- 1Department of Chemical Engineering, Imperial College London, London, United Kingdom
- 2Lawrence Livermore National Laboratory, Livermore, CA, United States
- 3Surface Measurement Systems, London, United Kingdom
Expanding populations and growing economies result in higher energy needs. Meeting this increasing demand, while lowering carbon emissions, calls for a broad energy mix and commercial deployment of solutions like carbon capture and carbon removal technologies. The scale-up of these solutions is partially hindered by the lack of materials-related information, particularly in the case of solid adsorption-based carbon capture technologies. Furthermore, experimental measurement parameters used and how data is presented lack uniformity, which makes material comparisons extremely difficult. This review examines the current state of solid sorbent characterization for carbon capture, exploring physical and chemical properties, performance parameters, and process indicators. Adsorbent performance parameters demonstrate to be the crucial link between intrinsic material properties and the overall adsorption process effectiveness and therefore are the focus of this work. This paper outlines the relevant techniques used to measure Key Performance Indicators (KPIs) related to adsorption performance such as CO2 adsorption capacity, selectivity, kinetics, ease of regeneration, stability, adsorbent cost, and environmental impact. Additionally, this study highlights the relevant experimental conditions for diluted versus concentrated CO2 streams. Lastly, efforts in harmonizing experimental data sets are considered, and an outlook on solid sorbent characterization for carbon capture processes is presented. Overall, the aim of this work is to provide the reader a critical understanding of KPIs from atomic to process scale, highlighting the importance of experimental data throughout.
1 Introduction
The continuously increasing concentration of CO2 in the atmosphere due to anthropogenic emissions has caused an estimated 0.8°C–1.2°C rise in average global temperature (Siegelman et al., 2021). These warming levels can have severe effects on our planet and its inhabitants, including extreme weather events, sea-level rise, species extinction and risks to human health (IPCC, 2018). To avoid these catastrophic events, carbon capture, utilization, and storage (CCUS) has been proposed as one of the potential solutions for decarbonizing sectors like energy, chemicals, construction, and other industries as well as for pulling CO2 out of the atmosphere (i.e., carbon dioxide removal). Despite its important role in mitigating climate change, CCUS commercial deployment has been slow and its impact on global CO2 emissions is currently limited. As of 2021, global CO2 capture capacity from power and industrial facilities reached 40 Mt, representing only 0.12% of the annual global energy-related CO2 emissions (IEA, 2020). According to the International Energy Agency (IEA), CCUS capacity needs to increase to 7,600 MtCO2/year to achieve net zero by 2050 (IEA, 2021).
CCUS involves the capture of CO2 from industrial processes and its subsequent transport via road, rail, or sea. CO2 can be used to produce valuable products such as chemicals, fuels, and building materials, or directly stored in carbon sinks. Although economically attractive, the environmental impact of CO2 utilization must be evaluated carefully to avoid potential net CO2 emission increase. Carbon capture systems can be classified into post-combustion, pre-combustion, and oxy-combustion; with post-combustion capture (PCC) systems being the most mature and adopted worldwide due to their retrofit ability (Bui et al., 2018) (See Figure 1). Each of these systems can employ different technologies for gas separation such as chemical absorption, solid adsorption, or membrane separation (Bui et al., 2018). More recently, other CO2 removal systems such as direct air capture (DAC) and bioenergy with carbon capture and storage (BECCS) have been proposed to help compensate for emissions from hard to decarbonize sectors such as aviation (Bui et al., 2018; Becattini et al., 2021).
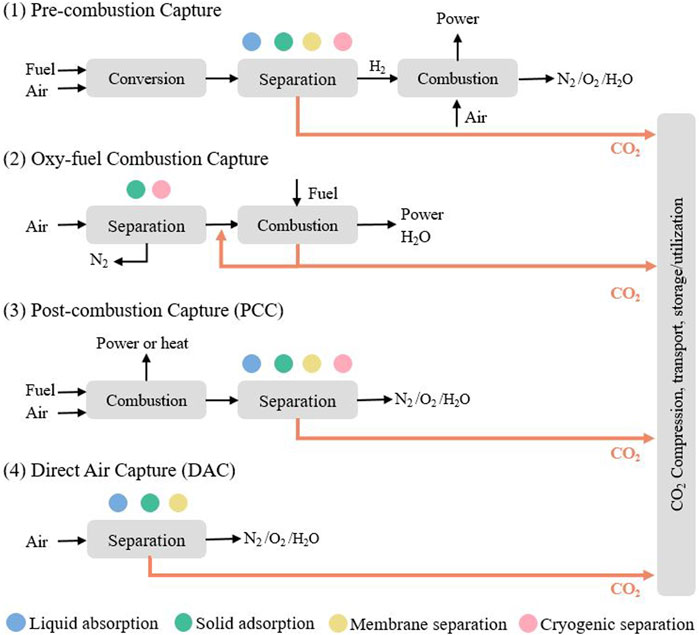
FIGURE 1. Carbon capture systems including pre-combustion, oxy-fuel combustion, post-combustion, and direct air capture with possible separation technologies.
While there is a clear need to ramp up the commercial implementation of CCUS, there are various associated challenges, such as high costs, lack of governmental policies and uncertain public acceptance (Jones et al., 2017). Currently, liquid absorption is considered the most mature and economic technology for PCC (Bui et al., 2018). However, a promising alternative is gas phase adsorption with solid sorbents, which has showed some advantages over liquid absorption. These include energy savings, no use of water make-up streams, and the flexibility to capture CO2 using different regeneration modes and process configurations (Bui et al., 2018; Kiani et al., 2020; Wang and Song, 2020; Liu et al., 2021).
Evaluating solid sorbents poses a significant challenge due to the vast array of materials and potential process configurations involved. The pursuit of the ideal solid sorbent for various carbon capture applications has been a primary focus of research. However, transitioning a material from the laboratory to pilot scale requires a thorough grasp of its adsorbent properties, adsorption performance, and process efficiency. This progression demands a combination of experimental and computational efforts, which can be both costly and time-consuming, resulting in incomplete or incomparable data sets across different materials. In this study, we aim to shed light on this pipeline highlighting the relevant Key Performance Indicators (KPIs) at each stage, to achieve a comprehensive material characterization, underscoring the significance of precise and reproducible experimental data.
1.1 Adsorption technology in carbon capture
At present, adsorption technology for CO2 capture is at pre-commercial demonstration with pilot projects including CO2 capture from power generation, iron, steel, chemical and cement industries, as well as DAC (Bui et al., 2018; IEA, 2020; Lin et al., 2021). In adsorption-based carbon capture processes, CO2 concentrates on a solid surface (e.g., activated carbon, alumina, metallic oxides, or zeolites). Adsorption can occur through two different mechanisms, physisorption or chemisorption. Physisorption involves non-bonding interactions between adsorbent and adsorbate, while chemisorption involves the formation of weak chemical bonds. After adsorption, molecules are released or desorbed by a change in temperature (temperature swing adsorption [TSA]), pressure (pressure swing adsorption [PSA], vacuum swing adsorption [VSA]) composition (moisture, pH swing adsorption) or combinations thereof. The recovery of CO2 is related to the binding strength of adsorption, defined by the adsorption enthalpy (
Adsorbents for CO2 capture can be classified into four main groups: (1) organic, (2) inorganic, (3) Metal Organic Frameworks (MOFs)/Porous Coordination Polymers (PCPs) and (4) hybrid adsorbents (Sreenivasulu et al., 2015; Liu et al., 2021). For specific material performance data, we draw the attention of the reader to several studies on the recent advances in solid sorbents for PCC and DAC systems (Samanta et al., 2012; Lee and Park, 2015; Sreenivasulu et al., 2015; Ünveren et al., 2017; Nie et al., 2018; Webley and Danaci, 2019; Liu et al., 2021; Siegelman et al., 2021), (Shi et al., 2020; Shi et al., 2022; Wu et al., 2022; Low et al., 2023; Panda et al., 2023). Alongside adsorbent development, their practical implementation has been assessed from an engineering and techno-economic perspective (Bui et al., 2018; Hu et al., 2019; Yang et al., 2019; Danaci et al., 2020; Liu et al., 2021; Raganati et al., 2021), (Azarabadi and Lackner, 2019; Sinha and Realff, 2019; Danaci et al., 2021).
These studies highlight the increasing variety of solid sorbents for carbon capture, and the prevalent use of amine-functionalized adsorbents that deliver superior CO2 absorption and selectivity. Additionally, a shift in lab-scale material synthesis has been observed, moving away from powders towards monoliths, pellets, and structured adsorbents which are better suited for large-scale processes. While analyzing performance data of specific materials is beyond the scope of this work, there is unanimous agreement among the cited studies about the importance of comprehensive material characterization. However, discourse on its execution and measurement remain scarce, and it is precisely this gap the current work aims to fill.
1.2 Point source capture
Refers to the capture of CO2 from flue gas or other concentrated industrial waste gas. Table 1 shows the composition of CO2-containing mixtures in different industries, together with their respective temperature and pressure conditions; the composition of air is included for reference.

TABLE 1. Flue gas composition and conditions for different industries, air composition is included for reference.
1.3 Direct air capture (DAC)
Direct air capture (DAC) is the capture of CO2 from ambient air, a process that has gained attention as a negative emissions technology for net CO2 removal. Nevertheless, DAC faces technical and economic limitations due to the exceptionally low concentration of CO2 in ambient air (see Table 1) which is ∼200 times more dilute than the flue gas of a gas-fired power plant, and thus requires high gas flows and highly specific adsorbents. Cost estimates for DAC are about an order of magnitude greater than point source capture. (Sinha and Realff, 2019; Erans et al., 2022; Herzog, 2022). Despite its high cost, DAC could be used to mitigate CO2 emissions of processes where the addition of a capture unit is not feasible due physical/space constraints or where mobile emissions are involved (Sendi et al., 2022).
1.4 Key performance indicators (KPIs)
To effectively design, evaluate and compare CO2 adsorbents for point source capture or DAC, it is important to understand the Key Performance Indicators (KPIs) relevant to each carbon capture process. For simplicity, throughout this work, KPIs are classified into three main groups (1) intrinsic material properties, (2) adsorbent performance parameters and (3) process performance indicators (see Figure 2). Intrinsic material properties refer to the basic characterization parameters that are typically measured to understand an adsorbents physical and chemical composition, such as density, BET area, porosity, etc. On the other hand, adsorbent performance parameters are the metrics used to measure adsorbent efficiency under certain process conditions, they typically include adsorption capacity, selectivity, and kinetics. Lastly, process performance indicators include metrics like recovery, product purity and energy consumption. These can be optimized to achieve a more efficient and cost-effective carbon capture process.
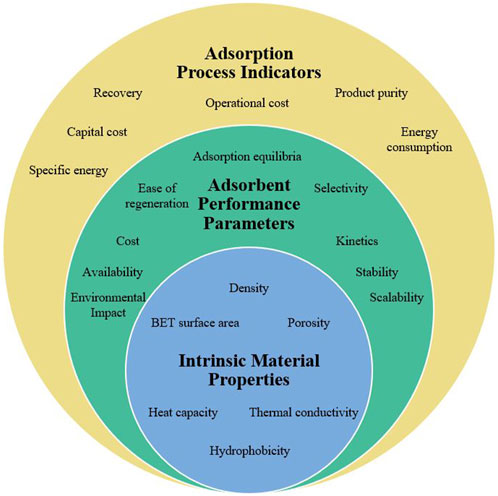
FIGURE 2. Classification of Key Performance Indicators (KPIs) for a carbon capture adsorption process.
The intrinsic material properties and related characterization techniques are well established. For some commercial materials like activated carbon (AC) its physical and chemical characterization has been standardized by the American Society for Testing and Materials (ASTM) (Bläker et al., 2019). Similarly, process performance indicators such as purity and recovery are typically set targets for a separation process and have been mathematically described for TSA/PSA processes (Rajagopalan et al., 2016). On the other hand, some adsorbent performance parameters are not yet standardized, and can be measured with a wide range of experimental techniques at varying conditions. Therefore, adsorption performance data for solid sorbents is often not consistent and difficult to compare. This issue has been raised both by the carbon capture and adsorption communities. For instance, Espinal et al., underline insufficient materials-related measurements, standards and data on solid sorbents which is critical for understanding and evaluating the overall performance of an adsorption-based carbon capture process (Espinal et al., 2013). Additionally, the National Institute of Standards and Technology (NIST) and the Council for Chemical Research (CCR) have highlighted the need to enhance experimental capability to better understand adsorption rate, diffusional mechanisms, and competitive adsorption capacity, all of which are key parameters regarding adsorbent performance (NIST, 2015; Walton, 2019).
This work comprehensively reviews advanced experimental measures of adsorbent performance parameters like adsorption capacity, selectivity, kinetics, and stability. It evaluates each technique’s advantages and limitations, and considers the suitable conditions for point source capture versus DAC. Furthermore, it provides a critical discussion on the interrelationship among the different KPIs, emphasizing on data reproducibility and standardization. Lastly, it concludes with a forward-looking commentary on the experimental characterization of solid sorbents for carbon capture. While a detailed examination of computational methods is not included, readers are directed to the work by Farmahini et al., for an in-depth review of computational approaches (Farmahini et al., 2021).
To present the information in an orderly manner, this paper guides the reader through the usual experimental pathway followed to assess a newly prepared CO2 capture material from laboratory to pilot scale (see Figure 3). It is important to point out that each material is different, and its analysis may vary depending on its specific characteristics. Experimental characterization is not a one-size-fits-all procedure and is subject to instrumentation and time availability.
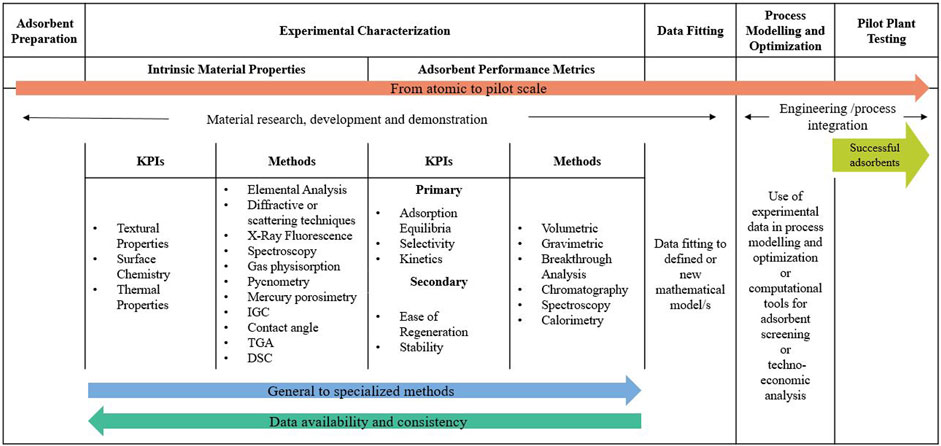
FIGURE 3. Experimental pathway to assess newly prepared CO2 capture materials from atomic to pilot scale.
2 Intrinsic material properties
The intrinsic material properties of an adsorbent describe the nature of the material outside the context of an adsorption process (i.e., physico-chemical properties). Typically, the first stage in material characterization is identification (i.e., to determine whether the desired material has been produced), also providing insight into the structure of the adsorbent. For crystalline or well-ordered materials this can be accomplished using diffractive ion (Galarneau et al., 2003; Papageorgiou et al., 2013; Zhou et al., 2018; Middelkoop et al., 2019) or scattering techniques (Kruk and Cao, 2007; Gu et al., 2022). Materials may also be investigated to probe chemical species or bonds present using elemental analysis (Geng et al., 2016; Wang and Okubayashi, 2019; Lee et al., 2022), X-ray fluorescence (Somerset et al., 2004; Bao et al., 2022), or spectroscopy (Meador et al., 2015; Wang and Okubayashi, 2019; Hadjiivanov et al., 2021).
2.1 Textural properties
Once the material’s composition has been confirmed, the determination of its surface area and pore morphology is typically a priority. A suite of techniques exist to measure BET surface area and pore morphology (Schlumberger and Thommes, 2021), the most common being physisorption of gases such as N2, Ar, or CO2. A report published by the International Union of Pure and Applied Chemistry (IUPAC) details the methodology and best practices for characterizing porous solids via gas physisorption, from sample preparation to data interpretation (Thommes et al., 2015). A range of resources are available on this topic, and the reader is directed to them for additional details (Rouquerol et al., 2007; Ambroz et al., 2018; Bardestani et al., 2019).
The textural and geometric properties of porous materials can also be evaluated computationally (Nguyen et al., 2008; Coudert and Fuchs, 2016). Quantitative differences between simulations and experimental data do exist in literature (Garberoglio et al., 2005; Chowdhury et al., 2009), due to factors such as the measurement technique(s) used and the associated sample activation procedure (Thommes et al., 2015; Coudert and Fuchs, 2016). Textural properties such as crystal size and shape (Zhang et al., 2014; Hobday et al., 2021), and the presence of defects (Liang et al., 2018) within the material can be difficult to account for in computational predictions. Nevertheless, a combination of both is required to obtain accurate data. For example, obtaining information about the pore structure in materials that exhibit an interconnected pore network of different pore sizes including micro-, meso- and macropores (e.g., hierarchically ordered zeolites) can be experimentally challenging. Here, statistical mechanical models such as NLDFT and molecular simulation-based methods can be coupled with gas physisorption to obtain a comprehensive pore size analysis (Schlumberger and Thommes, 2021). Recently, dual-gas analysis techniques have also been proposed to enhance the resolution and accuracy of pore-size distribution analyses (Jagiello et al., 2019; Blankenship et al., 2022; Jagiello and Kenvin, 2022).
For applications in which the adsorbent bed’s structure is a concern, when using monoliths or pellets, quantifying the total porosity and/or packing density of the material during operation is important. Studies on monolithic MOF’s have used bulk density to highlight high volume-relative properties compared to their powder equivalents (Tian et al., 2015; Tian et al., 2018; Hunter-Sellars et al., 2020a; Madden et al., 2022). Bulk and packing densities, and subsequent void fractions, may be determined through experimental means, such as envelopment (pycnometry) (Benyahia and O'Neill, 2005; Hunter-Sellars et al., 2020a) or mercury porosimetry (Liu et al., 2018). Furthermore, the mechanical stability of adsorbents can be measured by compression (Lawson et al., 2020), or by tensile strength (Bazer-Bachi et al., 2014). Lastly, determination of the geometry and form factor of the adsorbent, which can strongly influence the subsequent bed’s mass and heat transfer, may be determined using a variety of techniques including light scattering (Kim et al., 2012) and microscopy (Lu et al., 2022).
