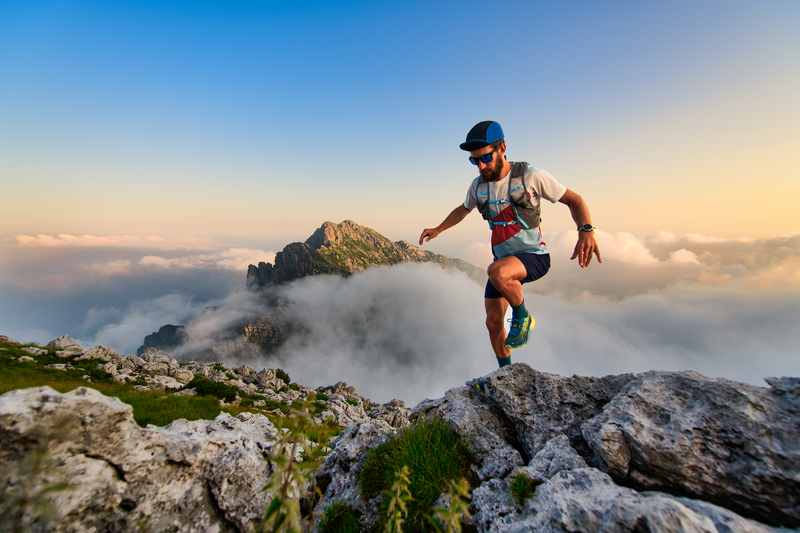
95% of researchers rate our articles as excellent or good
Learn more about the work of our research integrity team to safeguard the quality of each article we publish.
Find out more
REVIEW article
Front. Energy Res. , 07 June 2023
Sec. Nuclear Energy
Volume 11 - 2023 | https://doi.org/10.3389/fenrg.2023.1157394
Nuclear fusion technologies have re-gained momentum in the last decade thanks to their disruptive potential in different fields, such as energy production and space propulsion, and to new technological developments, especially high temperature superconductor tapes, which allow overcoming previous performance or design limits. To date, reviews of recent nuclear fusion designs are lacking. Therefore, this paper aims at giving a comprehensive overview of nuclear fusion concepts for industrial applications with a focus on the private sector. The designs are classified according to the three leading concepts for plasma confinement, namely, magnetic confinement, inertial confinement and magneto-inertial confinement. The working principles of the main devices are described in detail to highlight strengths and weaknesses of the different designs. The importance of the public sector on private projects is discussed. The technological maturity is estimated, and the main criticalities for each project are identified. Finally, the geographical distribution of the companies (or public institutions) pursuing the design of fusion devices for commercial applications is reported.
Nuclear fusion has been investigated throughout the years since the first theoretical works on stars core physics in the 20s and 30s (Atkinson and Houtermans, 1929; Oliphant et al., 1934; Bethe, 1939). The first machines to replicate fusion reactions on the Earth were built during the 50s (Barbarino, 2020), and both research and achievements have progressed steadily until now (Figure 1). Despite the physics and engineering complexity behind fusion reactors, nuclear fusion has always attracted mankind thanks to the following key features: it achieves extremely high power densities; it relies on abundant fuel (see Section 2.5.2 for details on fuel supply); it does not emit any greenhouse gas during operations and shows a very low carbon footprint on a life-cycle basis (Banacloche et al., 2020); it is intrinsically safe thanks to the absence of chain reactions (i.e., the reactants are different from the reaction products, so a runaway of the fusion process is not possible in case of loss of reaction control); the generation of high-level waste can be minimized by carefully selecting low-activation materials when designing the reactor (Zucchetti et al., 2013); and fusion power plants can in principle be designed for baseload production and with load-following capabilities (Segantin et al., 2019). Since the energy sector contributes to approximately 75% of the World greenhouse gas emissions (Ge et al., 2020), the introduction of a high-energy density, low-carbon energy source in the energy mix would be an additional tool to meet the Climate Action goals (SDG 13) and to improve the access to sustainable and modern energy (SDG 7) (UN, 2015). Nicholas et al. (2021) showed indeed the important role that fusion energy can have in the energy mix if commercial fusion becomes available in the second half of the 21st century [i.e., according to the timeline defined by the DEMO project (Romanelli et al., 2012)]. Nevertheless, commercial fusion reactors may enter the energy mix sooner than expected thanks to private companies and start-ups.
