 500 mD were identified as positive indicators for adequate storage capacity. Brine pH values below 6 and salinities above 100 mg/L are desirable for effective hydrogen storage that will minimize biotic and abiotic reactions. Based on our estimates, the total hydrogen storage capacity in underground natural gas facilities is projected to be as high as 8 billion cubic meters, or equivalently, 0.72 Mt at standard temperature and pressure. This capacity translates into a potential energy equivalent of 29 TWh of hydrogen. Additionally, saline aquifers could accommodate 81.6–691.8 Mt of hydrogen, equivalent to an energy potential of 3.2–27.3 PWh of hydrogen that would meet predicted storage requirements many times over. Most of these sites are located in the North German Basin. We conclude that pore storage systems are likely to play a vital role in the future German hydrogen infrastructure, particularly in regions with significant demand for industrial hydrogen (storage) and potential imports via pipelines and ships. We recommend that future research focus on assessing the technical storage potential of these sites and their compatibility with planned hydrogen infrastructures and industrial demand.
Data availability statement
The original contributions presented in the study are included in the article/Supplementary Material, further inquiries can be directed to the corresponding author.
Author contributions
KA designed the study. KA and BA organized the database. BA performed the thermophysical analysis. KA wrote the first draft of the manuscript. KA, BA, MG, and MN wrote sections of the manuscript. All authors contributed to manuscript revision, read, and approved the submitted. All authors listed have made a substantial, direct, and intellectual contribution to the work and approved it for publication.
Funding
This work is part of the flagship project TransHyDE supported by the German Federal Ministry of Education and Research (Bundesministerium für Bildung und Forschung BMBF) with the grant number: 03HY202H.
Acknowledgments
We thank Emily Bergup for graphical assistance and Dirk Boernecke for fruitful discussions. The original manuscript was improved by reviews by Bashirul Haq and Wei Liu.
Conflict of interest
The authors declare that the research was conducted in the absence of any commercial or financial relationships that could be construed as a potential conflict of interest.
Publisher’s note
All claims expressed in this article are solely those of the authors and do not necessarily represent those of their affiliated organizations, or those of the publisher, the editors and the reviewers. Any product that may be evaluated in this article, or claim that may be made by its manufacturer, is not guaranteed or endorsed by the publisher.
References
Agemar, T., Schellschmidt, R., and Schulz, R. (2012). Subsurface temperature distribution in Germany. Geothermics 44, 65–77. doi:10.1016/j.geothermics.2012.07.002
Akgerman, A., and Gainer, J. L. (1972). Predicting gas-liquid diffusivities. J. Chem. Eng. Data 17 (3), 372–377. doi:10.1021/je60054a008
Al-Yaseri, A., and Jha, N. K. (2021). On hydrogen wettability of basaltic rock. J. Petroleum Sci. Eng. 200, 108387. doi:10.1016/j.petrol.2021.108387
Al-Yaseri, A., Wolff-Boenisch, D., Fauziah, C. A., and Iglauer, S. (2021). Hydrogen wettability of clays: Implications for underground hydrogen storage. Int. J. Hydrogen Energy 46 (69), 34356–34361. doi:10.1016/j.ijhydene.2021.07.226
Al-Yaseri, A., Yekeen, N., Mahmoud, M., Kakati, A., Xie, Q., and Giwelli, A. (2022). Thermodynamic characterization of H2-brine-shale wettability: Implications for hydrogen storage at subsurface. Int. J. Hydrogen Energy 47 (53), 22510–22521. doi:10.1016/j.ijhydene.2022.05.086
Alfarra, A., Bertrams, N., and Bollingerfehr, W. (2020). RESUS. Fundamentals for the evaluation of a repository system in a clay formation of greater thickness (No. GRS-571). Köln, Germany: Gesellschaft für Anlagen-und Reaktorsicherheit (GRS) GmbH.
Ali, M., Pan, B., Yekeen, N., Al-Anssari, S., Al-Anazi, A., Keshavarz, A., et al. (2022). Assessment of wettability and rock-fluid interfacial tension of caprock: Implications for hydrogen and carbon dioxide geo-storage. Int. J. Hydrogen Energy 47 (30), 14104–14120. doi:10.1016/j.ijhydene.2022.02.149
American Gas Association (1997). Survey of underground storage of natural gas in the United States and Canada 1996. Arlington: American Gas Association.