2.2 Surface chemistry and chemical functionality
Alongside surface area and pore morphology, surface chemistry can strongly influence CO2 adsorption capacity, interaction strength, and selectivity. Examples of rational tuning of surfaces include enhancing hydrophobicity (Piscopo and Loebbecke, 2020; Sáenz Cavazos et al., 2021) and amine-functionalization (Vilarrasa-García et al., 2015; Ünveren et al., 2017; Zhang and Du, 2022). The dispersive and specific chemical contributions to surface energy, and by extension a metric of hydrophobicity, can be determined by conducting liquid contact angle (Kozbial et al., 2014) or inverse gas chromatography measurements (Hamieh et al., 2020) using several fluid probes with different chemistries.
For amine functionalized materials, distinguishing between primary, secondary, and tertiary amines, each of which forms different byproducts during reactions with CO2, may be done using NMR spectroscopy (Moschetta et al., 2015; Shimon et al., 2018). Finally, the total loading of amine within a composite material, such as a mesoporous alumina or silica, can be determined using thermogravimetric burn-out procedures (Sujan et al., 2019), provided the thermal stability of the amine-containing compound is significantly lower than the support.
2.3 Thermal properties
Adsorbent stability, including thermal stability is key to determining adsorbent drying and regeneration conditions. Adsorbent stability is a wider topic and is further explored later in this report (see Section 3.5). Thermogravimetric analysis (TGA) is typically used to analyze mass loss events as a function of temperature and/or gas environment to help determine the thermal stability of a material. Regarding some amine-functionalized adsorbents, it is important to highlight that the thermal stability of physically attached amines (e.g., via wet impregnation) will strongly depend on their volatility. This volatility is related to their chemistry (Zhang et al., 2012; Liu et al., 2013), molecular weight (Heydari-Gorji and Sayari, 2012; Liu et al., 2013), and their dispersion within the pores or external surface of a support (Son et al., 2008).
Differential scanning calorimetry (DSC), with or without TGA can provide information about the specific heat capacity of the adsorbent material (Mu and Walton, 2011; Querejeta et al., 2019). A material’s heat transfer properties can be measured in a multitude of ways, typically involving monitoring the temperature profile of a fixed volume of sample during exposure to a heating element (Menard et al., 2007; Wu et al., 2021). For samples with low thermal conductivity, laser flash analysis is also considered a useful technique (Wang et al., 2018).
3 Adsorption performance parameters
Following initial material characterization and insight into inherent material properties, adsorbents typically undergo a series of tests to evaluate key adsorption performance metrics such as CO2 uptake, selectivity, and kinetics, labeled as primary adsorption performance parameters (see Figure 3). If a material demonstrates potential through these primary KPIs, secondary performance parameters like ease of regeneration, stability, cost and environmental impact are then assessed. This classification does not diminish the importance of secondary KPIs; they are simply evaluated after the primary ones due to the latter’s ‘quicker’ measurement process.
3.1 CO2 adsorption capacity
Refers to the amount of CO2 retained by an adsorbent at a single relevant concentration and temperature, at thermodynamic equilibrium (Raganati et al., 2021). It is sometimes referred to as total capacity (q). The CO2 capacity can be reported per mass (gravimetric capacity), volume (volumetric capacity) or sometimes as molar basis of adsorbent material. It is important to note that the volumetric capacity (and to a lesser extent, the gravimetric one) is influenced by the density and structure of the material (e.g. in the case of monoliths) (Chanut et al., 2016; Bingre et al., 2018; Iacomi et al., 2019).
Working adsorption capacity (WC) is a more process-relevant metric derived from q (Mason et al., 2011; Elfving et al., 2017; Raganati et al., 2020). WC refers to the amount of CO2 captured in an adsorption/desorption cycle, defined as the difference between two capacities corresponding to the adsorption and desorption conditions.
Equilibrium adsorption capacity is reported more frequently than WC, though the latter is better suited for adsorbent comparison (Wu et al., 2022; Low et al., 2023). Ideally, comprehensive screening in a large pressure and temperature range should be performed. This is a challenging prospect, as often, researchers lack the time, instrumentation, or sufficient material quantity to report extensive datasets. Advances in instrument automation and parallelization, alongside modeling approaches, could help ease this burden. Several simple computational tools have been proposed to minimize the burden of acquiring large sets of experimental data, expediting the screening of adsorbents for CO2 capture (Maring and Webley, 2013; Subramanian Balashankar et al., 2019; Danaci et al., 2020; Pai et al., 2020).
Single-component isotherms are one of the most ubiquitous methods of probing an adsorbent’s CO2 uptake by measuring equilibrium capacity as a function of adsorbate concentration at a fixed temperature. The CO2 concentration range will dictate the applicability for a particular process (see Table 1). For materials proposed for point source capture, concentrations can range between 5%–33% CO2 by volume, depending on the industrial process, while materials for DAC must be assessed at concentrations near 400 ppm to match ambient CO2 levels. Alongside adsorption, the desorption and subsequent hysteresis can provide information regarding the reversibility of the process or the presence of mass transfer limitations (Ravikovitch and Neimark, 2005; Sircar et al., 2011), both of which will impact material regeneration. Hysteresis can also be used to probe phenomena such as structural changes during the sorption process (Culp et al., 2008; Wu et al., 2016; Rahman et al., 2020), and to obtain information about the materials pore morphology (Sing and Williams, 2004).
There are several experimental techniques that can be used to measure single-component gas adsorption, here we discuss the most used in carbon capture studies, including volumetric and gravimetric techniques, breakthrough analysis and desorption methods. Table 2 includes the advantages, disadvantages, and limitations of the gas sorption techniques included in this work. It should be noted that simple metrics such as total CO2 capacity, and pure component WC, are not sufficient to guarantee success in an adsorption process (Maring and Webley, 2013; Rajagopalan et al., 2016; Danaci et al., 2020). These metrics should be used as decision criteria to undertake further detailed experimental work.
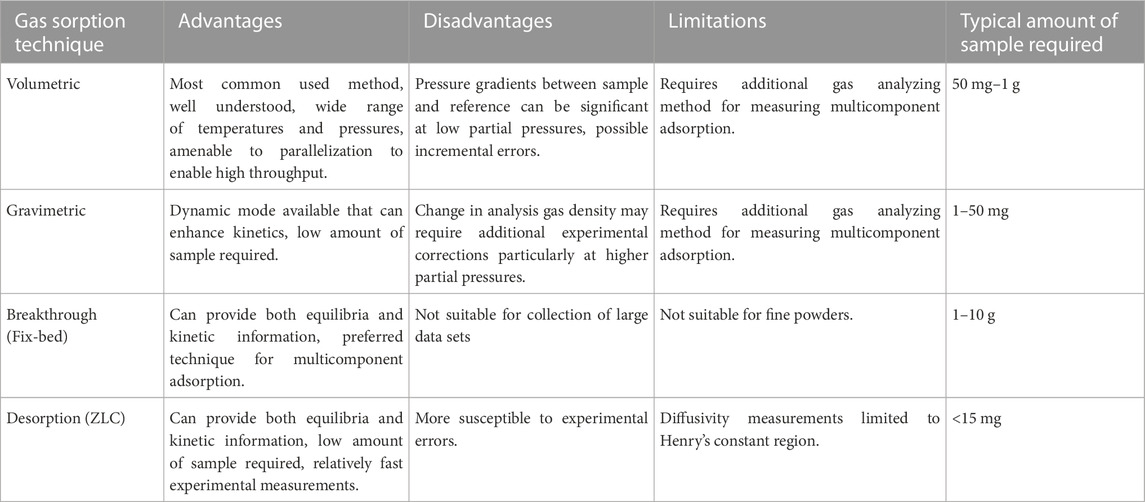
TABLE 2. Gas sorption techniques comparison table including advantages, disadvantages, and limitations.
3.1.1 Volumetric methods
Volumetry relies on an indirect measurement of amount adsorbed, through knowledge of state variables in the system such as pressure, temperature, and volume before and after adsorption occurs. Practically, this is achieved through expansion of gas from a calibrated reference volume to a second volume containing the sample. Pressure is monitored throughout the experiment, and appropriate equations of state are used to calculate the changes in the gas phase quantities, which correspond to the net or excess amount adsorbed if the cell volume or total accessible volume are known, respectively, (Mohammad et al., 2009). The amount of material commonly used for volumetric experiments will depend on its CO2 uptake, but also on the size of the reference and cell volumes, and accuracy of the pressure transducers. For most commercial instruments, amounts between 50 mg and 1 g are common (Mason et al., 2015). Volumetry is the most common method for probing the applicability of an adsorbent to carbon capture. In a recent review investigating sorbents for DAC, 47 out of 53 CO2 isotherms on different reported materials were recorded using volumetric instrumentation (Low et al., 2023). Notably, for higher pressures volumetry was nearly as common as gravimetry (7 out of 13 isotherms) in an inter-laboratory study (Nguyen et al., 2018).
As pressure is the measured variable, the accuracy of pressure transmitters is paramount in volumetry, particularly for the low concentration region of 400 ppm CO2, corresponding to a pressure of 40.5 Pa or 0.3 Torr. In this regime kinetics may be slow, and care should be taken for each point to fully reach equilibrium. Leak checks are particularly important in these conditions when studying under vacuum. Moreover, pressure gradients between the sample and reference volume may start to become significant at lower pressures when there is a large difference in temperature between the two volumes (e.g., during isotherm measurement at 77 K), requiring the use of thermal transpiration corrections (Takaishi and Sensui, 1963). A second notable aspect of volumetry is that it is an incremental measurement (each measured point is a delta from the previous). Regardless of the CO2 concentration, significant compounding of errors can occur if many isotherm points are recorded sequentially (Mohammad et al., 2009). In typical volumetry, a single variable is monitored, which precludes the measurement of multicomponent adsorption. Coupling with an analytical method capable of sampling the gas phase such as gas chromatography (GC) or mass spectroscopy (MS) is required to complete the mass balance and determine the composition of each adsorbed species and measure multi-component adsorption (see Section 3.2.1).
Volumetric techniques are highly amenable to parallelization by using a common dosing volume. A parallel system with six individual sample cells was used by Wiersum et al., to record CO2 and N2 isotherms on several standard materials to develop a KPI suited to post-combustion conditions (Wiersum et al., 2013). Bae et al. used a more complex parallelization setup to investigate up to 28 samples of zeolites for post-combustion CO2 capture, by recording single component CO2 and N2 isotherms at 3 temperatures, totaling 35 isotherms in 3 days. Most commercial instrumentation can be purchased with up to three sample ports (Bae et al., 2013).
3.1.2 Gravimetric methods
Together with volumetric techniques, gravimetric methods are among the most used to measure pure component adsorption isotherms (Low et al., 2023). Gravimetric instruments measure the net force resulting from changes in sample weight (Wang et al., 2021). A well-known benefit of gravimetric methods is that they typically require a low amount of sample: as little as 1 mg can be used to obtain reliable results for single component measurements. Furthermore, they can be used in a dynamic mode (Thompson and Zones, 2020) and can operate at either atmospheric pressure or under vacuum. Their dynamic operation mode entails a continuous flow of gas over the adsorbent, which significantly enhances the kinetics of adsorption, by (i) maintaining a constant concentration of CO2 around the sample and (ii) overcoming some diffusion barriers through forced convection. It also is not subject to cumulative errors that can occur for volumetric approaches.
When using gravimetric instruments to measure gas adsorption, regardless of the application, it is important to account for secondary contribution to the force balance from buoyancy and drag (Wang et al., 2021). Nevertheless, for carbon capture, when studying high-capacity physi- or chemisorbents utilized in DAC, sample weight changes will likely dominate. Whereas for high pressure applications (i.e., point source capture), the change in analysis gas density may require additional experimental corrections. Given the different microbalance configurations such as single arm (often magnetically suspended), or dual arms either symmetrical or asymmetrical. Correct accounting of force contributions for each configuration is important (Wang et al., 2021). Like vacuum volumetry, in vacuum gravimetric instruments, it is key to ensure there are no leaks when performing each experiment and that the temperature is accurately controlled. Furthermore, at high bulk fluid densities the accurate determination of the sample volume becomes critical (Pini, 2014; Brandani et al., 2016; Nguyen et al., 2019).
Gravimetric measurements can also be performed with adapted TGA systems and results have been reported in several CO2 screening studies (Plaza et al., 2008; Choi et al., 2012; Ojwang et al., 2018). While these can be straightforward to record single-point uptakes in a carrier gas, measuring complete, pure component isotherms generally requires the use of dedicated instruments. Commercial gravimetric instruments are available from different suppliers and like volumetric instruments, they require an additional gas detector to measure true multi-component adsorption (see Section 3.2.1). Gravimetric systems are less amenable to parallelization compared to volumetric systems, as often the bulk and the cost of the system is dictated by the microbalance. However, systems which can accommodate multiple samples at the same time are commercially available. Gravimetry also has the potential of automation, by using an autoloader to increase throughput (Chanut et al., 2017). For reference, simplified schematics of typical volumetric and gravimetric experimental setups are depicted in Figure 4.
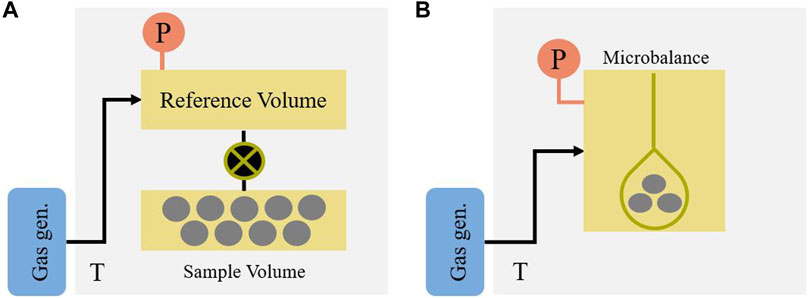
FIGURE 4. Simplified schematic of typical (A) volumetric experimental setup and (B) gravimetric experimental setup.
3.1.3 Breakthrough analysis
Breakthrough methods are based on the fixed-bed principle, in which, the response to a step change in adsorbate concentration is monitored at the end of a packed column of adsorbent. The shape of the resulting breakthrough curve or curves provides equilibrium, bed transport and kinetic information (Dantas et al., 2011; Patel, 2019). A typical breakthrough instrument consists of three main sections: generation, adsorption and detection (see Figure 5A). Generation is where the desired adsorbate or mix of gases is prepared at a constant flowrate, for carbon capture experiments typically a mixture of CO2 and N2 is used. The mixture of gases then enters the adsorption section into the adsorbent packed column. The column can typically be regenerated in-situ via heating using a water bath, oven, or heating tape, or via vacuum through a vacuum pump. As the gas is being adsorbed, the column starts to saturate until each gas reaches the “breakthrough point” (see Figure 5B). Finally, the concentration profile of each gas is monitored at the exit of the column using a gas detector, typically a mass spectrometer, a thermal conductivity detector (TCD), or a non-dispersive infrared (NDIR) gas analyzer.
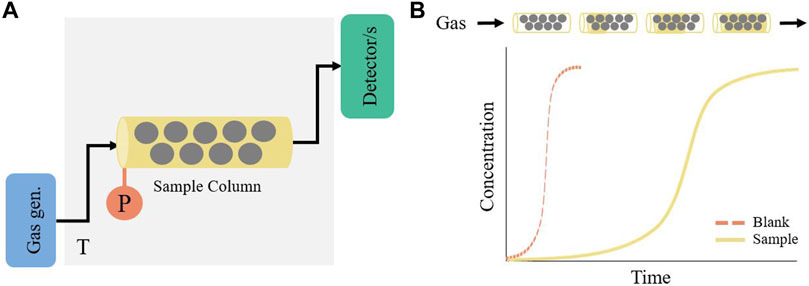
FIGURE 5. (A) Simplified schematic of a breakthrough experimental setup and (B) graphical representation of a breakthrough curve as gas travels through the packed column.
Following the completion of the experiment, either after a designated cycle time or upon sample saturation, the concentration profile can be integrated to calculate the amount of CO2 adsorbed and by extension the adsorption capacity (q). Providing the detector can independently measure multiple species’ concentrations without interference, the “breakthrough” and “equilibrium” adsorption capacity for each species can be calculated according to:
where Fin is the inlet flowrate of the specific gas, t is the adsorption time, Vdead is the dead volume of the system, Fout is the outlet concentration of the specific gas and m is the dry mass of the adsorbent (Oschatz and Antonietti, 2018). Important to consider when carrying out breakthrough experiments is to correctly account for the dead volume of the system (Vdead), this integral should be calculated between the signals of the sample column and a “blank” response with an equivalent volume but no adsorbent present (Rajendran et al., 2008). It should be noted that in the case of highly competitive systems, e.g., CO2/H2O, CO2/N2, CO2/H2 etc. It may be necessary to also perform a desorption experiment, as the integration of very small areas can yield inaccurate results (Wilkins et al., 2021).
The amount of sample required in breakthrough experiments will depend on the CO2 uptake of the material and the size of the column. Breakthrough systems usually require a higher amount of sample than volumetric or gravimetric systems. However, best practices have been recently proposed to address this issue (Wilkins et al., 2022). Due to the fixed-bed configuration, samples in fine powder form may result in high pressure drops, therefore breakthrough systems are better suited to work with monoliths or pellets.
In general, breakthrough is the preferred method to measure multi-component adsorption (Shade et al., 2022) (see Section 3.2.1). Despite some commercial instruments available in the market, the use of home-built breakthrough experimental systems is still prevalent (Shade et al., 2022). A comprehensive understanding of the critical features of an experimental rig and systematic experimental procedures in an in-house build system have been described by Wilkins et al. (2021). It should be noted that good breakthrough performance alone does not indicate good process performance, e.g., small plant size or low cost. However, breakthrough experiments are an important step in gathering experimental data to evaluate adsorbents and undertake rigorous process modelling.