FIGURE 1. Triple product achieved by different fusion devices along the years. The green region depicts the triple product required to achieve ignition. Data from: NIF (approximated) (Bishop, 2022), ITER (Mukhovatov et al., 2003), JT-60U (Fujita et al., 1998; Kishimoto et al., 2005), W7-X (Wolf R. et al., 2019), IPA (Slough et al., 2011), TFTR (Hawryluk et al., 1998), JET (JET Team, 1992), Alcator-C (Greenwald et al., 1984), PLT (Grove et al., 1977), TFR (TFR Group, 1985), ST (Stodiek, 1985), T-3 (Peacock et al., 1969), ZETA (Thonemann et al., 1958).
The goal of this paper is to provide a comprehensive, up-to-date review of fusion machine designs in light of the tremendous acceleration that the nuclear fusion field has experienced in the last decade. The attempts to develop nuclear fusion technologies is a driving force for research and industries in several fields, from structural materials to magnet technology, from energy production to space propulsion. The entry of the private sector into commercial fusion projects has greatly contributed to this acceleration, making nuclear fusion a technology of interest even in the short-to-medium term.
To date, available reviews on nuclear fusion reactors are outdated (Ribe, 1975; Post, 1976; Dabiri, 1988), focus on specific confinement approaches (Monsler et al., 1981; Ongena et al., 2016) or specific reactor systems (Muroga et al., 2002; Ihli et al., 2008), and are limited to specific regions (Andreani and Gasparotto, 2002; Morley et al., 2006; Tanaka, 2006). Given this, there is a need for a comprehensive framework of fusion reactors that takes into account the recent developments in the field, many of which are privately-funded, and on a global scale. This need has been acknowledged also by the International Atomic Energy Agency (IAEA), which has identified and mapped fusion devices worldwide (IAEA, 2021). In light of this, the present paper aims to provide an updated overview of recent fusion reactor projects on a global scale, with a focus on recent developments in the field. Each of these projects has advantages and different challenges to be overcome, related to plasma confinement and stability, machine engineering, and materials performances, to mention a few. An overview on nuclear fusion fundamentals, plasma confinement approaches and on the most relevant devices developed in the past is provided before introducing the different projects. Many of these companies make use of speculative or widely-extrapolative approaches to try to simplify physics or engineering problems. These approaches are described here for information and comparison, although it is beyond the scope of this paper to examine all the claims in detail. For specific discussions on advanced physics concepts and confinement schemes the reader is referred through the text to well-established works in literature.
The paper is structured as follows: Section 2 introduces the main applications of fusion energy and the challenges that are common among the different designs; Section 3 provides a review of the approaches to exploit fusion energy and the designs of fusion devices under development by private companies (or public-private partnerships); Section 4 discusses extensively the main achievements, the open issues and the technological maturity of the design described in Section 3.
Nuclear fusion is a nuclear reaction in which two nuclei overcome the repulsive electrostatic force that keeps them separated and form one or more new nuclei and subatomic particles. This reaction releases energy if the starting nuclei are relatively light (up to 56Fe and 62Ni) and therefore have a high binding energy per nucleon. The most studied nuclear fusion reactions for energy applications are those employing as reactants 1 a plasma of the isotopes of hydrogen (deuterium and tritium), 3He or 11B:
For these reactions to be sustained, the reactants must be in the plasma state. The fusion cross section for the aforementioned reactions is reported in Figure 2 as a function of the energy.2
FIGURE 2. Experimental cross sections (σ) for the most relevant fusion reactions. Most of the projects are based on D-T reactions, which show the highest cross section at low energy. The x-axis shows the energy in the laboratory frame. Libraries: ENDF/B-VIII.0 (D-D, D-T, D-3He, 3He-3He) (Brown et al., 2018) and EXFOR (p-11B) (Otuka et al., 2014). The (p-11B) cross section is the result of the interpolation of the experimental data in the EXFOR library.
The concept of plasma is fundamental in fusion engineering, being the state of matter in which the reacting fuel is present inside a fusion device. More precisely, the name of plasma is attributed to an ionized gas, with a behavior dominated by long-range electric and magnetic interaction (conversely, the most relevant interactions for a normal gas are short-range collision). A plasma can be obtained from a gas heating it up to temperatures in which the average kinetic energy of particles is comparable with ionization energy, obtaining a mixture of electrons and ions. Indeed kinetic thermodynamics states a clear relation between the average kinetic energy of particles Ek and the temperature of the system, in the form:
where kB is the Boltzmann constant. Because of the proportionality of E and T, in plasma physics it is customary to measure the temperature using an energetic unit, the electron volt (i.e., the energy gained by a particle with unitary charge accelerating through a potential of 1 V). In the following, temperatures are always indicated in eV or in its multiples. The state of plasma constitutes the largest fraction of matter in the Universe. Stellar coronas, interplanetary plasma, the ionosphere, electric arcs are just some examples of this abundance. Clearly, differences are present between all these examples of plasma, exactly as solids differ from each other, still being in the same state of aggregation. The two main parameters for the classification of plasmas are the particle density, n, and the plasma temperature, T. These parameters can vary on a surprisingly large range, around 25 orders of magnitudes for particle density and 7 for temperature. Plasmas of interest for fusion aims present a particle density of around 1020 m−3 for magnetic confinement or 1031m−3 for inertial confinement, with a similar temperature of about 10 keV.