Aslannezhad, M., Ali, M., Kalantariasl, A., Sayyafzadeh, M., You, Z., Iglauer, S., et al. (2023). A review of hydrogen/rock/brine interaction: Implications for Hydrogen Geo-storage. Prog. Energy Combust. Sci. 95, 101066. doi:10.1016/j.pecs.2022.101066
Bai, T., and Tahmasebi, P. (2022). Coupled hydro-mechanical analysis of seasonal underground hydrogen storage in a saline aquifer. J. Energy Storage 50, 104308. doi:10.1016/j.est.2022.104308
Beuth Verlag GmbH (2019). Din en 17124, 2022-12: Wasserstoff als Kraftstoff - Produktfestlegung und Qualitätssicherung - Protonenaustauschmembran (PEM) - Brennstoffzellenanwendungen für Straßenfahrzeuge. Berlin, Germany: Beuth Verlag GmbH. doi:10.31030/2839235
BMWi (2020). Nationales Reformprogramm 2020 – die nationale Wasserstoffstrategie. Berlin: Bundesministerium für Wirtschaft und Energie, 29.
Bourgeois, J. P., Aupaix, N., Bloise, R., and Millet, J. L. (1979). Formation of hydrogen sulfide in underground storage reservoirs by reduction of sulfurous minerals in reservoir rocks. France: Rev. Inst. Fr. Pet., 34.
Buzek, F., Onderka, V., Vančura, P., and Wolf, I. (1994). Carbon isotope study of methane production in a town gas storage reservoir. Fuel 73 (5), 747–752. doi:10.1016/0016-2361(94)90019-1
Caglayan, D. G., Weber, N., Heinrichs, H. U., Linßen, J., Robinius, M., Kukla, P. A., et al. (2020). Technical potential of salt caverns for hydrogen storage in Europe. Int. J. Hydrogen Energy 45 (11), 6793–6805. doi:10.1016/j.ijhydene.2019.12.161
Cárdenas, B., Swinfen-Styles, L., Rouse, J., and Garvey, S. D. (2021). Short-Medium-and long-duration energy storage in a 100% renewable electricity grid: A UK case study. Energies 14 (24), 8524. doi:10.3390/en14248524
Cedigaz (2021). Underground gas storage in the world – 2021. https://www.cedigaz.org/underground-gas-storage-in-the-world-2021-status/ (last accessed on 02 22, 2023).
Chabab, S., Theveneau, P., Coquelet, C., Corvisier, J., and Paricaud, P. (2020). Measurements and predictive models of high-pressure H2 solubility in brine (H2O+ NaCl) for underground hydrogen storage application. Int. J. Hydrogen Energy 45 (56), 32206–32220. doi:10.1016/j.ijhydene.2020.08.192
Chadwick, A., Arts, R., Bernstone, C., May, F., Thibeau, S., and Zweigel, P. (2008). Best practice for the storage of CO2 in saline aquifers-observations and guidelines from the SACS and CO2STORE projects. Nottingham, United Kingdom: British Geological Survey.
Chow, Y. F., Maitland, G. C., and Trusler, J. M. (2018). Interfacial tensions of (H2O+ H2) and (H2O+ CO2+ H2) systems at temperatures of (298–448) K and pressures up to 45 MPa. Fluid Phase Equilibria 475, 37–44. doi:10.1016/j.fluid.2018.07.022
Edlmann, K., Bensabat, J., Niemi, A., Haszeldine, R. S., and McDermott, C. I. (2016). Lessons learned from using expert elicitation to identify, assess and rank the potential leakage scenarios at the Heletz pilot CO2 injection site. Int. J. Greenh. Gas Control 49, 473–487. doi:10.1016/j.ijggc.2016.02.018
Einstein, A. (1906). Eine neue Bestimmung der Molekuldimensionen. Ann. Phys. 19 (1906), 289–306. doi:10.1002/andp.19063240204
Epelle, E. I., Obande, W., Udourioh, G. A., Afolabi, I. C., Desongu, K. S., Orivri, U., et al. (2022). Perspectives and prospects of underground hydrogen storage and natural hydrogen. Sustain. Energy & Fuels 6, 3324–3343. doi:10.1039/D2SE00618A
D. J. Evans, and R. A. Chadwick (Editors) (2009). Underground gas storage: Worldwide experiences and future development in the UK and Europe (Nottingham, United Kingdom: Geological Society of London).