3.1.4 Desorption methods
Desorption methods may include gravimetric and volumetric desorption, the Zero-Length Column (ZLC) and the differential column technique (DCT) (Shade et al., 2022). Here we focus on the ZLC method which is widely used in gas sorption studies for carbon capture (Mangano et al., 2013; Hu et al., 2015; 2018; Krishnamurthy et al., 2020).
The Zero-Length Column (ZLC) system, a short packed-bed typically <1 cm in length, acts as a breakthrough method under low flowrates using either the uptake or desorption curve. The manipulation of flow rates/pressures, feed concentrations, humidity, and temperatures, limited when using traditional chromatographic equipment, allow the simulation of industrially relevant conditions. CO2 concentration is typically measured using a similar suite of sensors as breakthrough (Teng et al., 2017).
At low purge flow rates, the integration of the desorption curve allows a measurement of total amount adsorbed or desorbed (Brandani et al., 2003). Since the mass balance in the ZLC column is well defined, adsorbed concentrations can be calculated with ease, often without the need for blank measurements (the empty system must be characterized at least once). The advantages of the ZLC technique arise from the nature of the small, packed bed, with sample mass used usually <15 mg (Mangano et al., 2013). A smaller bed is required to maintain the zero-length assumption, and minimize heat effects, but it also equates to short experimental times (typically <1 h) making the technique useful for high throughput measurements (Mangano et al., 2013). Naturally, a smaller sample mass means that the associated observed uptake is more susceptible to experimental or measurement errors, which arise from signal noise, the incorrect use of flowrate, or an incorrect measurement of the blank response. An insight into the correct analytical procedures has been provided by Brandani et al., for all ZLC measurements (Brandani and Mangano, 2021). Further to measuring CO2 uptake, the ZLC method has also been used to obtain full isotherms by running multiple adsorption-desorption steps. Finally a recently proposed method also allows the simultaneous determination of isotherms and kinetics (Azzan et al., 2022).
3.2 Selectivity
Selectivity is the preferential adsorption one component over others in a mixture at process conditions. In any real-world CO2 adsorption process, the presence of competing gases will inevitably affect both the rate and adsorption capacity of CO2. This effect can range from reduced performance to irreversible saturation of the material. As such, it is generally desirable to increase any adsorbents’ selectivity towards CO2 over other competing adsorbates. It should be noted that in certain cases, the presence of other components can be beneficial for the capture process (Wang et al., 2011; Zárate et al., 2016). Underlying effects for selective adsorption in solid sorbents may be provided by equilibrium separations (i.e., the selective uptake of CO2 based on physical interactions) or kinetic separations (i.e., the selective uptake of CO2 due to faster diffusivity than other components).
There are several related parameters which are often defined as selectivity. For an equilibrium process, selectivity (S) at a specific pressure and temperature is defined as the ratio of the concentration of the target component in the adsorbed phase and the concentration in the gas phase. Calculation of this metric necessarily implies knowledge of the composition of the two phases. However, true multicomponent adsorption data is difficult to obtain. Instead, single component isotherms measured with volumetric or gravimetric methods are often used to assess a material’s adsorption affinity towards each species (Hunter-Sellars et al., 2020b; Principe and Fletcher, 2020; Xie et al., 2020). This approach is typically done using the ideal adsorbed solution theory (IAST), which is a thermodynamic approach analogous to Raoult’s law for the gas phase (Myers and Prausnitz, 1965; Walton and Sholl, 2015).
An alternative method used to approximate selectivity from single component isotherm data, is the Henry’s law (Knaebel, 1995). This method is useful when the full isotherm data is not available since selectivity is calculated based on the Henry’s coefficients (KH) of the gases following the Eq. 3.
The use of thermodynamic approaches to predict multicomponent data is widespread, though simplified approaches like IAST and Henry’s law have their own limitations. For example, IAST approximation is not valid when the assumption of an ideal adsorbed phase is not met (Chen and Sholl, 2007; Fraux et al., 2018). This is particularly relevant to cooperative interactions between guest molecules, chemisorption or flexible hosts (Chen and Sholl, 2007; Fraux et al., 2018). Furthermore, Henry’s selectivity assumes the preference of one guest over the other at infinite dilution, without considering real adsorption behavior like site coverage, heterogeneity or pore filling (Xu et al., 2020). Finally, the ratio of Henry’s constants will reflect real mixture selectivity only at low pressures and loadings (Oschatz and Antonietti, 2018).
3.2.1 The importance of multicomponent adsorption measurements
To obtain a better prediction of process performance, optimize efficiency, and increase the chances of success, it is necessary to characterize all interactions with other gases present (N2, O2, H2O, etc.) (NIST, 2015; Bui et al., 2018; Low et al., 2023). Researchers should aim to perform measurements under conditions that are as close as possible to the actual process being investigated. Multicomponent gas adsorption measurements remain complicated and time-consuming despite the wide range of available techniques (Shade et al., 2022). Here, we discuss some of the issues that gas sorption methods present when measuring multi-component adsorption, highlighting those arising from CO2 and H2O mixtures.
The presence of water vapor is relevant for both point source capture and DAC (Marx et al., 2013). In the case of point source, it has been observed that in physisorptive solids like zeolites, H2O can compete with CO2 for binding sites leading to losses in adsorption capacity (Brandani and Ruthven, 2004; Mfoumou et al., 2018). Whereas in the case of some chemisorptive solids, such as amine-impregnated porous media, H2O can promote carbamate formation and thus cooperative binding of CO2 molecules which often lead to increased CO2 capacity (Choi et al., 2011; Zhang et al., 2017; Pokhrel et al., 2018; Gaikwad et al., 2021).
Breakthrough methods together with volumetric methods, appear to be the most popular to report multi-component adsorption data in general (Shade et al., 2022). Breakthrough data reporting has increased since 2010 due to the method’s ability to evaluate specific separation processes with a small number of data points (Shade et al., 2022). However, when water vapor is present, breakthrough experiments can face several challenges such as water condensation within the system (Wilkins et al., 2020) or sample breakage during direct reactivation (like in the case of zeolites) (Breck, 1973). Process conditions and reactivation temperatures should be carefully considered to avoid this scenario. Typically, water vapor is generated through a bubbler, and reaching a constant mole fraction of H2O in the mixture can take several minutes (Wilkins et al., 2020). When generating a mixture of CO2/H2O it is important to make sure that the desired concentration is reached before feeding it through the packed column (Wilkins et al., 2020).
Hyphenated volumetric methods are the second most common for multi-component adsorption measurements. Commercial instruments require a GC or another analytical method to determine the composition of the gas phase. For multi-component carbon capture experiments, specifically in the case of CO2/H2O mixtures, volumetric instruments are limited to low relative humidity due to the nature of their set-up.
Gravimetric methods can be useful in the measurement of multi-component adsorption both qualitatively and quantitatively. Gravimetric methods have been used to screen the effect of water vapor on the CO2 adsorption performance of different materials. In these studies, water is typically pre-adsorbed on the sample followed by CO2. The CO2 uptake is determined by the difference between the mass after CO2 adsorption and the mass before CO2 adsorption (Chanut et al., 2017; Sáenz Cavazos et al., 2021). When employing this method, the assumption that H2O is not displaced by CO2 should be made. However, as observed by Chanut et al., this depends on the adsorbent’s affinity to water, and this assumption may be valid only for hydrophobic materials where water can move freely and does not have a strong interaction with the adsorbent (Chanut et al., 2017). These experiments are useful to obtain qualitative information about multicomponent adsorption. However, to determine the individual amount of each adsorbed species, the concentration of the gas phase or the distributions in the adsorbed phase should be known (Broom et al., 2020).
In all the above methods, it is important to make sure the gas detection method used does not interfere with the species present in the mixture. For instance, it is well known that mass spectrometers can have issues when dealing with high relative humidity. Or as highlighted by Wilkins et al., CO2 can interfere with capacitance-based relative humidity sensors (Wilkins et al., 2020). Correct calibration measurements can help mitigate this problem but should be performed for each experiment, which can be a time-consuming task.
3.2.2 Capacity versus selectivity, what is more important?
An ideal CO2 adsorbent should exhibit both a high capacity and selectivity towards CO2 (Patel et al., 2017). However, often reported improvements in one property, come at the expense of the other (Oschatz and Antonietti, 2018). There have been some material breakthroughs like the case of SIFSIX, which contains optimal pore size and chemical functionality that enables a “sweet spot” for high capacity and selectivity (Nugent et al., 2013). SIFSIX materials have proven to be useful both for point source capture (Nugent et al., 2013) and DAC (Shekhah et al., 2014).
If a view is taken that a specific separation performance (product purity and recovery) must be achieved, then a minimum selectivity and CO2 working capacity are required. It is generally not possible to predict the working capacity required a priori, and some amount of process modelling is required. It should also be noted that the improved selectivity must be achieved by reducing N2 (or light-component) adsorption, and not by increasing CO2 adsorption (Maring and Webley, 2013; Rajagopalan et al., 2016; Rajagopalan and Rajendran, 2018; Danaci et al., 2020; Subraveti et al., 2022).
In the adsorption process context, total CO2 capacity is not proportional to process performance. The working capacity is the key metric in that case. For example, Mg-MOF-74 displays excellent total CO2 capacity, however, under VSA conditions it is not possible to remove the CO2 effectively and this significantly hampers performance (Maring and Webley, 2013; Danaci et al., 2020). On the other hand, adsorbents such as UTSA-16 and CALF-20 display “low” CO2 capacity, however, their CO2 isotherm shape and low N2 adsorption result in good process performance under VSA conditions (Khurana and Farooq, 2016; Danaci et al., 2020; Nguyen et al., 2022). Therefore, it is necessary to optimize CO2 working capacity, and selectivity (minimum N2 adsorption), in tandem to increase the chances of good process performance.
3.3 Kinetics
Kinetic information is critical to the simulation of adsorption/separation processes, process scale-up and design (Taddei and Petit, 2021; Low et al., 2023). Despite its importance, kinetic parameters are less reported than gas adsorption and selectivity in adsorptive-based CCS studies (Low et al., 2023). The small critical diameter of CO2 results in intra-particle mass transfer processes that can be limited by either micropore diffusion and or surface diffusion. Often with porous materials there is a hierarchy of pore sizes, through which adsorbates must diffuse. These pores may resist the overall mass transfer in the form of:
• Micropore intraparticle resistance.
• Transport resistance observed on the particle surface.
• Meso- and macropore interparticle diffusion.
Diffusion in porous materials can be classified into molecular and effective diffusion. Generally, molecular diffusion follows the movement of molecules from a gas or liquid phase solution into or out of a single pore, whereas effective diffusion is the sum movement of molecules through the entire pore network, tortuosity (Levy et al., 2015). Further distinctions can be made between self-diffusion and counter-diffusion, with the former an assessment of diffusion between same molecules, and the latter an assessment of diffusion in the presence of competing molecules (Brandani et al., 2000). In particles, diffusion can occur at several length scales interparticle diffusion typically comprises of macropore (macroscopic) diffusion within a packed bed, whereas intra-particle/inter-crystalline diffusion is made up of both macropore and micropore, and intra-crystalline comprises of micropore (microscopic) diffusion (see Figure 6) (Bhatia, 1997). The most common methods to measure the different types of diffusion are presented below. Each technique follows the rate of diffusive migration of molecules from saturated porous materials, however, they differ in the measurement mode and in the significance of the data produced (see Table 3).
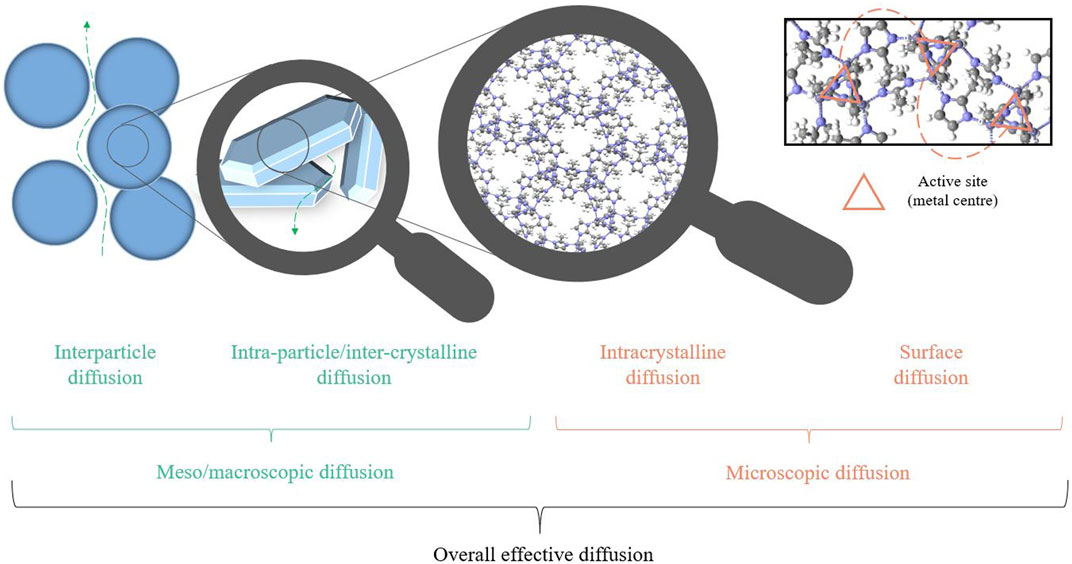
FIGURE 6. Summary of diffusion processes that occur in adsorbents and contribute to the overall effective diffusion, exemplified on a crystalline solid sorbent.
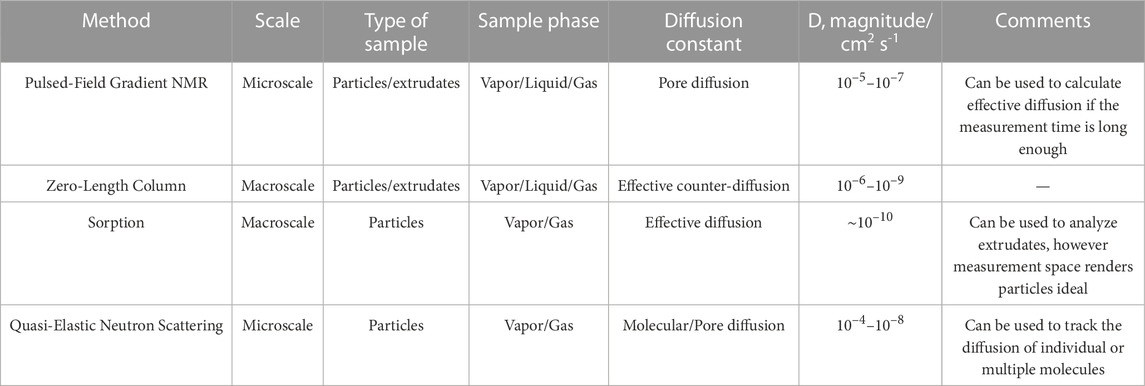
TABLE 3. Types of diffusion that can be measured with different methods including the typical orders of magnitude of results obtained.
3.3.1 Gravimetric and volumetric methods
Many of the modern gravimetric and volumetric systems continuously monitor changes in adsorbate loading as a function of time during simulated process conditions, this allows the dynamics of the sorption process to be quantified (Hong et al., 2016; Ojwang et al., 2018). Due to the system’s numerous transport resistances, it is common to fit experimental data to different diffusion models (e.g., film or intraparticle) to provide information on the rate-controlling process during adsorption. It is important, however, not to simply use just goodness-of-fit to identify the most suitable diffusion model (Loganathan et al., 2014). Alongside careful consideration of model assumptions and their relevance in different adsorbate-adsorbent systems, a recent review by Wang et al., concluded that kinetic parameters derived from gravimetric and volumetric data can be strongly influenced by the nature and conditions of the experimental method. This can include but is not limited to the dynamics of the experimental system reaching equilibrium in a similar timeframe to the adsorption; external mass transfer resistances in aggregated particles; isotherm nonlinearity (i.e., differences in adsorption and desorption kinetics in a similar pressure region) and non-isothermal conditions (Wang et al., 2021). The authors recommend several experimental guidelines and checks that should be carried out such as: testing multiple particle sizes, sample masses and configurations; reducing the magnitude of pressure or concentrations changes in the system; and accounting for experimental effects related to buoyancy, flowrate, drift, and heat transfer (Wang et al., 2021).
3.3.2 Zero-length column
The operating conditions in ZLC may be manipulated to assert kinetic or equilibrium control, allowing the determination of parameters such as diffusion constants, mass transfer coefficients (Brandani and Mangano, 2022) and equilibrium adsorption coefficients (Brandani et al., 2003). For ZLC kinetic experiments, packed beds (<15 mg) made up of small particles of a known radius are used (Gibson et al., 2016). The system may be run at high purge flow rates resulting in kinetically controlled desorption, or at low purge flow rates resulting in equilibrium-controlled desorption. By measuring the concentration loss from a packed bed at high purge flow rates, a measurement of the gradient allows the limiting diffusion kinetics to be determined. Further, ZLC diffusion experiments can aid in decoupling kinetic and mass transfer limitations in functionalized materials with amine impregnation, or hydrophobic modification (Teng et al., 2017; Gelles and Rezaei, 2020). In cases of competitive adsorption, binary mixtures of CO2 and other components may be run to assess the impact of the competing molecule on uptake kinetics (Brandani and Ruthven, 2004). Finally, diffusion experiments have been used to probe structural changes in flexible adsorbents upon adsorption of CO2, by running diffusivity measurements pre- and post-material saturation with CO2 (Lozinska et al., 2012). It is important to note that ZLC measurements of diffusivity are typically restricted to the Henry’s constant region (Eic and Ruthven, 1988).