However, considering the most studied D-T reaction, a particular condition must be reached for a steady state reactor: the power heating of alpha particles must be large enough to balance bremsstrahlung and thermal conduction losses, excluding any external power contribution. This condition is called ignition and its limits on p, T are set by the Lawson Criterion3 (Freidberg, 2007):
where Kα is a constant related to alpha power heating, KB and Kk are constants related to Bremmstrahlung and conduction losses, p is the plasma pressure, and τE is the energy confinement time. The Lawson criterion sets quantitative requirements to the plasma pressure and the energy confinement for a self-sustaining plasma (i.e., the energy released by the fusion reactions maintains the plasma hot enough for the reactions to keep occurring). The right hand side of Eq. 8 is indeed related to self-heating from the alpha particle produced by fusion reactions and plasma losses. An extension to the Lawson criterion can be derived by working out Eq. 8 and introducing the triple product nTτE. For instance, for D-T fusion in the 10–20 keV range (Wesson and Campbell, 2011):
to achieve ignition which is the operating regime of interest for fusion power plants. The triple product establishes therefore a link between plasma conditions (n and T), confinement (τE), and the capabilities of the plasma to be self-sustained, resulting in a useful metric to analyse and compare designs exploiting different confinement approaches (as shown in Section 3).
Nuclear fusion technologies find their main applications in energy production. The goal is to produce energy using the heat generated by nuclear fusion reactions. So far nuclear fission has been successfully used to produce energy without CO2 or other pollutants emissions, but still faces issues such as the production of high-level (nuclear) waste, the use of uranium that is not renewable, and a negative perception/reputation with part of the population (Kim et al., 2014). These problems are of less concern for fusion reactors. First generation fusion reactors would likely exploit deuterium and tritium, which can be considered as a renewable fuel, and are being designed not to produce high-level waste. Deuterium can indeed be obtained from seawater and therefore is a practically unlimited element, while tritium can be produced from lithium in the reactor itself (Section 2.5.2). Compared to the other energy sources, nuclear fusion has many advantages: no greenhouse gas or other pollutant emission during operations, no chain reactions, baseload production and load-following capabilities. These aspects make nuclear fusion extremely promising from the point of view of energy production, but it is not exempt from concerns and issues (Section 2.5).
Two useful figures of merit employed when assessing the power balance in fusion systems are the (physics) gain factor Q and the engineering gain factor Qe (Freidberg, 2007). Q is related to the Lawson criterion, and is defined as the ratio of the net thermal power produced by the fusion reactions to the external heating power required to keep the plasma in steady-state. For D-T fusion,
The technologies proposed to produce energy from fusion reactions are based on three main approaches: magnetic confinement fusion (MCF, Section 3.1), inertial confinement fusion (ICF, Section 3.2) and magnetized target fusion (MTF, Section 3.3).
Nuclear fusion has a tremendous potential for space propulsion. Chemical propulsion has been successfully used in the past decades for short-distance missions, but it is impractical for farther, manned missions. Nuclear (fission) propulsion has been investigated as an advanced solution during the Rover/NERVA program (Robbins, 1991) and the use of nuclear thermal propulsion is considered the most suitable propulsion system for a manned mission to Mars (Drake et al., 2010). Eventually, even nuclear fission will become impractical for long distance missions (e.g., beyond Mars or outside the Solar System). Nuclear fusion could then provide a more advanced solution to long distance travels (Genta and Kezerashvili, 2020; Aime et al., 2021). D-T fusion can be exploited to achieve higher specific power and higher exhaust velocity than nuclear fission propulsion by exploiting known fusion processes and available technologies (Slough, 2018). Despite being less investigated than nuclear fission propulsion, nuclear fusion propulsion has been studied since the 50s within the Project Orion (Dyson, 2002), and more recently by the NASA Innovative Advanced Concept program (Slough et al., 2012; Slough, 2018).