Feldmann, F., Hagemann, B., Ganzer, L., and Panfilov, M. (2016). Numerical simulation of hydrodynamic and gas mixing processes in underground hydrogen storages. Environ. Earth Sci. 75 (16), 1165–1215. doi:10.1007/s12665-016-5948-z
Flesch, S., Pudlo, D., Albrecht, D., Jacob, A., and Enzmann, F. (2018). Hydrogen underground storage - petrographic and petrophysical variations in reservoir sandstones from laboratory experiments under simulated reservoir conditions. Int. J. Hydrogen Energy 43 (45), 20822–20835. doi:10.1016/j.ijhydene.2018.09.112
Foh, S. E. (1991). The use of inert gas as cushion gas in underground storage: Practical and economic issues (No. CONF-910240-1). Chicago: Institute of Gas Technology.
Foh, S., Novil, M., Rockar, E., and Randolph, P. (1979). Underground hydrogen storage. final report. [salt caverns, excavated caverns, aquifers and depleted fields] (No. BNL-51275). Upton, NY (USA): Brookhaven National Lab.
Gabrielli, P., Poluzzi, A., Kramer, G. J., Spiers, C., Mazzotti, M., and Gazzani, M. (2020). Seasonal energy storage for zero-emissions multi-energy systems via underground hydrogen storage. Renew. Sustain. Energy Rev. 121, 109629. doi:10.1016/j.rser.2019.109629
Gils, H. C., Gardian, H., and Schmugge, J. (2021). Interaction of hydrogen infrastructures with other sector coupling options towards a zero-emission energy system in Germany. Renew. Energy 180, 140–156. doi:10.1016/j.renene.2021.08.016
Hagemann, B., Rasoulzadeh, M., Panfilov, M., Ganzer, L., and Reitenbach, V. (2014). Hydrogenization of underground storage of natural gas-Impact of hydrogen on biochemical transformations of stored gas. ECMOR XIV-14th Eur. Conf. Math. Oil Recovery 2014, 1–11.
Han, J., Lee, M., Lee, W., Lee, Y., and Sung, W. (2016). Effect of gravity segregation on CO2 sequestration and oil production during CO2 flooding. Appl. Energy 161, 85–91. doi:10.1016/j.apenergy.2015.10.021
Heidug, W. (2013). Methods to assess geologic CO2 storage capacity: Status and best practice. Paris, France: IEA Publications. OECD/IEA 2013.