3.3.3 Pulsed-field gradient (PFG) NMR
Pulsed-Field Gradient (PFG) NMR allows the determination of microscopic CO2 diffusion in porous materials by recording relaxation of polarized nuclei. This technique measures the average molecular diffusion of adsorbates through intraparticle and interparticle pores. Under longer diffusion analysis times, the PFG-NMR technique can also provide information on the much more hindered diffusion occurring where adsorbates interact with pore walls, providing effective diffusion constants (Díaz et al., 2010). Instead of measuring limiting diffusion, PFG-NMR can measure the varying contributions of different diffusional pathways (such as intrapore or interpore) dependent on the diffusion analysis time (Mueller et al., 2012; Pugh and Forse, 2023). Furthermore, since the technique can also make direct measurements of the adsorbate bulk diffusion, a comparison of bulk diffusion/transport diffusion provides more meaningful comparisons across different adsorbates compared to the effective diffusion constants achieved by ZLC. PFG-NMR requires a larger sample compared other methods, around ∼100 mg (Grenier et al., 1994). Multicomponent diffusion may be measured easily with PFG-NMR when adsorbates possess different NMR active nuclei (Rittig et al., 2003), for example CO2 and NOx diffusion may be decoupled by measurement of the 13C and 15N nuclei respectively. The reader is also directed to some recent works summarizing the PFG NMR technique (Baniani et al., 2021; Kärger et al., 2021).
3.3.4 Quasi-elastic neutron scattering (QENS)
Quasi-Elastic Neutron Scattering (QENS) is a microscale technique that provides molecular scale self-diffusion constants, and transport-diffusion constants at larger length scales. These diffusion constants are calculated from scattering functions which depend on the space-time positions of atoms (Kruteva, 2021). QENS experiments can provide information around the transport diffusivity of adsorbed CO2 and N2 in adsorbent materials (Papadopoulos et al., 2004). QENS diffusion constants are often comparable to those found from molecular dynamics due to the similar time (10–13 –10–7 s) and length scales (Embs et al., 2010). Since neutron scattering requires a source of neutrons of known momentum, specialist facilities are required.
3.4 Ease of regeneration
Following the capture of CO2, the adsorbent must be regenerated to release CO2 for conversion or storage, and for its re-use in subsequent adsorption cycles. As mentioned before, regeneration can be accomplished through the application of elevated temperatures, sweeping gas or vapor, reduced pressure, or combinations thereof.
In pressure swing adsorption processes (PSA/VSA), the ease of regeneration is reflected by the pressure of the blowdown and desorption steps. As compressors and vacuum pumps can represent a large percentage of the operating costs, it is recommended to have an adsorbent that takes up all its CO2 capacity in a narrow limit of pressure and preferentially close to process conditions (Ho et al., 2008; Subraveti et al., 2019). Here, isotherm-based analysis, WC and process modeling are used to understand the overall process performance.
Regarding temperature swing adsorption processes (TSA), the regeneration step requires heat input sufficient to desorb CO2 to a desired working capacity, based on optimal process conditions. The regeneration heat requirement accounts for (Zhang et al., 2016):
1. The sensible heat necessary to increase the adsorbent bed from the adsorption temperature to the desorption temperature.
2. The heat of desorption, or the energy required to overcome the physical or chemical interaction between CO2 and the adsorbent.
The first term is dominated by the heat capacity of the material (see Section 2.1.3) and the adsorbed phase, while the second is quantified by the enthalpy of adsorption (
Enthalpy of adsorption can also be measured directly through determination of evolved heat throughout adsorption, by using a calorimetric setup. In its most common embodiment, isotherms and adsorption enthalpies are measured simultaneously by placing the sample in one side of a Tian-Calvet differential calorimeter and performing a volumetric measurement while recording and integrating the calorimeter signal (Llewellyn and Maurin, 2005). The experiment is more challenging, as it involves non-trivial instrumentation and larger sample amounts, however, it provides an unequivocal, direct measurement of
3.5 Stability
Promising CO2 adsorbents should remain stable over thousands of adsorption and regeneration cycles (Bui et al., 2018). Beyond assessing the mechanical, chemical, and thermal stability of an adsorbent, it is crucial to gauge its cycling stability under pertinent operational conditions. This comprehensive evaluation is essential to grasp a holistic picture of the adsorbent’s stability. Cycling experiments are usually performed using breakthrough or modified TGA apparatus (Low et al., 2023). However, often only a few cycles are performed (∼10) due to time constraints (Low et al., 2023). Furthermore, other impurities such as H2O, O2 and other acidic gases can induce sorbent degradation or deactivation, this is often measured indirectly via mass loss events (Sayari et al., 2012a; Si et al., 2019) or reductions in adsorption performance (Thouchprasitchai et al., 2018; Elfving et al., 2021). A recent study by van Paasen et al., investigated the impact of these types of degradation on an amine-functionalized adsorbent used for post-combustion CO2 capture (van Paasen et al., 2021). However, these indirect methods usually offer a limited understanding of the mechanisms by which adsorbents degrade. Below, different degradation pathways that can occur and how they can be identified are discussed.
3.5.1 CO2-induced degradation
Adsorption based on chemical reaction of amine groups with CO2 can, alongside formation of carbamate and bicarbonate, form urea linkages, significantly inhibiting the ability of the adsorbent to capture CO2 (Sayari et al., 2012b). This degradation is exacerbated at elevated temperatures and CO2 concentrations, such as those observed in point source capture or processes using high-purity CO2 as a desorption sweep gas (Choi et al., 2016). The exact conditions necessary for urea formation depend on a range of factors including the nature of the amine (Sayari et al., 2012a), and the presence of water vapor within the gas stream. The presence of urea linkages can be confirmed using either ex-situ (Sayari et al., 2012b) or in-situ (Said et al., 2020) spectroscopic analysis.
3.5.2 Oxidative stability
Compared to many sorbents such as zeolites and carbons, the volatile and organic nature of amine-based chemisorbents makes reactions with oxygen a concern. Oxidation of grafted amines was confirmed by Calleja et al., who, through pre- and post-exposure DRIFT spectra, detected additional bands attributed to C=N stretching vibrations (Calleja et al., 2011). These changes were used to explain significant losses in CO2 capacity, which could not be fully attributed to losses in nitrogen content, measured via elemental analysis (Calleja et al., 2011). Furthermore, MOFs with open metal sites can bind O2 irreversibly above a certain temperature depending on the material (Bloch et al., 2011).
Since early studies, the dependence of amine oxidation on material- and process-specific factors has been investigated. This includes, but is not limited to: the temperature during oxidation (Nezam et al., 2021); oxygen concentration (Min et al., 2018); the presence of water vapor (Sayari and Belmabkhout, 2010) or CO2 (Min et al., 2018) in the environment; the size and chemistry of the amine(s) (Calleja et al., 2011; Heydari-Gorji et al., 2011); and the presence of chemical additives within the composite (Min et al., 2018). Analysis of the chemical composition of the sample, or the species emitted during oxidation may be accomplished using spectroscopic methods (Meng et al., 2019), e.g. mass, infrared, NMR. These techniques are then combined with metrics of CO2 capture, typically measured using gravimetric, volumetric, or fixed-bed methods to understand the role of oxidation on performance. While analysis of chemical changes in-situ presents additional complexity in terms of experimental design, the value of detecting chemical changes during realistic process conditions is a key driving force to the different experimental approaches being developed (Du et al., 2010; Köck et al., 2013; Moore et al., 2018; Roztocki et al., 2021).
3.5.3 Hydrolytic stability
Water is ubiquitous in CO2 capture applications, typically existing as either vapor, pressurized steam, or condensed liquid. As mentioned previously, a material’s affinity for water vapor may be assessed via its surface chemistry (Ding and Jiang, 2020), or by isotherm analysis (Fröhlich et al., 2014). Compared to water vapor, steam can have much more significant impacts on material stability due to its high temperature and pressure. Porous silicas, commonly used as amine-functionalized supports, can undergo structural collapse during steam exposure. This effect, typically detected via porosimetry or electron microscopy, has been observed in several silica-based materials (Fayaz and Sayari, 2017; Min et al., 2018). The presence and extent of collapse appears to depend on both process conditions, such as temperature and duration of steaming, and material properties, such as the pore size and wall thickness (Zhang et al., 2005; Jahandar Lashaki et al., 2019). The nature of steam exposure makes in-situ analysis difficult, compared to the relatively mild pressures and temperatures of ambient levels of humidity.
3.5.4 Stability to acidic gases and other impurities
Compared to levels of CO2, N2, O2 and H2O, acidic gases such as SO2, NO2, and H2S are found at significantly lower concentrations. However, even at part-per-million concentration levels, amine-functionalized adsorbents can undergo irreversible reactions with SO2 and NO2, measured via Raman spectroscopy, leading to significant reductions in CO2 capacity (Rezaei and Jones, 2013). This irreversibility has also been confirmed in multi-component fixed-bed measurements, finding that while CO2 and NOx can both be adsorbed simultaneously, the latter compound was not removed during regeneration (Xu et al., 2005). The impact of these acidic gases on supported amines appears to be dependent on the nature of the supported amine (Rezaei and Jones, 2014), the temperature of the system (Liu et al., 2014), and the gases’ concentration (Liu et al., 2011). For example, Liu et al. found that while NO had no significant influence on CO2 capacity up to 400ppm, SO2-induced losses increased from 2%–3% at 300ppm to 24%–25% at 400ppm, hypothesized to be due to the formation of heat-stable salts (Liu et al., 2011). Alongside reactions with amines in functionalized adsorbents, SO2 has been found, via diffraction measurements, to induce structural degradation in Mg2(dobdc) (Yeon et al., 2015) and Mg2(dobpdc) (Jo et al., 2017). In the case of CO2 removal, ambient air, where concentrations of these compounds are not expected to exceed the part-per-billion concentration range, performance losses due to these species are typically neglected (Stedman et al., 1997; Sanz-Pérez et al., 2016; Zhang et al., 2021).
3.6 Adsorbent cost and environmental impact
Determining the adsorbent cost for a carbon capture process at scale can be difficult, particularly for novel materials being synthesized and tested only at laboratory scale. Using the price of raw materials and energy required during the synthesis process is often used to estimate the adsorbent cost (Sinha et al., 2017). Researchers have also proposed the use of factors and rules of thumb, highlighting that their use may not apply when the raw materials are expensive and cannot benefit from economies of scale (Sinha et al., 2017; Danaci et al., 2021). Recently, Azarabadi et al., proposed a model that can estimate how much the adsorbent should cost in order to have an economically feasible process, rather than how much its actual bulk price or production cost is (Azarabadi and Lackner, 2019). The model was built for DAC, but the principle can also be applied to point source capture, and it demonstrates useful to set a limit or benchmark on the adsorbent cost (Azarabadi and Lackner, 2019). Nevertheless, the model predictions can be greatly influenced by particular CO2 markets and the adsorbent lifetime. The problem with the latter is the scarcity of accurate data available in literature (Azarabadi and Lackner, 2019). It should be noted that there are many factors that dictate the economic feasibility of an adsorption process, and even if the adsorbent is ‘free’, other costs may still dominate the cost of CO2 capture (Subraveti et al., 2022).
The environmental impact of adsorbents for CO2 capture is rarely reported in the literature. There are very few studies that include experimental characterization together with the environmental impact of newly proposed adsorbents (Fayemiwo et al., 2019; Leonzio et al., 2022a; Leonzio et al., 2022b). Usually, Life Cycle Assessment (LCA) is used to evaluate the environmental performance of a process or product. Studies like the one by Grande et al., have assessed the environmental footprint of adsorbents themselves (Grande et al., 2017), while others have analyzed both the adsorbents together with the carbon capture process (Deutz and Bardow, 2021; Leonzio et al., 2022b; Heidari et al., 2022). When looking at the adsorbent production as a system boundary, it is important to highlight that between 60%–91% of their total carbon footprint comes from their production and the rest from their end of life (Deutz and Bardow, 2021). Furthermore, amine synthesis and the utilization of organic solvents can have a significant impact on climate change. Albeit efforts towards green synthesis procedures, many of the novel proposed materials are functionalized using amines. Ultimately, both the adsorbent production/disposal and use in a specific capture process are required to have a comprehensive environmental impact assessment. Studies like the one from Heidari et al., which investigates the most suitable adsorbent option from an environmental, economic, and technical perspective can be very valuable (Heidari et al., 2022).
4 Process performance indicators
Full experimental characterization of promising adsorbents for CO2 capture should be coupled with process modelling and techno-economic analysis to better understand and optimize an actual process. Usually, key process performance indicators include purity, recovery, productivity, energy consumption, specific energy, and cost (Farmahini et al., 2021; Taddei and Petit, 2021; Low et al., 2023).
Experimental data obtained from the intrinsic material properties and adsorbent performance parameters is often required to build and validate process models. One of the main challenges here is finding a full and comparable set of data at relevant process conditions for different adsorbents. It is important to note that isotherms of at least three temperatures are required for all species considered in the modelling. Furthermore, experimental data for adsorption isotherms is formed by discrete points and require an analytical expression describing adsorption equilibria to serve as input in process models. The most common approach to obtain this is the dual-site Langmuir (DSL) adsorption model (Farmahini et al., 2018). However, it is important to consider the actual mechanism of adsorption in the different materials used (physi- and/or chemisorbents), as they may require different mathematical models to accurately describe them. Indeed, for the new proposed materials, it can be difficult to find a suitable analytical expression (Farmahini et al., 2021). One way to overcome this limitation is to perform a set of single-component breakthrough experiments at different fluid phase concentrations and describe the relationship as a set of discrete points (Rajendran et al., 2020). Recently, Farmahini et al., compiled an exhaustive list of the input parameters required for a PSA/VSA process simulation and while some depend on design specifications or can be obtained from literature, more than half require some sort of experimental method (Farmahini et al., 2021).
When evaluating process performance indicators, it is important to understand the differences between point source capture and DAC processes. For instance, in point source capture, purity and recovery are usually set values and optimization tends towards cost minimization. Whereas in DAC processes there is no current specification on recovery and optimization focuses on minimizing specific energy (Low et al., 2023). It should be noted that for DAC processes, although cost and energy are important considerations, the key requirement is for the process to have net CO2 removal from the atmosphere. This will indirectly impose some requirements on CO2 recovery, and the end-use of CO2 will impose some requirements on the purity of the CO2. Nevertheless, the ultimate goal will always be to reduce the overall cost of CO2 capture, and therefore linking process modelling with techno-economic analysis plays a key role. The main drawback observed here is the lack of adsorbent cost and lifetime data availability (Danaci et al., 2021; Farmahini et al., 2021).
5 Interconnection between KPIs
In previous sections there have been brief mentions of the interconnections between the different KPIs. Undoubtedly, understanding these links can help devise more effective and tailored solutions for adsorption-based carbon capture processes. In a recent study, Taddei and Petit discussed the different existing links between process and sorbent parameters focusing specifically on MOFs (Taddei and Petit, 2021). It is important to note that not all materials will behave the same and interconnections between KPIs can vary depending on the specific material structure. However, it is possible to establish some ‘common’ relationships between material intrinsic properties, adsorption performance and process effectiveness.
Starting with CO2 adsorption capacity, the adsorbent surface area and pore morphology parameters typically affect material suitability in CO2 capture applications. For free-standing physi- and chemi-sorbents, these properties can directly influence CO2 uptake by increasing the number of available sites for CO2 to interact with (Alazmi et al., 2018; Akpasi and Isa, 2022); while for amine-functionalized chemisorbents, the degree of amine grafting or impregnation, and the subsequent accessibility of these amines strongly depends on the support’s morphology (Franchi et al., 2005; Vilarrasa-García et al., 2015; Zhang and Du, 2022). Aside from CO2 adsorption capacity, the rate at which the CO2 molecules diffuse through solid sorbents and adsorb on active sites is important to determine process parameters like cycle time and overall process productivity (Wu et al., 2022). Regarding the ease of regeneration, strong binding of CO2 to the adsorbent can result in greater energy consumption in the desorption step, hamper CO2 recovery, and increase capture costs (Patel et al., 2017). Moreover, adsorbent stability and lifetime can have an impact on the operating expenses of a capture facility, adsorbents that degrade rapidly or lose their capture efficiency over time necessitate frequent replacements, contributing to increased operating costs.
Indeed, this work underscores the necessity of a thorough characterization of solid sorbents for CO2 capture, extending beyond the conventional parameters of capture capacity or selectivity. It directs particular attention on how to measure the often overlooked but vital aspects such as kinetics and material operational stability. By offering a holistic understanding of the materials’ performance under realistic working conditions, informed decisions can be made on their practical utility. The insights gathered from comprehensive assessments could catalyze the development of more robust, efficient, and economically viable carbon capture technologies.
6 Data reproducibility and homogenization efforts
The critical evaluation of literature pertaining to solid sorbents for CO2 is often hampered by data availability and reproducibility. Calculation of KPIs detailed above can be highly variable, not only due to material variability and experimental conditions, but also due to user choices (Park et al., 2017). We highlight a recent interlaboratory study that emphasizes the lack of reproducibility in the determination of BET areas from the same raw data (Osterrieth et al., 2022). Many measurements are only reported graphically, making interpretation of exact numbers difficult. Further knowledge of secondary metadata, such as activation temperature, assumed dead volume, flowrates, is invaluable for understanding the full picture.
Special mention should be given to several efforts which attempt to introduce standardization and shareability to all KPI-relevant data, adsorption in particular. One of the first attempts towards this end is the NIST ISODB, a collection of more than 32 k isotherms digitized from published data (Siderius et al., 2015). The authors also introduce a standard sharable format based on JSON, which can encode isotherm data and metadata, both for single and multi-component adsorption. More recently, a large-scale collection of more than 900 multicomponent isotherms were assembled (Cai et al., 2021). This study, which analyzed more than 11 k publications, could only unearth about ∼50 CO2/N2 isotherms, underlining a lack of complete multicomponent adsorption data in existing literature.
Progress has been made towards developing a data format that is both human- and computer-readable, an adsorption information file, or AIF, analogous to a crystallographic CIF (Evans et al., 2021). The AIF aims to become the de facto standard for sharing isotherm data and is currently the focus of an IUPAC project (IUPAC, 2021). A similar IUPAC standardization effort is underway with the aim of providing best practices and guidelines for diffusion measurements (IUPAC, 2015). These initiatives can hopefully gain traction within the adsorption community, and help establish a common language that would aid the efforts of screening solid sorbents for CCUS (Pini et al., 2022).