A nuclear fusion rocket is substantially different from a nuclear fusion reactor. The large mass and the need for a high power removal of a terrestrial fusion reactor make the MCF and the ICF hardly implementable for space propulsion applications. Rocket mass is indeed a hard constraint in space propulsion, and power exhaust is a critical issue in space due to the absence of an efficient thermal sink. MTF is therefore the leading approach for space propulsion (Adams et al., 2003), even though alternative designs based on field-reversed configuration have been proposed as well (Razin et al., 2014).
The main applications for nuclear fusion are undoubtedly energy production and space propulsion. Most of the research and the investments in the last decades focused on these two fields. Nevertheless, there are several other applications of nuclear fusion that have yet to be fully explored. Two notable examples include nuclear waste processing (e.g., from fission or medicine) and the production of radioisotopes for medical applications. High energy neutrons from fusion reactions have the capability to transmute long-lived radioactive isotopes into shorter-lived or non-radioactive isotopes, reducing the volume and hazard of high-level nuclear waste. The reader can find conceptual design for nuclear waste treatment in (Parish and Davidson, 1980; Stacey et al., 2002; Şahin and Übeyli, 2004; Mehlhorn et al., 2008).
Fusion reactions can be also used to produce a wide range of isotopes for medical imaging and therapy. These isotopes play a vital role in the diagnosis and treatment of cancer and other diseases. Despite being still in early stages of research, this application of nuclear fusion promises to be used as an additional source of these isotopes together with fission reactors and particle accelerators. The reader can find additional information in (Cipiti and Kulcinski, 2005; Leung et al., 2018; Agostini et al., 2021).
There are other few options as side applications for nuclear fusion. We refer to side applications to stress that those are not considered as core business [despite radical innovations in fusion technology or the growth of new markets may change this paradigm (Griffiths et al., 2022)] but as possible additional revenue streams for fusion power plants (Alhamdan et al., 2022). Low-temperature applications of fusion energy include desalination and district heating, while high-temperature applications include hydrogen production and process heat production (Griffiths et al., 2022).
This subsection briefly presents the challenges that are common to all the confinement approaches, applications, and devices.
As presented before, to obtain an energy gain and a self-sustained system from fusion, plasma temperature and particle density must be tuned in the desired range; the maintaining of such conditions by a suitable system of forces is called plasma confinement. Ions must overcome coulomb repulsion by means of their kinetic energy, to exploit the strong nuclear force that tends to bring nucleons closer together. Unfortunately, the strong nuclear force becomes dominant only on a very short range, introducing the necessity of high particle energy (e.g., high plasma temperatures); even by increasing the temperature to maximize the reaction cross section (see Figure 2), the probability to obtain a fusion interaction remains dramatically low. A large enough time must be given to the system for interaction. This can be achieved by confining the plasma by external means to slow down the spontaneous expansion and cooling of the plasma itself. The confinement time τE, is consequently introduced in the Lawson criterion (Eq. 8). While in nature gravity represents the confinement force for fusing plasma (e.g., in stars), such strategy cannot be exploited by fusion reactors due to the enormous masses of the reacting systems that would be required. Consequently the main approaches previously mentioned (MCF, ICF, MTF) have been developed to substitute gravity.
Magnetic confinement relies on magnetic fields to confine the plasma, starting from the consideration that the trajectory of charged particles can be modified by the Lorentz force, while the inertial confinement exploits the inertia principle to compress the system thanks to an implosion of the fuel. Considering MCF, the system should be confined in a stable condition in which the main plasma parameters are the desired ones, but still allowing for efficient helium ash removal from the core plasma to avoid excessive fuel dilution (i.e., a pathway for particle exhaust must be present). Since the plasma is a highly-conductive fluid, the magnetic field lines and the plasma itself are tightly coupled and moves together (in the ideal case), allowing to manipulate the plasma by acting on the magnetic field, and vice versa. Unfortunately, small perturbations naturally appear in the plasma column, and could evolve and diverge, quickly destroying the plasma column itself and in the worst scenarios leading to surface damages to the reactor walls. As far as the inertial confinement approach is concerned, stability is mostly related to the symmetry of the shock wave that triggers the implosion of the fuel. Even small perturbations in the front of the shock wave can lead to ineffective compression, with a consequent degradation of plasma density and temperature. The physics of instabilities is beyond the aim of the present paper, but dealing with them is one of the more challenging issues in fusion research.