Heinemann, N., Alcalde, J., Miocic, J. M., Hangx, S. J., Kallmeyer, J., Ostertag-Henning, C., et al. (2021b). Enabling large-scale hydrogen storage in porous media–the scientific challenges. Energy & Environ. Sci. 14 (2), 853–864. doi:10.1039/d0ee03536j
Heinemann, N., Booth, M. G., Haszeldine, R. S., Wilkinson, M., Scafidi, J., and Edlmann, K. (2018). Hydrogen storage in porous geological formations–onshore play opportunities in the midland valley (Scotland, UK). Int. J. Hydrogen Energy 43 (45), 20861–20874. doi:10.1016/j.ijhydene.2018.09.149
Heinemann, N., Scafidi, J., Pickup, G., Thaysen, E. M., Hassanpouryouzband, A., Wilkinson, M., et al. (2021c). Hydrogen storage in saline aquifers: The role of cushion gas for injection and production. Int. J. Hydrogen Energy 46 (79), 39284–39296. doi:10.1016/j.ijhydene.2021.09.174
Heinemann, N., Scafidi, J., Pickup, G., Thaysen, E. M., Hassanpouryouzband, A., Wilkinson, M., et al. (2021a). Hydrogen storage in saline aquifers: The role of cushion gas for injection and production. Int. J. Hydrogen Energy 46 (79), 39284–39296. doi:10.1016/j.ijhydene.2021.09.174
Hematpur, H., Abdollahi, R., Rostami, S., Haghighi, M., and Blunt, M. J. (2023). Review of underground hydrogen storage: Concepts and challenges. Adv. Geo-Energy Res. 7 (2), 111–131. doi:10.46690/ager.2023.02.05
Hemme, C., and Van Berk, W. (2018). Hydrogeochemical modeling to identify potential risks of underground hydrogen storage in depleted gas fields. Appl. Sci. 1 (11), 2282. doi:10.3390/app8112282
Hernandez, D. D., and Gençer, E. (2021). Techno-economic analysis of balancing California’s power system on a seasonal basis: Hydrogen vs. lithium-ion batteries. Appl. Energy 300, 117314. doi:10.1016/j.apenergy.2021.117314
Hosseini, M., Fahimpour, J., Ali, M., Keshavarz, A., and Iglauer, S. (2022). H2− brine interfacial tension as a function of salinity, temperature, and pressure; implications for hydrogen geo-storage. J. Petroleum Sci. Eng. 213, 110441. doi:10.1016/j.petrol.2022.110441
Husarek, D., Schmugge, J., and Niessen, S. (2021). Hydrogen supply chain scenarios for the decarbonisation of a German multi-modal energy system. Int. J. Hydrogen Energy 46 (76), 38008–38025. doi:10.1016/j.ijhydene.2021.09.041
Ide, S. T., Jessen, K., and Orr, F. M. (2007). Storage of CO2 in saline aquifers: Effects of gravity, viscous, and capillary forces on amount and timing of trapping. Int. J. Greenh. gas control 1 (4), 481–491. doi:10.1016/s1750-5836(07)00091-6
Iglauer, S., Ali, M., and Keshavarz, A. (2021). Hydrogen wettability of sandstone reservoirs: Implications for hydrogen geo-storage. Geophys. Res. Lett. 48 (3), e2020GL090814. doi:10.1029/2020gl090814
Iglauer, S. (2022). Optimum geological storage depths for structural H2 geo-storage. J. Petroleum Sci. Eng. 212, 109498. doi:10.1016/j.petrol.2021.109498
Iordache, I., Schitea, D., Gheorghe, A. V., and Iordache, M. (2014). Hydrogen underground storage in Romania, potential directions of development, stakeholders and general aspects. Int. J. hydrogen energy 39 (21), 11071–11081. doi:10.1016/j.ijhydene.2014.05.067
Jamshidnezhad, M., Shen, C., Kool, P., Mojaddam Zadeh, A., and Rossen, W. R. (2010). Improving injectivity to fight gravity segregation in gas enhanced oil recovery. SPE J. 15 (01), 91–104. doi:10.2118/112375-pa
Jones, S. H., and Machsen, M. (1963). Joint meeting with institution of gas engineers. Storage of gas undergrand in aquifers, 18 march 1963. Proc. Institution Civ. Eng. 26 (2), 317–330. doi:10.1680/iicep.1963.10444
Kanaani, M., Sedaee, B., and Asadian-Pakfar, M. (2022). Role of cushion gas on underground hydrogen storage in depleted oil reservoirs. J. Energy Storage 45, 103783. doi:10.1016/j.est.2021.103783
Knopf, S., and May, F. (2017). Comparing methods for the estimation of CO2 storage capacity in saline aquifers in Germany: Regional aquifer based vs. structural trap-based assessments. Energy Procedia 114, 4710–4721. doi:10.1016/j.egypro.2017.03.1605
Lankof, L., and Tarkowski, R. (2020). Assessment of the potential for underground hydrogen storage in bedded salt formation. Int. J. hydrogen energy 45 (38), 19479–19492. doi:10.1016/j.ijhydene.2020.05.024
Lanz, A., Heffel, J., and Messer, C. (2001). Hydrogen fuel cell engines and related technologies (No. FTA-CA-26-7022-01.1). United States: Department of Transportation. Federal Transit Administration.