7 Conclusion and outlook
Achieving technical maturity and wide scale implementation of CO2 capture from relevant sources (flue gas or air) requires an integrative approach, from understanding the intrinsic material properties at an atomic scale all the way through pilot-scale testing and demonstration plants. Experimental adsorbent characterization techniques play a key role in helping researchers understand an adsorbent’s physical and chemical characteristics as well as adsorption performance and can be coupled with process modeling and techno-economic analysis to optimize cost and process performance.
Thorough adsorbent characterization is complex and time-consuming task. Performing the full gamut of experiments requires access to expensive laboratory instrumentation and often, specialized equipment and people for adsorption measurements. It is acknowledged that all the characterization proposed here is unreasonable for a single research group to carry out. Therefore, cooperation and collaboration between multidisciplinary laboratories is required to enhance, fast-track, and share best practices regarding sorbent characterization and evaluation.
In terms of measurement and characterization, new adsorbents are normally prepared in milligram scale batches. Although a difficult endeavor, adsorption equipment manufacturers should aim to develop instruments and techniques that are compatible with such low sample amounts. It would also be desirable to undertake these measurements in a somewhat standardized manner including both equilibrium and kinetics data sets, to facilitate comparison between adsorbents and publications.
From a materials design perspective, the “adsorbent–process marriage” remains ever relevant (Sircar, 2001). The required adsorbent properties are a function of the process conditions, co-adsorbing components, any impurities present, and the cycle-design. Therefore, fundamental materials characterization is the first step that feeds into adsorption process modelling and design. Generating these links between materials properties and process behavior is imperative to better understand them.
In terms of adsorption scale-up and commercialization, there are several factors that need to be addressed. Adsorbent cost should be as low as possible, and reagents used in their preparation should be bulk or commodity chemicals. Although not front-of-mind in the past, the environmental impacts should also be evaluated through a ‘cradle-to-grave’ life-cycle analysis, especially if overall process decarbonization is desired. The behavior of novel adsorbents is also unknown from a disposal perspective and needs to be investigated further. Adsorbent recycling or raw material recovery could also be considered.
Adsorption-based carbon capture processes will play a role in industrial decarbonization, and carbon dioxide removal from the atmosphere. There has been significant focus on adsorbent development for carbon capture applications over the last two decades. However, a divide remains between “materials” and “process”. If it is desired to expedite technology development and deployment, the divide needs to be bridged. We hope that this work highlights the entwined nature of adsorption processes, initiates discussions between parties, and encourages more coordinated research efforts towards deeper understanding of adsorption performance.
Author contributions
The authors confirm contribution to the paper as follows: PS: initial study conception, design, writing–original draft preparation. PS, EH-S, PI, and SM: conceptualization, writing–original draft preparation, review, and editing. DD: writing, review, and editing DW: review and editing. All authors contributed to the article and approved the submitted version.
Funding
PS was supported by CONACyT-SENER PhD grant in Energy and Sustainability and Imperial College London studentship. SM was supported by (UKRI-EPSRC) and Imperial College London studentship. DD was supported by funding from UK Research and Innovation (UKRI) under grants EP/P026214/1 and EP/T033940/1.
Acknowledgments
The authors would like to thank Lucy Victoria Barton, May-Yin (Ashlyn) Low, and Camille Petit from the Department of Chemical Engineering at Imperial College London for their valuable consultations and input regarding this topic.
Conflict of interest
Author PI is employed by Surface Measurement Systems.
The remaining authors declare that the research was conducted in the absence of any commercial or financial relationships that could be construed as a potential conflict of interest.
Publisher’s note
All claims expressed in this article are solely those of the authors and do not necessarily represent those of their affiliated organizations, or those of the publisher, the editors and the reviewers. Any product that may be evaluated in this article, or claim that may be made by its manufacturer, is not guaranteed or endorsed by the publisher.
References
Akpasi, S. O., and Isa, Y. M. (2022). Effect of operating variables on CO2 adsorption capacity of activated carbon, kaolinite, and activated carbon – kaolinite composite adsorbent. Water-Energy Nexus 5, 21–28. doi:10.1016/j.wen.2022.08.001
Alazmi, A., Tall, O. E., Hedhili, M. N., and Costa, P. M. F. J. (2018). The impact of surface chemistry and texture on the CO2 uptake capacity of graphene oxide. Inorganica Chim. Acta 482, 470–477. doi:10.1016/j.ica.2018.06.031
Ambroz, F., Macdonald, T. J., Martis, V., and Parkin, I. P. (2018). Evaluation of the BET theory for the characterization of meso and microporous MOFs. Small Methods 2 (11), 1800173. doi:10.1002/smtd.201800173
Azarabadi, H., and Lackner, K. S. (2019). A sorbent-focused techno-economic analysis of direct air capture. Appl. Energy 250, 959–975. doi:10.1016/j.apenergy.2019.04.012
Azzan, H., Rajagopalan, A. K., L’Hermitte, A., Pini, R., and Petit, C. (2022). Simultaneous estimation of gas adsorption equilibria and kinetics of individual shaped adsorbents. Chem. Mater. 34 (15), 6671–6686. doi:10.1021/acs.chemmater.2c01567
Bae, T.-H., Hudson, M. R., Mason, J. A., Queen, W. L., Dutton, J. J., Sumida, K., et al. (2013). Evaluation of cation-exchanged zeolite adsorbents for post-combustion carbon dioxide capture. Energy & Environ. Sci. 6 (1), 128–138. doi:10.1039/C2EE23337A
Baniani, A., Berens, S. J., Rivera, M. P., Lively, R. P., and Vasenkov, S. (2021). Potentials and challenges of high-field PFG NMR diffusion studies with sorbates in nanoporous media. Adsorption 27 (3), 485–501. doi:10.1007/s10450-020-00255-y
Bao, J., Yang, Q.-h., Zeng, S.-q., Sang, X.-y., Zhai, W.-m., and Nie, H. (2022). Synthesis of amorphous silica-alumina with enhanced specific surface area and acidity by pH-swing method and its catalytic activity in cumene cracking. Microporous Mesoporous Mater. 337, 111897. doi:10.1016/j.micromeso.2022.111897
Bardestani, R., Patience, G. S., and Kaliaguine, S. (2019). Experimental methods in chemical engineering: Specific surface area and pore size distribution measurements—BET, BJH, and DFT. Can. J. Chem. Eng. 97 (11), 2781–2791. doi:10.1002/cjce.23632
Bazer-Bachi, D., Assié, L., Lecocq, V., Harbuzaru, B., and Falk, V. (2014). Towards industrial use of metal-organic framework: Impact of shaping on the MOF properties. Powder Technol. 255, 52–59. doi:10.1016/j.powtec.2013.09.013
Becattini, V., Gabrielli, P., and Mazzotti, M. (2021). Role of carbon capture, storage, and utilization to enable a net-zero-CO2-emissions aviation sector. Industrial Eng. Chem. Res. 60 (18), 6848–6862. doi:10.1021/acs.iecr.0c05392
Benyahia, F., and O'Neill, K. E. (2005). Enhanced voidage correlations for packed beds of various particle shapes and sizes. Part. Sci. Technol. 23 (2), 169–177. doi:10.1080/02726350590922242
Bhatia, S. K. (1997). Transport in bidisperse adsorbents: Significance of the macroscopic adsorbate flux. Chem. Eng. Sci. 52 (8), 1377–1386. doi:10.1016/S0009-2509(96)00512-X
Bingre, R., Louis, B., and Nguyen, P. (2018). An overview on zeolite shaping technology and solutions to overcome diffusion limitations. Catalysts 8 (4), 163. doi:10.3390/catal8040163
Bläker, C., Muthmann, J., Pasel, C., and Bathen, D. (2019). Characterization of activated carbon adsorbents – state of the art and novel approaches. ChemBioEng Rev. 6 (4), 119–138. doi:10.1002/cben.201900008
Blankenship, L. S., Jagiello, J., and Mokaya, R. (2022). Confirmation of pore formation mechanisms in biochars and activated carbons by dual isotherm analysis. Mater. Adv. 3 (9), 3961–3971. doi:10.1039/D2MA00141A
Bloch, E. D., Murray, L. J., Queen, W. L., Chavan, S., Maximoff, S. N., Bigi, J. P., et al. (2011). Selective binding of O2 over N2 in a redox–active metal–organic framework with open iron(II) coordination sites. J. Am. Chem. Soc. 133 (37), 14814–14822. doi:10.1021/ja205976v
Brandani, F., Ruthven, D., and Coe, C. G. (2003). Measurement of adsorption equilibrium by the zero length column (ZLC) technique Part 1: Single-component systems. Industrial Eng. Chem. Res. 42 (7), 1451–1461. doi:10.1021/ie020572n
Brandani, F., and Ruthven, D. M. (2004). The effect of water on the adsorption of CO2 and C3H8 on type X zeolites. Industrial Eng. Chem. Res. 43 (26), 8339–8344. doi:10.1021/ie040183o
Brandani, S., Jama, M., and Ruthven, D. (2000). Diffusion, self-diffusion and counter-diffusion of benzene and p-xylene in silicalite. Microporous Mesoporous Mater. 35-36, 283–300. doi:10.1016/S1387-1811(99)00228-0
Brandani, S., and Mangano, E. (2022). Direct measurement of the mass transport coefficient of water in silica-gel using the zero length column technique. Energy 239, 121945. doi:10.1016/j.energy.2021.121945
Brandani, S., Mangano, E., and Sarkisov, L. (2016). Net, excess and absolute adsorption and adsorption of helium. Adsorption 22 (2), 261–276. doi:10.1007/s10450-016-9766-0
Brandani, S., and Mangano, E. (2021). The zero length column technique to measure adsorption equilibrium and kinetics: Lessons learnt from 30 years of experience. Adsorption 27 (3), 319–351. doi:10.1007/s10450-020-00273-w
Broom, D. P., Talu, O., and Benham, M. J. (2020). Integral mass balance (IMB) method for measuring multicomponent gas adsorption equilibria in nanoporous materials. Industrial Eng. Chem. Res. 59 (46), 20478–20491. doi:10.1021/acs.iecr.0c04162
Bui, M., Adjiman, C. S., Bardow, A., Anthony, E. J., Boston, A., Brown, S., et al. (2018). Carbon capture and storage (CCS): The way forward. Energy & Environ. Sci. 11 (5), 1062–1176. doi:10.1039/C7EE02342A
Cai, X., Gharagheizi, F., Bingel, L. W., Shade, D., Walton, K. S., and Sholl, D. S. (2021). A collection of more than 900 gas mixture adsorption experiments in porous materials from literature meta-analysis. Industrial Eng. Chem. Res. 60 (1), 639–651. doi:10.1021/acs.iecr.0c05398
Calleja, G., Sanz, R., Arencibia, A., and Sanz-Pérez, E. S. (2011). Influence of drying conditions on amine-functionalized SBA-15 as adsorbent of CO2. Top. Catal. 54 (1), 135–145. doi:10.1007/s11244-011-9652-7
Chanut, N., Bourrelly, S., Kuchta, B., Serre, C., Chang, J.-S., Wright, P. A., et al. (2017). Screening the effect of water vapour on gas adsorption performance: Application to CO2 capture from flue gas in metal–organic frameworks. ChemSusChem 10 (7), 1543–1553. doi:10.1002/cssc.201601816
Chanut, N., Wiersum, A. D., Lee, U. H., Hwang, Y. K., Ragon, F., Chevreau, H., et al. (2016). Observing the effects of shaping on gas adsorption in metal-organic frameworks. Eur. J. Inorg. Chem. 2016 (27), 4416–4423. doi:10.1002/ejic.201600410
Chen, H., and Sholl, D. S. (2007). Examining the accuracy of ideal adsorbed solution theory without curve-fitting using transition matrix Monte Carlo simulations. Langmuir 23 (11), 6431–6437. doi:10.1021/la700351c
Choi, S., Gray, M. L., and Jones, C. W. (2011). Amine-tethered solid adsorbents coupling high adsorption capacity and regenerability for CO2 capture from ambient air. ChemSusChem 4 (5), 628–635. doi:10.1002/cssc.201000355
Choi, S., Watanabe, T., Bae, T.-H., Sholl, D. S., and Jones, C. W. (2012). Modification of the Mg/DOBDC MOF with amines to enhance CO2 adsorption from ultradilute gases. J. Phys. Chem. Lett. 3 (9), 1136–1141. doi:10.1021/jz300328j
Choi, W., Min, K., Kim, C., Ko, Y. S., Jeon, J. W., Seo, H., et al. (2016). Epoxide-functionalization of polyethyleneimine for synthesis of stable carbon dioxide adsorbent in temperature swing adsorption. Nat. Commun. 7 (1), 12640. doi:10.1038/ncomms12640
Chowdhury, P., Bikkina, C., Meister, D., Dreisbach, F., and Gumma, S. (2009). Comparison of adsorption isotherms on Cu-BTC metal organic frameworks synthesized from different routes. Microporous Mesoporous Mater. 117 (1), 406–413. doi:10.1016/j.micromeso.2008.07.029
Coudert, F.-X., and Fuchs, A. H. (2016). Computational characterization and prediction of metal–organic framework properties. Coord. Chem. Rev. 307, 211–236. doi:10.1016/j.ccr.2015.08.001
Culp, J. T., Smith, M. R., Bittner, E., and Bockrath, B. (2008). Hysteresis in the physisorption of CO2 and N2 in a flexible pillared layer nickel cyanide. J. Am. Chem. Soc. 130 (37), 12427–12434. doi:10.1021/ja802474b
Danaci, D., Bui, M., Mac Dowell, N., and Petit, C. (2020). Exploring the limits of adsorption-based CO2 capture using MOFs with PVSA – from molecular design to process economics. Mol. Syst. Des. Eng. 5 (1), 212–231. doi:10.1039/C9ME00102F
Danaci, D., Webley, P. A., and Petit, C. (2021). Guidelines for techno-economic analysis of adsorption processes. Front. Chem. Eng. 2. doi:10.3389/fceng.2020.602430
Dantas, T. L. P., Luna, F. M. T., Silva, I. J., de Azevedo, D. C. S., Grande, C. A., Rodrigues, A. E., et al. (2011). Carbon dioxide–nitrogen separation through adsorption on activated carbon in a fixed bed. Chem. Eng. J. 169 (1), 11–19. doi:10.1016/j.cej.2010.08.026
Denayer, J. F., Souverijns, W., Jacobs, P. A., Martens, J. A., and Baron, G. V. (1998). High-temperature low-pressure adsorption of branched C5−C8 alkanes on zeolite beta, ZSM-5, ZSM-22, zeolite Y, and mordenite. J. Phys. Chem. B 102 (23), 4588–4597. doi:10.1021/jp980674k
Deutz, S., and Bardow, A. (2021). Life-cycle assessment of an industrial direct air capture process based on temperature–vacuum swing adsorption. Nat. Energy 6 (2), 203–213. doi:10.1038/s41560-020-00771-9
Díaz, K., Garrido, L., López-González, M., del Castillo, L. F., and Riande, E. (2010). CO2 transport in polysulfone membranes containing zeolitic imidazolate frameworks as determined by permeation and PFG NMR techniques. Macromolecules 43 (1), 316–325. doi:10.1021/ma902303e
Ding, M., and Jiang, H.-L. (2020). Improving water stability of metal–organic frameworks by a general surface hydrophobic polymerization. CCS Chem. 3 (8), 2740–2748. doi:10.31635/ccschem.020.202000515
Du, H., Williams, C. T., Ebner, A. D., and Ritter, J. A. (2010). In situ FTIR spectroscopic analysis of carbonate transformations during adsorption and desorption of CO2 in K-promoted HTlc. Chem. Mater. 22 (11), 3519–3526. doi:10.1021/cm100703e
Eic, M., and Ruthven, D. M. (1988). A new experimental technique for measurement of intracrystalline diffusivity. Zeolites 8 (1), 40–45. doi:10.1016/S0144-2449(88)80028-9
Elfving, J., Bajamundi, C., Kauppinen, J., and Sainio, T. (2017). Modelling of equilibrium working capacity of PSA, TSA and TVSA processes for CO2 adsorption under direct air capture conditions. J. CO2 Util. 22, 270–277. doi:10.1016/j.jcou.2017.10.010
Elfving, J., Kauppinen, J., Jegoroff, M., Ruuskanen, V., Järvinen, L., and Sainio, T. (2021). Experimental comparison of regeneration methods for CO2 concentration from air using amine-based adsorbent. Chem. Eng. J. 404, 126337. doi:10.1016/j.cej.2020.126337
Embs, J. P., Juranyi, F., and Hempelmann, R. (2010). Introduction to quasielastic neutron scattering, Introd. Quasielastic Neutron Scatt. 224 (1-2), 5–32. doi:10.1524/zpch.2010.6090
Erans, M., Sanz-Pérez, E. S., Hanak, D. P., Clulow, Z., Reiner, D. M., and Mutch, G. A. (2022). Direct air capture: Process technology, techno-economic and socio-political challenges. Energy & Environ. Sci. 15 (4), 1360–1405. doi:10.1039/D1EE03523A
Espinal, L., Poster, D. L., Wong-Ng, W., Allen, A. J., and Green, M. L. (2013). Measurement, standards, and data needs for CO2 capture materials: A critical review. Environ. Sci. Technol. 47 (21), 11960–11975. doi:10.1021/es402622q
Evans, J. D., Bon, V., Senkovska, I., and Kaskel, S. (2021). A universal standard archive file for adsorption data. Langmuir 37 (14), 4222–4226. doi:10.1021/acs.langmuir.1c00122
Farmahini, A. H., Krishnamurthy, S., Friedrich, D., Brandani, S., and Sarkisov, L. (2018). From crystal to adsorption column: Challenges in multiscale computational screening of materials for adsorption separation processes. Industrial Eng. Chem. Res. 57 (45), 15491–15511. doi:10.1021/acs.iecr.8b03065
Farmahini, A. H., Krishnamurthy, S., Friedrich, D., Brandani, S., and Sarkisov, L. (2021). Performance-based screening of porous materials for carbon capture. Chem. Rev. 121 (17), 10666–10741. doi:10.1021/acs.chemrev.0c01266
Fayaz, M., and Sayari, A. (2017). Long-term effect of steam exposure on CO2 capture performance of amine-grafted silica. ACS Appl. Mater. Interfaces 9 (50), 43747–43754. doi:10.1021/acsami.7b15463
Fayemiwo, K. A., Chiarasumran, N., Nabavi, S. A., Loponov, K. N., Manović, V., Benyahia, B., et al. (2019). Eco-friendly fabrication of a highly selective amide-based polymer for CO2 capture. Industrial Eng. Chem. Res. 58 (39), 18160–18167. doi:10.1021/acs.iecr.9b02347
Franchi, R. S., Harlick, P. J. E., and Sayari, A. (2005). Applications of pore-expanded mesoporous silica. 2. Development of a high-capacity, water-tolerant adsorbent for CO2. Industrial Eng. Chem. Res. 44 (21), 8007–8013. doi:10.1021/ie0504194
Fraux, G., Boutin, A., Fuchs, A. H., and Coudert, F.-X. (2018). On the use of the IAST method for gas separation studies in porous materials with gate-opening behavior. Adsorption 24 (3), 233–241. doi:10.1007/s10450-018-9942-5
Fröhlich, D., Henninger, S. K., and Janiak, C. (2014). Multicycle water vapour stability of microporous breathing MOF aluminium isophthalate CAU-10-H. Dalton Trans. 43 (41), 15300–15304. doi:10.1039/C4DT02264E
Gaikwad, S., Kim, Y., Gaikwad, R., and Han, S. (2021). Enhanced CO2 capture capacity of amine-functionalized MOF-177 metal organic framework. J. Environ. Chem. Eng. 9 (4), 105523. doi:10.1016/j.jece.2021.105523
Galarneau, A., Cambon, H., Di Renzo, F., Ryoo, R., Choi, M., and Fajula, F. (2003). Microporosity and connections between pores in SBA-15 mesostructured silicas as a function of the temperature of synthesis. New J. Chem. 27 (1), 73–79. doi:10.1039/B207378C
Garberoglio, G., Skoulidas, A. I., and Johnson, J. K. (2005). Adsorption of gases in metal organic materials: Comparison of simulations and experiments. J. Phys. Chem. B 109 (27), 13094–13103. doi:10.1021/jp050948l
Gelles, T., and Rezaei, F. (2020). Diffusion kinetics of CO2 in amine-impregnated MIL-101, alumina, and silica adsorbents. AIChE J. 66 (1), e16785. doi:10.1002/aic.16785
Geng, Z., Xiao, Q., Lv, H., Li, B., Wu, H., Lu, Y., et al. (2016). One-step synthesis of microporous carbon monoliths derived from biomass with high nitrogen doping content for highly selective CO2 capture. Sci. Rep. 6 (1), 30049. doi:10.1038/srep30049
Gibson, J. A. A., Mangano, E., Shiko, E., Greenaway, A. G., Gromov, A. V., Lozinska, M. M., et al. (2016). Adsorption materials and processes for carbon capture from gas-fired power plants: AMPGas. Industrial Eng. Chem. Res. 55 (13), 3840–3851. doi:10.1021/acs.iecr.5b05015
Grande, C. A., Blom, R., Spjelkavik, A., Moreau, V., and Payet, J. (2017). Life-cycle assessment as a tool for eco-design of metal-organic frameworks (MOFs). Sustain. Mater. Technol. 14, 11–18. doi:10.1016/j.susmat.2017.10.002
Grenier, , Meunier, F., Gray, P. G., Kärger, J., Xu, Z., and Ruthven, D. M. (1994). Diffusion of methanol in NaX crystals: Comparison of i.r., ZLC, and PFG-n.m.r. measurements. Zeolites 14 (4), 242–249. doi:10.1016/0144-2449(94)90091-4
Gu, J., Lin, J., Smith, A. J., Soontaranon, S., Rugmai, S., Kongmark, C., et al. (2022). Towards understanding mesopore formation in zeolite Y crystals using alkaline additives via in situ small-angle X-ray scattering. Microporous Mesoporous Mater. 338, 111867. doi:10.1016/j.micromeso.2022.111867
Hadjiivanov, K. I., Panayotov, D. A., Mihaylov, M. Y., Ivanova, E. Z., Chakarova, K. K., Andonova, S. M., et al. (2021). Power of infrared and Raman spectroscopies to characterize metal-organic frameworks and investigate their interaction with guest molecules. Chem. Rev. 121 (3), 1286–1424. doi:10.1021/acs.chemrev.0c00487
Hamieh, T., Ali Ahmad, A., Roques-Carmes, T., and Toufaily, J. (2020). New approach to determine the surface and interface thermodynamic properties of H-β-zeolite/rhodium catalysts by inverse gas chromatography at infinite dilution. Sci. Rep. 10 (1), 20894. doi:10.1038/s41598-020-78071-1
Heidari, A., Boleydei, H., Rohani, A., Lu, H. R., and Younesi, H. (2022). Integrating life cycle assessment and life cycle costing using TOPSIS to select sustainable biomass-based -carbonaceous adsorbents for CO2 capture. J. Clean. Prod. 357, 131968. doi:10.1016/j.jclepro.2022.131968
Herzog, H. (2022). “Direct air capture,” in Greenhouse gas removal technologies. Editors M. Bui, and N. Mac Dowell (The Royal Society of Chemistry).