LBEG (2021). Jahresbericht "Erdöl und Erdgas in der Bundesrepublik Deutschland. https://www.lbeg.niedersachsen.de/erdoel-erdgas-jahresbericht/jahresbericht-erdoel-und-erdgas-in-der-bundesrepublik-deutschland-936.html (Accessed 02 22, 2023).
Leachman, J. W., Jacobsen, R. T., Penoncello, S. G., and Lemmon, E. W. (2009). Fundamental equations of state for parahydrogen, normal hydrogen, and orthohydrogen. J. Phys. Chem. Ref. Data 38 (3), 721–748. doi:10.1063/1.3160306
Lemmon, E. W., McLinden, M. O., and Friend, D. G. (2023). “Thermophysical properties of fluid systems,” in NIST chemistry WebBook, NIST standard reference database number 69. Editors P. J. Linstrom, and W. G. Mallard (Gaithersburg MD: National Institute of Standards and Technology), 20899. (retrieved January 20, 2023).
Li, D., Beyer, C., and Bauer, S. (2018). A unified phase equilibrium model for hydrogen solubility and solution density. Int. J. Hydrogen Energy 43 (1), 512–529. doi:10.1016/j.ijhydene.2017.07.228
Lord, A. S., Kobos, P. H., Borns, D. J., and Klise, G. T. (2011). A life cycle cost analysis framework for geologic storage of hydrogen: A user's tool (No. SAND2011-6221). Albuquerque, NM, and Livermore, CA (United States): Sandia National Laboratories.
Lux, B., Deac, G., Kiefer, C. P., Kleinschmitt, C., Bernath, C., Franke, K., et al. (2022). The role of hydrogen in a greenhouse gas-neutral energy supply system in Germany. Energy Convers. Manag. 270, 116188. doi:10.1016/j.enconman.2022.116188
Lysyy, M., Fernø, M., and Ersland, G. (2021). Seasonal hydrogen storage in a depleted oil and gas field. Int. J. Hydrogen Energy 46 (49), 25160–25174. doi:10.1016/j.ijhydene.2021.05.030
Marcogaz (2017). Guidance - injection of hydrogen/natural gas admixtures in underground gas storage (UGS). Available at: https://www.marcogaz.org/publications/.
May, F., Krull, P., and Gerling, P. (2004). CO2 storage scenarios in North Germany GESTCO project case studies. Hannover: Bundesanstalt fur Geowissenschaften und Rohstoffe.
Michalski, J., Bünger, U., Crotogino, F., Donadei, S., Schneider, G. S., Pregger, T., et al. (2017). Hydrogen generation by electrolysis and storage in salt caverns: Potentials, economics and systems aspects with regard to the German energy transition. Int. J. Hydrogen Energy 42 (19), 13427–13443. doi:10.1016/j.ijhydene.2017.02.102
Mouli-Castillo, J., Heinemann, N., and Edlmann, K. (2021). Mapping geological hydrogen storage capacity and regional heating demands: An applied UK case study. Appl. Energy 283, 116348. doi:10.1016/j.apenergy.2020.116348
Muhammed, N. S., Haq, B., Al Shehri, D., Al-Ahmed, A., Rahman, M. M., and Zaman, E. (2022). A review on underground hydrogen storage: Insight into geological sites, influencing factors and future outlook. Energy Rep. 8, 461–499. doi:10.1016/j.egyr.2021.12.002
Muhammed, N. S., Haq, M. B., Al Shehri, D. A., Al-Ahmed, A., Rahman, M. M., Zaman, E., et al. (2023). Hydrogen storage in depleted gas reservoirs: A comprehensive review. Fuel 337, 127032. doi:10.1016/j.fuel.2022.127032
Neuwirth, M., Fleiter, T., Manz, P., and Hofmann, R. (2022). The future potential hydrogen demand in energy-intensive industries - a site-specific approach applied to Germany. Energy Convers. Manag. 252, 115052. doi:10.1016/j.enconman.2021.115052
Osman, A. I., Mehta, N., Elgarahy, A. M., Hefny, M., Al-Hinai, A., Al-Muhtaseb, A. A. H., et al. (2021). Hydrogen production, storage, utilisation and environmental impacts: A review. Environ. Chem. Lett. 20, 153–188. doi:10.1007/s10311-021-01322-8
Pan, B., Yin, X., Ju, Y., and Iglauer, S. (2021a). Underground hydrogen storage: Influencing parameters and future outlook. Adv. Colloid Interface Sci. 294, 102473. doi:10.1016/j.cis.2021.102473
Panfilov, M. (2016). “Underground and pipeline hydrogen storage,” in Compendium of hydrogen energy (Sawston, United Kingdom: Woodhead Publishing), 91–115.
Paterson, L. (1983). The implications of fingering in underground hydrogen storage. Int. J. hydrogen energy 8 (1), 53–59. doi:10.1016/0360-3199(83)90035-6
Pawar, R. J., Bromhal, G. S., Carey, J. W., Foxall, W., Korre, A., Ringrose, P. S., et al. (2015). Recent advances in risk assessment and risk management of geologic CO2 storage. Int. J. Greenh. Gas Control 40, 292–311. doi:10.1016/j.ijggc.2015.06.014
Pfeiffer, W. T., and Bauer, S. (2015). Subsurface porous media hydrogen storage–scenario development and simulation. Energy Procedia 76, 565–572. doi:10.1016/j.egypro.2015.07.872
Rabinovich, A., and Cheng, K. B. (2020). Equilibrium gravity segregation in porous media with capillary heterogeneity. J. Fluid Mech. 890, A3. doi:10.1017/jfm.2020.133
Raza, A., Arif, M., Glatz, G., Mahmoud, M., Al Kobaisi, M., Alafnan, S., et al. (2022). A holistic overview of underground hydrogen storage: Influencing factors, current understanding, and outlook. Fuel 330, 125636. doi:10.1016/j.fuel.2022.125636
Reinhold, K., Müller, C., and Riesenberg, C. (2011). Informationssystem Speichergesteine für den Standort Deutschland - Synthese. Berlin/Hannover: Bundesanstalt für Geowissenschaften und Rohstoffe.
Reitenbach, V., Ganzer, L., Albrecht, D., and Hagemann, B. (2015). Influence of added hydrogen on underground gas storage: A review of key issues. Environ. Earth Sci. 73 (11), 6927–6937. doi:10.1007/s12665-015-4176-2
Rhouma, S. B., Smaï, F. F., Veloso, F. D. M. L., Masson, R., Broseta, D., Chiquet, P., et al. (2022). “Underground Hydrogen storage with CO2 cushion gas in aquifers: Which Equation-of-State?,” in Revitalizing old fields and energy transition in mature basins (Corrèze: HAL portal BRGM).
Rossen, W. R., Van Duijn, C. J., Nguyen, Q. P., Shen, C., and Vikingstad, A. K. (2010). Injection strategies to overcome gravity segregation in simultaneous gas and water injection into homogeneous reservoirs. SPE J. 15 (01), 76–90. doi:10.2118/99794-pa
Ruith, M., and Meiburg, E. (2000). Miscible rectilinear displacements with gravity override. Part 1. Homogeneous porous medium. J. Fluid Mech. 420, 225–257. doi:10.1017/s0022112000001543
Rutqvist, J., and Stephansson, O. (2003). The role of hydromechanical coupling in fractured rock engineering. Hydrogeol. J. 11 (1), 7–40. doi:10.1007/s10040-002-0241-5
Sáinz-García, A., Abarca, E., Rubí, V., and Grandia, F. (2017). Assessment of feasible strategies for seasonal underground hydrogen storage in a saline aquifer. Int. J. hydrogen energy 42 (26), 16657–16666. doi:10.1016/j.ijhydene.2017.05.076
Samsatli, S., and Samsatli, N. J. (2019). The role of renewable hydrogen and inter-seasonal storage in decarbonising heat – comprehensive optimisation of future renewable energy value chains. Appl. Energy 233-234, 854–893. doi:10.1016/j.apenergy.2018.09.159
Scafidi, J., Wilkinson, M., Gilfillan, S. M., Heinemann, N., and Haszeldine, R. S. (2021). A quantitative assessment of the hydrogen storage capacity of the UK continental shelf. Int. J. Hydrogen Energy 46 (12), 8629–8639. doi:10.1016/j.ijhydene.2020.12.106
Sedlacek, R. (1999a). “Underground gas storage in Europe and Central Asia,” in Study of the united nations economic commission for eurpe (UN/ECE) (Hamburg: Oil, gas).