Heydari-Gorji, A., Belmabkhout, Y., and Sayari, A. (2011). Degradation of amine-supported CO2 adsorbents in the presence of oxygen-containing gases. Microporous Mesoporous Mater. 145 (1), 146–149. doi:10.1016/j.micromeso.2011.05.010
Heydari-Gorji, A., and Sayari, A. (2012). Thermal, oxidative, and CO2-induced degradation of supported polyethylenimine adsorbents. Industrial Eng. Chem. Res. 51 (19), 6887–6894. doi:10.1021/ie3003446
Ho, M. T., Allinson, G. W., and Wiley, D. E. (2008). Reducing the cost of CO2 capture from flue gases using pressure swing adsorption. Industrial Eng. Chem. Res. 47 (14), 4883–4890. doi:10.1021/ie070831e
Hobday, C. L., Krause, S., Rogge, S. M. J., Evans, J. D., and Bunzen, H. (2021). Perspectives on the influence of crystal size and morphology on the properties of porous framework materials. Front. Chem. 9, 772059. doi:10.3389/fchem.2021.772059
Hong, S.-M., Jang, E., Dysart, A. D., Pol, V. G., and Lee, K. B. (2016). CO2 capture in the sustainable wheat-derived activated microporous carbon compartments. Sci. Rep. 6 (1), 34590. doi:10.1038/srep34590
Hu, X., Brandani, S., Benin, A. I., and Willis, R. R. (2015). Development of a semiautomated zero length column technique for carbon capture applications: Rapid capacity ranking of novel adsorbents. Industrial Eng. Chem. Res. 54 (26), 6772–6780. doi:10.1021/acs.iecr.5b00513
Hu, X., Brandani, S., Benin, A. I., and Willis, R. R. (2018). Testing the stability of novel adsorbents for carbon capture applications using the zero length column technique. Chem. Eng. Res. Des. 131, 406–413. doi:10.1016/j.cherd.2018.01.023
Hu, Z., Wang, Y., Shah, B. B., and Zhao, D. (2019). CO2 capture in metal–organic framework adsorbents: An engineering perspective. Adv. Sustain. Syst. 3 (1), 1800080. doi:10.1002/adsu.201800080
Hunter-Sellars, E., Saenz-Cavazos, P. A., Houghton, A. R., McIntyre, S. R., Parkin, I. P., and Williams, D. R. (2020a). Sol–Gel synthesis of high-density zeolitic imidazolate framework monoliths via ligand assisted methods: Exceptional porosity, hydrophobicity, and applications in vapor adsorption. Adv. Funct. Mater. n/a, 2008357. doi:10.1002/adfm.202008357
Hunter-Sellars, E., Tee, J. J., Parkin, I. P., and Williams, D. R. (2020b). Adsorption of volatile organic compounds by industrial porous materials: Impact of relative humidity. Microporous Mesoporous Mater. 298, 110090. doi:10.1016/j.micromeso.2020.110090
Iacomi, P., Lee, U. H., Valekar, A. H., Chang, J.-S., and Llewellyn, P. L. (2019). Investigating the effect of alumina shaping on the sorption properties of promising metal–organic frameworks. RSC Adv. 9 (13), 7128–7135. doi:10.1039/C9RA00534J
Iacomi, P., and Llewellyn, P. L. (2019). pyGAPS: a Python-based framework for adsorption isotherm processing and material characterisation. Adsorption 25 (8), 1533–1542. doi:10.1007/s10450-019-00168-5
IPCC (2018). “Framing and context,” in Global warming of 1.5°C: IPCC special report on impacts of global warming of 1.5°C above pre-industrial levels in context of strengthening response to climate change, sustainable development, and efforts to eradicate poverty. (Cambridge: Cambridge University Press), 49–92.
IUPAC (2021). Standardized reporting of gas adsorption isotherms. [Online]. Available: https://iupac.org/project/2021-016-1-024/.
Jagiello, J., Kenvin, J., Celzard, A., and Fierro, V. (2019). Enhanced resolution of ultra micropore size determination of biochars and activated carbons by dual gas analysis using N2 and CO2 with 2D-NLDFT adsorption models. Carbon 144, 206–215. doi:10.1016/j.carbon.2018.12.028
Jagiello, J., and Kenvin, J. (2022). NLDFT adsorption models for zeolite porosity analysis with particular focus on ultra-microporous zeolites using O2 and H2. J. Colloid Interface Sci. 625, 178–186. doi:10.1016/j.jcis.2022.06.044
Jahandar Lashaki, M., Khiavi, S., and Sayari, A. (2019). Stability of amine-functionalized CO2 adsorbents: A multifaceted puzzle. Chem. Soc. Rev. 48 (12), 3320–3405. doi:10.1039/C8CS00877A
Jo, H., Lee, W. R., Kim, N. W., Jung, H., Lim, K. S., Kim, J. E., et al. (2017). Fine-tuning of the carbon dioxide capture capability of diamine-grafted metal–organic framework adsorbents through amine functionalization. ChemSusChem 10 (3), 541–550. doi:10.1002/cssc.201601203
Jones, C. R., Olfe-Kräutlein, B., Naims, H., and Armstrong, K. (2017). The social acceptance of carbon dioxide utilisation: A review and research agenda. Front. Energy Res. 5. doi:10.3389/fenrg.2017.00011
Kärger, J., Avramovska, M., Freude, D., Haase, J., Hwang, S., and Valiullin, R. (2021). Pulsed field gradient NMR diffusion measurement in nanoporous materials. Adsorption 27 (3), 453–484. doi:10.1007/s10450-020-00290-9
Khurana, M., and Farooq, S. (2016). Simulation and optimization of a 6-step dual-reflux VSA cycle for post-combustion CO2 capture. Chem. Eng. Sci. 152, 507–515. doi:10.1016/j.ces.2016.06.033
Kiani, A., Jiang, K., and Feron, P. (2020). Techno-economic assessment for CO2 capture from air using a conventional liquid-based absorption process. Front. Energy Res. 8. doi:10.3389/fenrg.2020.00092
Kim, K.-M., Oh, H.-T., Lim, S.-J., Ho, K., Park, Y., and Lee, C.-H. (2016). Adsorption equilibria of water vapor on zeolite 3A, zeolite 13X, and dealuminated Y zeolite. J. Chem. Eng. Data 61 (4), 1547–1554. doi:10.1021/acs.jced.5b00927
Kim, M., Cahill, J. F., Su, Y., Prather, K. A., and Cohen, S. M. (2012). Postsynthetic ligand exchange as a route to functionalization of ‘inert’ metal–organic frameworks. Chem. Sci. 3 (1), 126–130. doi:10.1039/C1SC00394A
Knaebel, K. S. (1995). For your next separation consider adsorption. Chem. Eng. Technol. 102, 92–102.
Köck, E.-M., Kogler, M., Bielz, T., Klötzer, B., and Penner, S. (2013). In situ FT-IR spectroscopic study of CO2 and CO adsorption on Y2O3, ZrO2, and yttria-stabilized ZrO2. J. Phys. Chem. C 117 (34), 17666–17673. doi:10.1021/jp405625x
Kozbial, A., Li, Z., Conaway, C., McGinley, R., Dhingra, S., Vahdat, V., et al. (2014). Study on the surface energy of graphene by contact angle measurements. Langmuir 30 (28), 8598–8606. doi:10.1021/la5018328
Krishnamurthy, S., Blom, R., Ferrari, M. C., and Brandani, S. (2020). Adsorption and diffusion of CO2 in CPO-27–Ni beads. Adsorption 26 (5), 711–721. doi:10.1007/s10450-019-00162-x
Kruk, M., and Cao, L. (2007). Pore size tailoring in large-pore SBA-15 silica synthesized in the presence of hexane. Langmuir 23 (13), 7247–7254. doi:10.1021/la0702178
Kruteva, M. (2021). Dynamics studied by quasielastic neutron scattering (QENS). Adsorption 27 (5), 875–889. doi:10.1007/s10450-020-00295-4
Lawson, S., Snarzyk, M., Hanify, D., Rownaghi, A. A., and Rezaei, F. (2020). Development of 3D-printed polymer-MOF monoliths for CO2 adsorption. Industrial Eng. Chem. Res. 59 (15), 7151–7160. doi:10.1021/acs.iecr.9b05445
Lee, S.-K., Han, S. W., Cha, G.-Y., Park, J. M., Park, H., Ryoo, R., et al. (2022). Base-type nitrogen doping in zeolite-templated carbon for enhancement of carbon dioxide sorption. J. CO2 Util. 62, 102084. doi:10.1016/j.jcou.2022.102084
Lee, S.-Y., and Park, S.-J. (2015). A review on solid adsorbents for carbon dioxide capture. J. Industrial Eng. Chem. 23, 1–11. doi:10.1016/j.jiec.2014.09.001
Leonzio, G., Fennell, P. S., and Shah, N. (2022a). A comparative study of different sorbents in the context of direct air capture (DAC): Evaluation of key performance indicators and comparisons. Appl. Sci. 12 (5), 2618. doi:10.3390/app12052618
Leonzio, G., Mwabonje, O., Fennell, P. S., and Shah, N. (2022b). Environmental performance of different sorbents used for direct air capture. Sustain. Prod. Consum. 32, 101–111. doi:10.1016/j.spc.2022.04.004
Levy, C. L., Matthews, G. P., Laudone, G. M., Gribble, C. M., Turner, A., Ridgway, C. J., et al. (2015). Diffusion and tortuosity in porous functionalized calcium carbonate. Industrial Eng. Chem. Res. 54 (41), 9938–9947. doi:10.1021/acs.iecr.5b02362
Liang, W., Li, L., Hou, J., Shepherd, N. D., Bennett, T. D., D'Alessandro, D. M., et al. (2018). Linking defects, hierarchical porosity generation and desalination performance in metal–organic frameworks. Chem. Sci. 9 (14), 3508–3516. doi:10.1039/C7SC05175A
Lin, J.-B., Nguyen, T. T. T., Vaidhyanathan, R., Burner, J., Taylor, J. M., Durekova, H., et al. (2021). A scalable metal-organic framework as a durable physisorbent for carbon dioxide capture. Science 374 (6574), 1464–1469. doi:10.1126/science.abi7281
Liu, J., Liu, X., Sun, Y., Sun, C., Liu, H., Stevens, L. A., et al. (2018). High density and super ultra-microporous-activated carbon macrospheres with high volumetric capacity for CO2 capture. Adv. Sustain. Syst. 2 (2), 1700115. doi:10.1002/adsu.201700115
Liu, Q., Xiong, B., Shi, J., Tao, M., He, Y., and Shi, Y. (2014). Enhanced tolerance to flue gas contaminants on carbon dioxide capture using amine-functionalized multiwalled carbon nanotubes. Energy & Fuels 28 (10), 6494–6501. doi:10.1021/ef501614m
Liu, R.-S., Shi, X.-D., Wang, C.-T., Gao, Y.-Z., Xu, S., Hao, G.-P., et al. (2021). Advances in post-combustion CO2 capture by physical adsorption: From materials innovation to separation practice. ChemSusChem 14 (6), 1428–1471. doi:10.1002/cssc.202002677
Liu, Y., Ye, Q., Shen, M., Shi, J., Chen, J., Pan, H., et al. (2011). Carbon dioxide capture by functionalized solid amine sorbents with simulated flue gas conditions. Environ. Sci. Technol. 45 (13), 5710–5716. doi:10.1021/es200619j
Liu, Z.-l., Teng, Y., Zhang, K., Cao, Y., and Pan, W.-p. (2013). CO2 adsorption properties and thermal stability of different amine-impregnated MCM-41 materials. J. Fuel Chem. Technol. 41 (4), 469–475. doi:10.1016/S1872-5813(13)60025-0
Llewellyn, P. L., and Maurin, G. (2005). Gas adsorption microcalorimetry and modelling to characterise zeolites and related materials. Comptes Rendus Chim. 8 (3), 283–302. doi:10.1016/j.crci.2004.11.004
Loganathan, S., Tikmani, M., Edubilli, S., Mishra, A., and Ghoshal, A. K. (2014). CO2 adsorption kinetics on mesoporous silica under wide range of pressure and temperature. Chem. Eng. J. 256, 1–8. doi:10.1016/j.cej.2014.06.091
Low, M.-Y., Barton, L. V., Pini, R., and Petit, C. (2023). Analytical review of the current state of knowledge of adsorption materials and processes for direct air capture. Chem. Eng. Res. Des. 189, 745–767. doi:10.1016/j.cherd.2022.11.040
Lozinska, M. M., Mangano, E., Mowat, J. P. S., Shepherd, A. M., Howe, R. F., Thompson, S. P., et al. (2012). Understanding carbon dioxide adsorption on univalent cation forms of the flexible zeolite rho at conditions relevant to carbon capture from flue gases. J. Am. Chem. Soc. 134 (42), 17628–17642. doi:10.1021/ja3070864
Lu, S., Han, R., Wang, H., Song, C., Ji, N., Lu, X., et al. (2022). Three birds with one stone: Designing a novel binder-free monolithic zeolite pellet for wet VOC gas adsorption. Chem. Eng. J. 448, 137629. doi:10.1016/j.cej.2022.137629
Madden, D. G., O’Nolan, D., Rampal, N., Babu, R., Çamur, C., Al Shakhs, A. N., et al. (2022). Densified HKUST-1 monoliths as a route to high volumetric and gravimetric hydrogen storage capacity. J. Am. Chem. Soc. 144 (30), 13729–13739. doi:10.1021/jacs.2c04608
Mangano, E., Brandani, S., Ferrari, M. C., Ahn, H., Friedrich, D., Lozinska, M. L., et al. (2013). Efficient and rapid screening of novel adsorbents for carbon capture in the UK IGSCC project. Energy Procedia 37, 40–47. doi:10.1016/j.egypro.2013.05.083
Maring, B. J., and Webley, P. A. (2013). A new simplified pressure/vacuum swing adsorption model for rapid adsorbent screening for CO2 capture applications. Int. J. Greenh. Gas Control 15, 16–31. doi:10.1016/j.ijggc.2013.01.009
Marx, D., Joss, L., Hefti, M., Pini, R., and Mazzotti, M. (2013). The role of water in adsorption-based CO2 capture systems. Energy Procedia 37, 107–114. doi:10.1016/j.egypro.2013.05.090