Sens, L., Piguel, Y., Neuling, U., Timmerberg, S., Wilbrand, K., and Kaltschmitt, M. (2022). Cost minimized hydrogen from solar and wind – production and supply in the European catchment area. Energy Convers. Manag. 265, 115742. doi:10.1016/j.enconman.2022.115742
Setzmann, U., and Wagner, W. (1991). A new equation of state and tables of thermodynamic properties for methane covering the range from the melting line to 625 K at pressures up to 100 MPa. J. Phys. Chem. Ref. Data 20 (6), 1061–1155. doi:10.1063/1.555898
Simón, J., Férriz, A. M., and Correas, L. C. (2015). HyUnder–hydrogen underground storage at large scale: Case study Spain. Energy procedia 73, 136–144. doi:10.1016/j.egypro.2015.07.661
Šmigáň, P., Greksak, M., Kozánková, J., Buzek, F., Onderka, V., and Wolf, I. (1990). Methanogenic bacteria as a key factor involved in changes of town gas stored in an underground reservoir. FEMS Microbiol. Ecol. 6 (3), 221–224. doi:10.1111/j.1574-6968.1990.tb03944.x
Sørensen, B. (2007). “Underground hydrogen storage in geological formations, and comparison with other storage solutions,” in Hydrogen power theoretical and engineering international symposium VII (Merida, Mexico: CDROM).
Span, R., and Wagner, W. (1996). A new equation of state for carbon dioxide covering the fluid region from the triple-point temperature to 1100 K at pressures up to 800 MPa. J. Phys. Chem. Ref. Data 25 (6), 1509–1596. doi:10.1063/1.555991
Stober, I., and Bucher, K. (2021). “Hydrothermal systems, geothermal doublets,” in Geothermal energy (Cham: Springer), 155–203.
Suchi, E., Dittmann, J., Knopf, S., Müller, C., and Schulz, R. (2014). Geothermal Atlas to visualise potential conflicts of interest between CO2 storage (CCS) and deep geothermal energy in Germany. Z. Dtsch. Ges. für Geowiss. 165, 439–453. doi:10.1127/1860-1804/2014/0070
Tarkowski, R., and Czapowski, G. (2018). Salt domes in Poland–potential sites for hydrogen storage in caverns. Int. J. Hydrogen Energy 43 (46), 21414–21427. doi:10.1016/j.ijhydene.2018.09.212
Tarkowski, R., Uliasz-Misiak, B., and Tarkowski, P. (2021). Storage of hydrogen, natural gas, and carbon dioxide–Geological and legal conditions. Int. J. Hydrogen Energy 46 (38), 20010–20022. doi:10.1016/j.ijhydene.2021.03.131
Tarkowski, R. (2019). Underground hydrogen storage: Characteristics and prospects. Renew. Sustain. Energy Rev. 105, 86–94. doi:10.1016/j.rser.2019.01.051
M. R. Tek (Editor) (1989). Underground storage of natural gas: Theory and practice (Berlin, Germany: Springer Science & Business Media).
Truche, L., Berger, G., Destrigneville, C., Guillaume, D., and Giffaut, E. (2010). Kinetics of pyrite to pyrrhotite reduction by hydrogen in calcite buffered solutions between 90 and 180 C: Implications for nuclear waste disposal. Geochimica Cosmochimica Acta 74 (10), 2894–2914. doi:10.1016/j.gca.2010.02.027
Truche, L., Jodin-Caumon, M. C., Lerouge, C., Berger, G., Mosser-Ruck, R., Giffaut, E., et al. (2013). Sulphide mineral reactions in clay-rich rock induced by high hydrogen pressure. Application to disturbed or natural settings up to 250 C and 30 bar. Chem. Geol. 351, 217–228. doi:10.1016/j.chemgeo.2013.05.025
Wang, A., Jens, J., Mavins, D., Moultak, M., Schimmel, M., van der Leun, K., et al. (2021). European Hydrogen Backbone: Analysing future demand, supply, and transport of hydrogen. Utrecht, Netherlands: Creos, DESFA, Elering, Enagás, Energinet, Eustream, FGSZ, Fluxys Belgium, Gas Connect.