Mason, J. A., McDonald, T. M., Bae, T.-H., Bachman, J. E., Sumida, K., Dutton, J. J., et al. (2015). Application of a high-throughput analyzer in evaluating solid adsorbents for post-combustion carbon capture via multicomponent adsorption of CO2, N2, and H2O. J. Am. Chem. Soc. 137 (14), 4787–4803. doi:10.1021/jacs.5b00838
Mason, J. A., Sumida, K., Herm, Z. R., Krishna, R., and Long, J. R. (2011). Evaluating metal–organic frameworks for post-combustion carbon dioxide capture via temperature swing adsorption. Energy & Environ. Sci. 4 (8), 3030–3040. doi:10.1039/C1EE01720A
Meador, M. A. B., Alemán, C. R., Hanson, K., Ramirez, N., Vivod, S. L., Wilmoth, N., et al. (2015). Polyimide aerogels with amide cross-links: A low cost alternative for mechanically strong polymer aerogels. ACS Appl. Mater. Interfaces 7 (2), 1240–1249. doi:10.1021/am507268c
Menard, D., Py, X., and Mazet, N. (2007). Activated carbon monolith of high thermal conductivity for adsorption processes improvement: Part B. Thermal regeneration. Chem. Eng. Process. Process Intensif. 46 (6), 565–572. doi:10.1016/j.cep.2006.C7EE02110K07.013
Meng, Y., Jiang, J., Aihemaiti, A., Ju, T., Gao, Y., Liu, J., et al. (2019). Feasibility of CO2 capture from O2-containing flue gas using a poly(ethylenimine)-functionalized sorbent: Oxidative stability in long-term operation. ACS Appl. Mater. Interfaces 11 (37), 33781–33791. doi:10.1021/acsami.9b08048
Mfoumou, C. M., Mignard, S., and Belin, T. (2018). The preferential adsorption sites of H2O on adsorption sites of CO2 at low temperature onto NaX and BaX zeolites. Adsorpt. Sci. Technol. 36 (5-6), 1246–1259. doi:10.1177/0263617418762494
Middelkoop, V., Coenen, K., Schalck, J., Van Sint Annaland, M., and Gallucci, F. (2019). 3D printed versus spherical adsorbents for gas sweetening. Chem. Eng. J. 357, 309–319. doi:10.1016/j.cej.2018.09.130
Min, K., Choi, W., Kim, C., and Choi, M. (2018). Oxidation-stable amine-containing adsorbents for carbon dioxide capture. Nat. Commun. 9 (1), 726. doi:10.1038/s41467-018-03123-0
Mohammad, S., Fitzgerald, J., Robinson, R. L., and Gasem, K. A. M. (2009). Experimental uncertainties in volumetric methods for measuring equilibrium adsorption. Energy & Fuels 23 (5), 2810–2820. doi:10.1021/ef8011257
Moore, J. K., Marti, R. M., Guiver, M. D., Du, N., Conradi, M. S., and Hayes, S. E. (2018). CO2 adsorption on PIMs studied with 13C NMR spectroscopy. J. Phys. Chem. C 122 (8), 4403–4408. doi:10.1021/acs.jpcc.7b12312
Moschetta, E. G., Sakwa-Novak, M. A., Greenfield, J. L., and Jones, C. W. (2015). Post-grafting amination of alkyl halide-functionalized silica for applications in catalysis, adsorption, and 15N NMR spectroscopy. Langmuir 31 (7), 2218–2227. doi:10.1021/la5046817
Mu, B., and Walton, K. S. (2011). Thermal analysis and heat capacity study of metal–organic frameworks. J. Phys. Chem. C 115 (46), 22748–22754. doi:10.1021/jp205538a
Mueller, R., Kanungo, R., Kiyono-Shimobe, M., Koros, W. J., and Vasenkov, S. (2012). Diffusion of methane and carbon dioxide in carbon molecular sieve membranes by multinuclear pulsed field gradient NMR. Langmuir 28 (27), 10296–10303. doi:10.1021/la301674k
Myers, A. L., and Prausnitz, J. M. (1965). Thermodynamics of mixed-gas adsorption. AIChE J. 11 (1), 121–127. doi:10.1002/aic.690110125
Nezam, I., Xie, J., Golub, K. W., Carneiro, J., Olsen, K., Ping, E. W., et al. (2021). Chemical kinetics of the autoxidation of poly(ethylenimine) in CO2 sorbents. ACS Sustain. Chem. Eng. 9 (25), 8477–8486. doi:10.1021/acssuschemeng.1c01367
Nguyen, H. G. T., Espinal, L., van Zee, R. D., Thommes, M., Toman, B., Hudson, M. S. L., et al. (2018). A reference high-pressure CO2 adsorption isotherm for ammonium ZSM-5 zeolite: Results of an interlaboratory study. Adsorption 24 (6), 531–539. doi:10.1007/s10450-018-9958-x
Nguyen, H. G. T., Horn, J. C., Bleakney, M., Siderius, D. W., and Espinal, L. (2019). Understanding material characteristics through signature traits from helium pycnometry. Langmuir 35 (6), 2115–2122. doi:10.1021/acs.langmuir.8b03731
Nguyen, T. T. T., Lin, J.-B., Shimizu, G. K. H., and Rajendran, A. (2022). Separation of CO2 and N2 on a hydrophobic metal organic framework CALF-20. Chem. Eng. J. 442, 136263. doi:10.1016/j.cej.2022.136263
Nguyen, T. X., Cohaut, N., Bae, J.-S., and Bhatia, S. K. (2008). New method for atomistic modeling of the microstructure of activated carbons using hybrid reverse Monte Carlo simulation. Langmuir 24 (15), 7912–7922. doi:10.1021/la800351d
Nie, L., Mu, Y., Jin, J., Chen, J., and Mi, J. (2018). Recent developments and consideration issues in solid adsorbents for CO2 capture from flue gas. Chin. J. Chem. Eng. 26 (11), 2303–2317. doi:10.1016/j.cjche.2018.07.012
Nugent, P., Belmabkhout, Y., Burd, S. D., Cairns, A. J., Luebke, R., Forrest, K., et al. (2013). Porous materials with optimal adsorption thermodynamics and kinetics for CO2 separation. Nature 495 (7439), 80–84. doi:10.1038/nature11893
Nuhnen, A., and Janiak, C. (2020). A practical guide to calculate the isosteric heat/enthalpy of adsorption via adsorption isotherms in metal–organic frameworks, MOFs. Dalton Trans. 49 (30), 10295–10307. doi:10.1039/D0DT01784A
Ojwang, D. O., Grins, J., and Svensson, G. (2018). The adsorption kinetics of CO2 on copper hexacyanoferrate studied by thermogravimetric analysis. Microporous Mesoporous Mater. 272, 70–78. doi:10.1016/j.micromeso.2018.06.019
Oschatz, M., and Antonietti, M. (2018). A search for selectivity to enable CO2 capture with porous adsorbents. Energy & Environ. Sci. 11 (1), 57–70. doi:10.1039/C7EE02110K
Osterrieth, J. W. M., Rampersad, J., Madden, D., Rampal, N., Skoric, L., Connolly, B., et al. (2022). How reproducible are surface areas calculated from the BET equation? Adv. Mater. 34 (27), 2201502. doi:10.1002/adma.202201502
Pai, K. N., Prasad, V., and Rajendran, A. (2020). Generalized, adsorbent-agnostic, artificial neural network framework for rapid simulation, optimization, and adsorbent screening of adsorption processes. Industrial Eng. Chem. Res. 59 (38), 16730–16740. doi:10.1021/acs.iecr.0c02339
Panda, D., Kulkarni, V., and Singh, S. K. (2023). Evaluation of amine-based solid adsorbents for direct air capture: A critical review. React. Chem. Eng. 8 (1), 10–40. doi:10.1039/D2RE00211F
Papadopoulos, G. K., Jobic, H., and Theodorou, D. N. (2004). Transport diffusivity of N2 and CO2 in silicalite: Coherent quasielastic neutron scattering measurements and molecular dynamics simulations. J. Phys. Chem. B 108 (34), 12748–12756. doi:10.1021/jp049265g
Papageorgiou, G. Z., Palani, A., Gilliopoulos, D., Triantafyllidis, K. S., and Bikiaris, D. N. (2013). Mechanical properties and crystallization of high-density polyethylene composites with mesostructured cellular silica foam. J. Therm. Analysis Calorim. 113 (3), 1651–1665. doi:10.1007/s10973-013-3223-z
Park, J., Howe, J. D., and Sholl, D. S. (2017). How reproducible are isotherm measurements in metal–organic frameworks? Chem. Mater. 29 (24), 10487–10495. doi:10.1021/acs.chemmater.7b04287
Patel, H. A., Byun, J., and Yavuz, C. T. (2017). Carbon dioxide capture adsorbents: Chemistry and methods. ChemSusChem 10 (7), 1303–1317. doi:10.1002/cssc.201601545
Patel, H. (2019). Fixed-bed column adsorption study: A comprehensive review. Appl. Water Sci. 9 (3), 45. doi:10.1007/s13201-019-0927-7
Pini, R. (2014). Interpretation of net and excess adsorption isotherms in microporous adsorbents. Microporous Mesoporous Mater. 187, 40–52. doi:10.1016/j.micromeso.2013.12.005
Pini, R., Siderius, D. W., and Siepmann, J. I. (2022). Preface to adsorption and diffusion in porous materials special issue: Equilibrium adsorption data for energy and environmental applications. J. Chem. Eng. Data 67 (7), 1597–1598. doi:10.1021/acs.jced.2c00382
Piscopo, C. G., and Loebbecke, S. (2020). Strategies to enhance carbon dioxide capture in metal-organic frameworks. ChemPlusChem 85 (3), 538–547. doi:10.1002/cplu.202000072
Plaza, M., Pevida, C., Arias, B., Fermoso, J., Arenillas, A., Rubiera, F., et al. (2008). Application of thermogravimetric analysis to the evaluation of aminated solid sorbents for CO2 capture. J. Therm. Analysis Calorim. 92 (2), 601–606. doi:10.1007/s10973-007-8493-x
Pokhrel, J., Bhoria, N., Anastasiou, S., Tsoufis, T., Gournis, D., Romanos, G., et al. (2018). CO2 adsorption behavior of amine-functionalized ZIF-8, graphene oxide, and ZIF-8/graphene oxide composites under dry and wet conditions. Microporous Mesoporous Mater. 267, 53–67. doi:10.1016/j.micromeso.2018.03.012
Principe, I. A., and Fletcher, A. J. (2020). Adsorption selectivity of CO2 over CH4, N2 and H2 in melamine–resorcinol–formaldehyde xerogels. Adsorption 26 (5), 723–735. doi:10.1007/s10450-020-00203-w
Pugh, S. M., and Forse, A. C. (2023). Nuclear magnetic resonance studies of carbon dioxide capture. J. Magnetic Reson. 346, 107343. doi:10.1016/j.jmr.2022.107343
Querejeta, N., García, S., Álvarez-Gutiérrez, N., Rubiera, F., and Pevida, C. (2019). Measuring heat capacity of activated carbons for CO2 capture. J. CO2 Util. 33, 148–156. doi:10.1016/j.jcou.2019.05.018
Raganati, F., Chirone, R., and Ammendola, P. (2020). CO2 capture by temperature swing adsorption: Working capacity as affected by temperature and CO2 partial pressure. Industrial Eng. Chem. Res. 59 (8), 3593–3605. doi:10.1021/acs.iecr.9b04901
Raganati, F., Miccio, F., and Ammendola, P. (2021). Adsorption of carbon dioxide for post-combustion capture: A review. Energy & Fuels 35 (16), 12845–12868. doi:10.1021/acs.energyfuels.1c01618
Rahman, S., Arami-Niya, A., Yang, X., Xiao, G., Li, G., and May, E. F. (2020). Temperature dependence of adsorption hysteresis in flexible metal organic frameworks. Commun. Chem. 3 (1), 186. doi:10.1038/s42004-020-00429-3
Rajagopalan, A. K., Avila, A. M., and Rajendran, A. (2016). Do adsorbent screening metrics predict process performance? A process optimisation based study for post-combustion capture of CO2. Int. J. Greenh. Gas Control 46, 76–85. doi:10.1016/j.ijggc.2015.12.033
Rajagopalan, A. K., and Rajendran, A. (2018). The effect of nitrogen adsorption on vacuum swing adsorption based post-combustion CO2 capture. Int. J. Greenh. Gas Control 78, 437–447. doi:10.1016/j.ijggc.2018.09.002
Rajendran, A., Kariwala, V., and Farooq, S. (2008). Correction procedures for extra-column effects in dynamic column breakthrough experiments. Chem. Eng. Sci. 63 (10), 2696–2706. doi:10.1016/j.ces.2008.02.023
Rajendran, A., Maruyama, R. T., Landa, H. O. R., and Seidel-Morgenstern, A. (2020). Modelling binary non-linear chromatography using discrete equilibrium data. Adsorption 26 (7), 973–987. doi:10.1007/s10450-020-00220-9
Ravikovitch, P. I., and Neimark, A. V. (2005). Diffusion-controlled hysteresis. Adsorption 11 (1), 265–270. doi:10.1007/s10450-005-5935-2
Rezaei, F., and Jones, C. W. (2013). Stability of supported amine adsorbents to SO2 and NOx in postcombustion CO2 capture. 1. Single-component adsorption. Industrial Eng. Chem. Res. 52 (34), 12192–12201. doi:10.1021/ie4019116
Rezaei, F., and Jones, C. W. (2014). Stability of supported amine adsorbents to SO2 and NOx in postcombustion CO2 capture. 2. Multicomponent adsorption. Industrial Eng. Chem. Res. 53 (30), 12103–12110. doi:10.1021/ie502024z
Rittig, F., Coe, C. G., and Zielinski, J. M. (2003). Pure and multicomponent gas diffusion within zeolitic adsorbents: Pulsed field gradient NMR analysis and model development. J. Phys. Chem. B 107 (19), 4560–4566. doi:10.1021/jp0219451
Rouquerol, J., Llewellyn, P., and Rouquerol, F. (2007). “Is the BET equation applicable to microporous adsorbents?” in Characterization of porous solids VII - proceedings of the 7th international symposium on the characterization of porous solids (COPS-VII). Aix-en-Provence, France 26, 49–56.