Wang, A., van der Leun, K., Peters, D., and Buseman, M. (2020). European Hydrogen Backbone: How a dedicated hydrogen infrastructure can be created. Tech. Rep. European Hydrogen Backbone Initiative.
Watts, N. L. (1987). Theoretical aspects of cap-rock and fault seals for single-and two-phase hydrocarbon columns. Mar. Petroleum Geol. 4 (4), 274–307. doi:10.1016/0264-8172(87)90008-0
Welder, L., Ryberg, D. S., Kotzur, L., Grube, T., Robinius, M., and Stolten, D. (2018). Spatio-temporal optimization of a future energy system for power-to-hydrogen applications in Germany. Energy 158, 1130–1149. doi:10.1016/j.energy.2018.05.059
White, J. A., and Foxall, W. (2016). Assessing induced seismicity risk at CO2 storage projects: Recent progress and remaining challenges. Int. J. Greenh. Gas Control 49, 413–424. doi:10.1016/j.ijggc.2016.03.021
Wiebe, R., and Gaddy, V. L. (1934). The solubility of hydrogen in water at 0, 50, 75 and 100 from 25 to 1000 atmospheres. J. Am. Chem. Soc. 56 (1), 76–79. doi:10.1021/ja01316a022
Wilke, C. R., and Chang, P. (1955). Correlation of diffusion coefficients in dilute solutions. AIChE J. 1 (2), 264–270. doi:10.1002/aic.690010222
Williams, J. D., Williamson, J. P., Parkes, D., Evans, D. J., Kirk, K. L., Sunny, N., et al. (2022). Does the United Kingdom have sufficient geological storage capacity to support a hydrogen economy? Estimating the salt cavern storage potential of bedded halite formations. J. Energy Storage 53, 105109. doi:10.1016/j.est.2022.105109
Yekta, A. E., Pichavant, M., and Audigane, P. (2018). Evaluation of geochemical reactivity of hydrogen in sandstone: Application to geological storage. Appl. Geochem. 95, 182–194. doi:10.1016/j.apgeochem.2018.05.021
Zivar, D., Kumar, S., and Foroozesh, J. (2021). Underground hydrogen storage: A comprehensive review. Int. J. Hydrogen Energy 46 (45), 23436–23462. doi:10.1016/j.ijhydene.2020.08.138
Keywords: underground hydrogen storage, UHS, porous media, energy transition, UHS criteria, UHS storage potential, saline aquifers
Citation: Alms K, Ahrens B, Graf M and Nehler M (2023) Linking geological and infrastructural requirements for large-scale underground hydrogen storage in Germany. Front. Energy Res. 11:1172003. doi: 10.3389/fenrg.2023.1172003
Received: 22 February 2023; Accepted: 04 May 2023;
Published: 02 June 2023.
Edited by:
Henrietta Wakuna Langmi, University of Pretoria, South AfricaReviewed by:
Bashirul Haq, King Fahd University of Petroleum and Minerals, Saudi ArabiaWei Liu, Chongqing University, China
Copyright © 2023 Alms, Ahrens, Graf and Nehler. This is an open-access article distributed under the terms of the Creative Commons Attribution License (CC BY). The use, distribution or reproduction in other forums is permitted, provided the original author(s) and the copyright owner(s) are credited and that the original publication in this journal is cited, in accordance with accepted academic practice. No use, distribution or reproduction is permitted which does not comply with these terms.
*Correspondence: Katharina Alms, a2F0aGFyaW5hLmFsbXNAaWVnLmZyYXVuaG9mZXIuZGU=