Roztocki, K., Rauche, M., Bon, V., Kaskel, S., Brunner, E., and Matoga, D. (2021). Combining in situ techniques (XRD, IR, and 13C NMR) and gas adsorption measurements reveals CO2-induced structural transitions and high CO2/CH4 selectivity for a flexible metal–organic framework JUK-8. ACS Appl. Mater. Interfaces 13 (24), 28503–28513. doi:10.1021/acsami.1c07268
Sáenz Cavazos, P. A., Díaz-Ramírez, M. L., Hunter-Sellars, E., McIntyre, S. R., Lima, E., Ibarra, I. A., et al. (2021). Fluorinated MIL-101 for carbon capture utilisation and storage: Uptake and diffusion studies under relevant industrial conditions. RSC Adv. 11 (22), 13304–13310. doi:10.1039/D1RA01118A
Said, R. B., Kolle, J. M., Essalah, K., Tangour, B., and Sayari, A. (2020). A unified approach to CO2–amine reaction mechanisms. ACS Omega 5 (40), 26125–26133. doi:10.1021/acsomega.0c03727
Samanta, A., Zhao, A., Shimizu, G. K. H., Sarkar, P., and Gupta, R. (2012). Post-combustion CO2 capture using solid sorbents: A review. Industrial Eng. Chem. Res. 51 (4), 1438–1463. doi:10.1021/ie200686q
Sanz-Pérez, E. S., Murdock, C. R., Didas, S. A., and Jones, C. W. (2016). Direct capture of CO2 from ambient air. Chem. Rev. 116 (19), 11840–11876. doi:10.1021/acs.chemrev.6b00173
Sayari, A., Belmabkhout, Y., and Da’na, E. (2012a). CO2 deactivation of supported amines: Does the nature of amine matter? Langmuir 28 (9), 4241–4247. doi:10.1021/la204667v
Sayari, A., and Belmabkhout, Y. (2010). Stabilization of amine-containing CO2 adsorbents: Dramatic effect of water vapor. J. Am. Chem. Soc. 132 (18), 6312–6314. doi:10.1021/ja1013773
Sayari, A., Heydari-Gorji, A., and Yang, Y. (2012b). CO2-Induced degradation of amine-containing adsorbents: Reaction products and pathways. J. Am. Chem. Soc. 134 (33), 13834–13842. doi:10.1021/ja304888a
Schlumberger, C., and Thommes, M. (2021). Characterization of hierarchically ordered porous materials by physisorption and mercury porosimetry—a tutorial review. Adv. Mater. Interfaces 8 (4), 2002181. doi:10.1002/admi.202002181
Sendi, M., Bui, M., Mac Dowell, N., and Fennell, P. (2022). Geospatial analysis of regional climate impacts to accelerate cost-efficient direct air capture deployment. One Earth 5 (10), 1153–1164. doi:10.1016/j.oneear.2022.09.003
Shade, D., Bout, B. W. S., Sholl, D. S., and Walton, K. S. (2022). Opening the toolbox: 18 experimental techniques for measurement of mixed gas adsorption. Industrial Eng. Chem. Res. 61 (6), 2367–2391. doi:10.1021/acs.iecr.1c03756
Shekhah, O., Belmabkhout, Y., Chen, Z., Guillerm, V., Cairns, A., Adil, K., et al. (2014). Made-to-order metal-organic frameworks for trace carbon dioxide removal and air capture. Nat. Commun. 5 (1), 4228. doi:10.1038/ncomms5228
Shi, X., Lin, Y., and Chen, X. (2022). Development of sorbent materials for direct air capture of CO2. MRS Bull. 47 (4), 405–415. doi:10.1557/s43577-022-00320-7
Shi, X., Xiao, H., Azarabadi, H., Song, J., Wu, X., Chen, X., et al. (2020). Sorbents for the direct capture of CO2 from ambient air. Angew. Chem. Int. Ed. 59 (18), 6984–7006. doi:10.1002/anie.201906756
Shimon, D., Chen, C.-H., Lee, J. J., Didas, S. A., Sievers, C., Jones, C. W., et al. (2018). 15N solid state NMR spectroscopic study of surface amine groups for carbon capture: 3-Aminopropylsilyl grafted to SBA-15 mesoporous silica. Environ. Sci. Technol. 52 (3), 1488–1495. doi:10.1021/acs.est.7b04555
Si, W., Yang, B., Yu, Q., Lei, L., and Zhu, J. (2019). Deactivation kinetics of polyethylenimine-based adsorbents used for the capture of low concentration CO2. ACS Omega 4 (6), 11237–11244. doi:10.1021/acsomega.9b00792
Siderius, D., Shen, V., Johnson, R., and Van Zee, R. D. (2015). NIST/ARPA-E database of novel and emerging adsorbent materials. doi:10.18434/T43882
Siegelman, R. L., Kim, E. J., and Long, J. R. (2021). Porous materials for carbon dioxide separations. Nat. Mater. 20 (8), 1060–1072. doi:10.1038/s41563-021-01054-8
Sing, K. S. W., and Williams, R. T. (2004). Physisorption hysteresis loops and the characterization of nanoporous materials. Adsorpt. Sci. Technol. 22 (10), 773–782. doi:10.1260/0263617053499032
Sinha, A., Darunte, L. A., Jones, C. W., Realff, M. J., and Kawajiri, Y. (2017). Systems design and economic analysis of direct air capture of CO2 through temperature vacuum swing adsorption using MIL-101(Cr)-PEI-800 and mmen-Mg2(dobpdc) MOF adsorbents. Industrial Eng. Chem. Res. 56 (3), 750–764. doi:10.1021/acs.iecr.6b03887
Sinha, A., and Realff, M. J. (2019). A parametric study of the techno-economics of direct CO2 air capture systems using solid adsorbents. AIChE J. 65 (7), e16607. doi:10.1002/aic.16607
Sircar, S. (2001). Applications of gas separation by adsorption for the future. Adsorpt. Sci. Technol. 19 (5), 347–366. doi:10.1260/0263617011494222
Sircar, S., Wu, H., Li, J., and Lueking, A. D. (2011). Effect of time, temperature, and kinetics on the hysteretic adsorption–desorption of H2, Ar, and N2 in the metal–organic framework Zn2(bpdc)2(bpee). Langmuir 27 (23), 14169–14179. doi:10.1021/la202842m
Somerset, V. S., Petrik, L. F., White, R. A., Klink, M. J., Key, D., and Iwuoha, E. (2004). The use of X-ray fluorescence (XRF) analysis in predicting the alkaline hydrothermal conversion of fly ash precipitates into zeolites. Talanta 64 (1), 109–114. doi:10.1016/j.talanta.2003.10.059
Son, W.-J., Choi, J.-S., and Ahn, W.-S. (2008). Adsorptive removal of carbon dioxide using polyethyleneimine-loaded mesoporous silica materials. Microporous Mesoporous Mater. 113 (1), 31–40. doi:10.1016/j.micromeso.2007.10.049
Sreenivasulu, B., Sreedhar, I., Suresh, P., and Raghavan, K. V. (2015). Development trends in porous adsorbents for carbon capture. Environ. Sci. Technol. 49 (21), 12641–12661. doi:10.1021/acs.est.5b03149
Stedman, J. R., Vincent, K. J., Campbell, G. W., Goodwin, J. W. L., and Downing, C. E. H. (1997). New high resolution maps of estimated background ambient NOx and NO2 concentrations in the U.K. Atmos. Environ. 31 (21), 3591–3602. doi:10.1016/S1352-2310(97)00159-3
Subramanian Balashankar, V., Rajagopalan, A. K., de Pauw, R., Avila, A. M., and Rajendran, A. (2019). Analysis of a batch adsorber analogue for rapid screening of adsorbents for postcombustion CO2 capture. Industrial Eng. Chem. Res. 58 (8), 3314–3328. doi:10.1021/acs.iecr.8b05420
Subraveti, S. G., Pai, K. N., Rajagopalan, A. K., Wilkins, N. S., Rajendran, A., Jayaraman, A., et al. (2019). Cycle design and optimization of pressure swing adsorption cycles for pre-combustion CO2 capture. Appl. Energy 254, 113624. doi:10.1016/j.apenergy.2019.113624
Subraveti, S. G., Roussanaly, S., Anantharaman, R., Riboldi, L., and Rajendran, A. (2022). How much can novel solid sorbents reduce the cost of post-combustion CO2 capture? A techno-economic investigation on the cost limits of pressure–vacuum swing adsorption. Appl. Energy 306, 117955. doi:10.1016/j.apenergy.2021.117955
Sujan, A. R., Kumar, D. R., Sakwa-Novak, M., Ping, E. W., Hu, B., Park, S. J., et al. (2019). Poly(glycidyl amine)-loaded SBA-15 sorbents for CO2 capture from dilute and ultradilute gas mixtures. ACS Appl. Polym. Mater. 1 (11), 3137–3147. doi:10.1021/acsapm.9b00788
Taddei, M., and Petit, C. (2021). Engineering metal–organic frameworks for adsorption-based gas separations: From process to atomic scale. Mol. Syst. Des. Eng. 6 (11), 841–875. doi:10.1039/D1ME00085C
Takaishi, T., and Sensui, Y. (1963). Thermal transpiration effect of hydrogen, rare gases and methane. Trans. Faraday Soc. 59 (0), 2503–2514. doi:10.1039/TF9635902503
Teng, Y., Liu, Z., Xu, G., and Zhang, K. (2017). Desorption kinetics and mechanisms of CO2 on amine-based mesoporous silica materials. Energies 10 (1), 115. doi:10.3390/en10010115
Thommes, M., Kaneko, K., Neimark, A. V., Olivier, J. P., Rodriguez-Reinoso, F., Rouquerol, J., et al. (2015). Physisorption of gases, with special reference to the evaluation of surface area and pore size distribution (IUPAC Technical Report). Pure Appl. Chem. 87 (9-10), 1051–1069. doi:10.1515/pac-2014-1117
Thompson, J. A., and Zones, S. I. (2020). Binary- and pure-component adsorption of CO2, H2O, and C6H14 on SSZ-13. Industrial Eng. Chem. Res. 59 (40), 18151–18159. doi:10.1021/acs.iecr.0c03480
Thouchprasitchai, N., Pintuyothin, N., and Pongstabodee, S. (2018). Optimization of CO2 adsorption capacity and cyclical adsorption/desorption on tetraethylenepentamine-supported surface-modified hydrotalcite. J. Environ. Sci. 65, 293–305. doi:10.1016/j.jes.2017.02.015
Tian, T., Velazquez-Garcia, J., Bennett, T. D., and Fairen-Jimenez, D. (2015). Mechanically and chemically robust ZIF-8 monoliths with high volumetric adsorption capacity. J. Mater. Chem. A 3 (6), 2999–3005. doi:10.1039/C4TA05116E
Tian, T., Zeng, Z., Vulpe, D., Casco, M. E., Divitini, G., Midgley, P. A., et al. (2018). A sol–gel monolithic metal–organic framework with enhanced methane uptake. Nat. Mater. 17 (2), 174–179. doi:10.1038/nmat5050
Ünveren, E. E., Monkul, B. Ö., Sarıoğlan, Ş., Karademir, N., and Alper, E. (2017). Solid amine sorbents for CO2 capture by chemical adsorption: A review. Petroleum 3 (1), 37–50. doi:10.1016/j.petlm.2016.11.001
van Paasen, S., Infantino, M., Yao, J., Leenders, S. H. A. M., van de Graaf, J. M., Klingler, A., et al. (2021). Development of the solid sorbent technology for post combustion CO2 capture towards commercial prototype. Int. J. Greenh. Gas Control 109, 103368. doi:10.1016/j.ijggc.2021.103368
Vilarrasa-García, E., Cecilia, J. A., Moya, E. M., Cavalcante, C. L., Azevedo, D. C., and Rodríguez-Castellón, E. (2015). Low cost pore expanded SBA-15 functionalized with amine groups applied to CO2 adsorption. Materials 8 (5), 2495–2513. doi:10.3390/ma8052495
Walton, K. S. (2019). 110th anniversary: Commentary: Perspectives on adsorption of complex mixtures. Industrial Eng. Chem. Res. 58 (37), 17100–17105. doi:10.1021/acs.iecr.9b04243
Walton, K. S., and Sholl, D. S. (2015). Predicting multicomponent adsorption: 50 years of the ideal adsorbed solution theory. AIChE J. 61 (9), 2757–2762. doi:10.1002/aic.14878
Wang, C., and Okubayashi, S. (2019). Polyethyleneimine-crosslinked cellulose aerogel for combustion CO2 capture. Carbohydr. Polym. 225, 115248. doi:10.1016/j.carbpol.2019.115248
Wang, J.-Y., Mangano, E., Brandani, S., and Ruthven, D. M. (2021). A review of common practices in gravimetric and volumetric adsorption kinetic experiments. Adsorption 27 (3), 295–318. doi:10.1007/s10450-020-00276-7
Wang, L., Gandorfer, M., Selvam, T., and Schwieger, W. (2018). Determination of faujasite-type zeolite thermal conductivity from measurements on porous composites by laser flash method. Mater. Lett. 221, 322–325. doi:10.1016/j.matlet.2018.03.157
Wang, T., Lackner, K. S., and Wright, A. (2011). Moisture swing sorbent for carbon dioxide capture from ambient air. Environ. Sci. Technol. 45 (15), 6670–6675. doi:10.1021/es201180v
Wang, X., and Song, C. (2020). Carbon capture from flue gas and the atmosphere. A Perspect. 8. doi:10.3389/fenrg.2020.560849
Webley, P. A., and Danaci, D. (2019). “CO2 capture by adsorption processes,” in Carbon capture and storage. Editors D. Reiner, M. Bui, and N. Mac Dowell (The Royal Society of Chemistry).
Wiersum, A. D., Chang, J.-S., Serre, C., and Llewellyn, P. L. (2013). An adsorbent performance indicator as a first step evaluation of novel sorbents for gas separations: Application to metal–organic frameworks. Langmuir 29 (10), 3301–3309. doi:10.1021/la3044329
Wilkins, N. S., Rajendran, A., and Farooq, S. (2021). Dynamic column breakthrough experiments for measurement of adsorption equilibrium and kinetics. Adsorption 27 (3), 397–422. doi:10.1007/s10450-020-00269-6
Wilkins, N. S., Sawada, J. A., and Rajendran, A. (2020). Measurement of competitive CO2 and H2 O adsorption on zeolite 13X for post-combustion CO2 capture. Adsorption 26 (5), 765–779. doi:10.1007/s10450-020-00199-3
Wilkins, N. S., Sawada, J. A., and Rajendran, A. (2022). Quantitative microscale dynamic column breakthrough apparatus for measurement of unary and binary adsorption equilibria on milligram quantities of adsorbents. Industrial Eng. Chem. Res. 61 (20), 7032–7051. doi:10.1021/acs.iecr.2c00115
Wu, B., Liu, F.-Q., Luo, S.-W., Zhang, L.-Q., and Zou, F. (2021). Carbonaceous materials-supported polyethylenimine with high thermal conductivity: A promising adsorbent for CO2 capture. Compos. Sci. Technol. 208, 108781. doi:10.1016/j.compscitech.2021.108781
Wu, H., Thibault, C. G., Wang, H., Cychosz, K. A., Thommes, M., and Li, J. (2016). Effect of temperature on hydrogen and carbon dioxide adsorption hysteresis in an ultramicroporous MOF. Microporous Mesoporous Mater. 219, 186–189. doi:10.1016/j.micromeso.2015.08.005
Wu, J., Zhu, X., Chen, Y., Wang, R., and Ge, T. (2022). The analysis and evaluation of direct air capture adsorbents on the material characterization level. Chem. Eng. J. 450, 137958. doi:10.1016/j.cej.2022.137958
Xie, L.-H., Xu, M.-M., Liu, X.-M., Zhao, M.-J., and Li, J.-R. (2020). Hydrophobic metal–organic frameworks: Assessment, construction, and diverse applications. Adv. Sci. 7 (4), 1901758. doi:10.1002/advs.201901758
Xu, H., Prasetyo, L., Do, D. D., and Nicholson, D. (2020). The Henry constant and isosteric heat at zero loading for adsorption on energetically heterogeneous solids absolute versus excess. Chem. Eng. J. 395, 125035. doi:10.1016/j.cej.2020.125035
Xu, X., Song, C., Miller, B. G., and Scaroni, A. W. (2005). Adsorption separation of carbon dioxide from flue gas of natural gas-fired boiler by a novel nanoporous “molecular basket” adsorbent. Fuel Process. Technol. 86 (14), 1457–1472. doi:10.1016/j.fuproc.2005.01.002
Yang, M., Ma, C., Xu, M., Wang, S., and Xu, L. (2019). Recent advances in CO2 adsorption from air: A review. Curr. Pollut. Rep. 5 (4), 272–293. doi:10.1007/s40726-019-00128-1
Yeon, J. S., Lee, W. R., Kim, N. W., Jo, H., Lee, H., Song, J. H., et al. (2015). Homodiamine-functionalized metal–organic frameworks with a MOF-74-type extended structure for superior selectivity of CO2 over N2. J. Mater. Chem. A 3 (37), 19177–19185. doi:10.1039/C5TA02357B
Zárate, A., Peralta, R. A., Bayliss, P. A., Howie, R., Sánchez-Serratos, M., Carmona-Monroy, P., et al. (2016). CO2 capture under humid conditions in NH2-MIL-53(Al): The influence of the amine functional group. RSC Adv. 6 (12), 9978–9983. doi:10.1039/C5RA26517G
Zhang, C., Gee, J. A., Sholl, D. S., and Lively, R. P. (2014). Crystal-size-dependent structural transitions in nanoporous crystals: Adsorption-induced transitions in ZIF-8. J. Phys. Chem. C 118 (35), 20727–20733. doi:10.1021/jp5081466
Zhang, F., Yan, Y., Yang, H., Meng, Y., Yu, C., Tu, B., et al. (2005). Understanding effect of wall structure on the hydrothermal stability of mesostructured silica SBA-15. J. Phys. Chem. B 109 (18), 8723–8732. doi:10.1021/jp044632+
Zhang, H., Goeppert, A., Olah, G. A., and Prakash, G. K. S. (2017). Remarkable effect of moisture on the CO2 adsorption of nano-silica supported linear and branched polyethylenimine. J. CO2 Util. 19, 91–99. doi:10.1016/j.jcou.2017.03.008
Zhang, W., Liu, H., Sun, Y., Cakstins, J., Sun, C., and Snape, C. E. (2016). Parametric study on the regeneration heat requirement of an amine-based solid adsorbent process for post-combustion carbon capture. Appl. Energy 168, 394–405. doi:10.1016/j.apenergy.2016.01.049
Zhang, X., and Du, T. (2022). Study of rice husk ash derived MCM-41-type materials on pore expansion, Al incorporation, PEI impregnation, and CO2 adsorption. Korean J. Chem. Eng. 39 (3), 736–759. doi:10.1007/s11814-021-0904-3
Zhang, X., Wang, Z., Cheng, M., Wu, X., Zhan, N., and Xu, J. (2021). Long-term ambient SO2 concentration and its exposure risk across China inferred from OMI observations from 2005 to 2018. Atmos. Res. 247, 105150. doi:10.1016/j.atmosres.2020.105150
Zhang, X., Zheng, X., Zhang, S., Zhao, B., and Wu, W. (2012). AM-TEPA impregnated disordered mesoporous silica as CO2 capture adsorbent for balanced adsorption–desorption properties. Industrial Eng. Chem. Res. 51 (46), 15163–15169. doi:10.1021/ie300180u
Keywords: adsorption, adsorbent, carbon capture, DAC, post-combustion capture, characterization, MOFs
Citation: Saenz Cavazos PA, Hunter-Sellars E, Iacomi P, McIntyre SR, Danaci D and Williams DR (2023) Evaluating solid sorbents for CO2 capture: linking material properties and process efficiency via adsorption performance. Front. Energy Res. 11:1167043. doi: 10.3389/fenrg.2023.1167043
Received: 15 February 2023; Accepted: 05 July 2023;
Published: 27 July 2023.
Edited by:
Eugenio Quaranta, University of Bari Aldo Moro, ItalyReviewed by:
Shuzhuang Sun, Queen’s University Belfast, United KingdomCarlo Pastore, National Research Council (CNR), Italy
Copyright © 2023 Saenz Cavazos, Hunter-Sellars, Iacomi, McIntyre, Danaci and Williams. This is an open-access article distributed under the terms of the Creative Commons Attribution License (CC BY). The use, distribution or reproduction in other forums is permitted, provided the original author(s) and the copyright owner(s) are credited and that the original publication in this journal is cited, in accordance with accepted academic practice. No use, distribution or reproduction is permitted which does not comply with these terms.
*Correspondence: Paola A. Saenz Cavazos, cGFvbGEuc2FlbnotY2F2YXpvczE3QGltcGVyaWFsLmFjLnVr; Daryl R. Williams, ZC5yLndpbGxpYW1zQGltcGVyaWFsLmFjLnVr