Any device that exploits fusion reactions requires fuel (and refueling). Fuel can be provided as a gas or as a solid pellet, usually stored and processed in loco. For p-11B fusion, both the reactants are abundant, but this is not the case for D-T or D-3He fusion. Deuterium can be easily extracted from water, while tritium is a radioactive isotope with a half-life of 12.3 years (meaning that hardly any T is available in nature), and 3He is an extremely rare element on the Earth.
Current reserves of tritium are estimated as 30–40 kg (Kovari et al., 2017; Pearson et al., 2018). As a means of comparison, a 3,000 MWth fusion reactor would consume approximately 170 kg of tritium per full power year. D-T fusion devices are therefore being designed to produce (breed) tritium in the reactor itself by exploiting the nuclear reactions between neutrons and lithium. The component devoted to tritium breeding is the breeding blanket. Each D-T fusion reaction produces a neutron, which travels almost freely through the plasma until reaching the structure surrounding the vacuum chamber, where the breeding blanket is located. Most of the neutrons are slowed down in the blanket, releasing energy in the form of heat and eventually being absorbed by the blanket materials. Materials with a high content of lithium are used to breed tritium according to the reactions in Eqs 10, 11:
where the former reaction is exothermic, whereas the latter reaction requires a neutron of energy greater than 2.5 MeV to occur. Available reserves of lithium are enough to sustain fusion energy development at any reasonable rate for the next decades (Meschini, 2021). A D-T fusion device that produces more tritium than the amount it burns is said to achieve tritium self-sufficiency. The figure of merit used to assess tritium self-sufficiency is the tritium breeding ratio (TBR), i.e., the ratio between tritium produced and tritium burnt in the system. TBR
3He is instead produced by D-D reactions or by tritium decay. In principle, a D-3He fusion device does not require a breeding blanket because it relies on an open fuel cycle, removing the complexity of a breeding blanket from the reactor design but introducing the need of an external source of 3He. A large source of 3He is found in Moon regolith (Kulcinski and Schmitt, 1988). Many analyses have been carried out on the feasibility of 3He mining from the Moon (Sviatoslavsky, 1988; Crabb and Jacobs, 1992), but only conceptual designs and missions have been produced so far because of the high financial risk of the projects and lack of validated technologies.
Boron is much more common than T or 3He on the Earth. The main reserves are in Chile, China, Russia, and in the US. No supply issues are foreseen in the next future at the current rate of consumption (U.S. Geological Survey, 2023).
In the majority of confinement approaches being currently pursued (MCF and MTF), the success of the project relies on the possibility of generating a precisely controlled magnetic field distribution, and superconducting magnets and coils are needed to obtain it in a cost-effective manner. In fact, resistive Cu magnets can attain very high fields, but the amount of power required to run them, due to dissipation, makes them not viable for a power plant design requiring therefore the use of superconductors. In order to achieve compact MCF or MTF reactors the magnetic fields need to be particularly high, and the only currently available superconducting technology that allows conveniently achieving such
Materials operating within a fusion reactor will be exposed to a harsh radiation environment, depending on the region of the device where they will be employed, and mainly determined by the long-term irradiation by high-energy (up to 14 MeV) neutrons (Guo et al., 2015). A strong research effort is devoted to understand the radiation hardness of plasma facing components and structural materials (Ferraris et al., 2008; Rowcliffe et al., 2018; Torsello et al., 2022a), but this issue is also crucial for sensing and detection devices and functional materials such as superconductors for magnets. Although placed relatively far from the plasma, the radiation environment can represent an issue for materials whose useful properties are sensitive to much lower damage levels (of the order of 10–3 dpa, where dpa stand for displacement per atoms and is a measure of the amount of defects introduced by the radiation). This is the case of superconductors (Fischer et al., 2018), for which the operating conditions (cryogenic temperature and high circulating current) make it particularly difficult and interesting to investigate experimentally the performance under or after neutron irradiation (Iliffe et al., 2021; Torsello et al., 2022b). In this regard, aneutronic fusion reactions (i.e., those fusion reactions which do not produce neutrons) are of particular interest because they may relax some of the constraints in reactor design related to material damage. It should be noted that p-11B and 3He-3He are aneutronic reactions Eqs 5, 6, while the D-3He reaction (Eq. 4) is not completely aneutronic due to the D-D side reaction (Eq. 1).
The goal of a fusion device is to achieve net energy production, which is essential for its practical application as an energy source. One of the key components of a fusion device is therefore the power conversion system, which is necessary to convert the energy released by fusion reactions into a form that can be used to generate electricity. In D-T fusion, 80% of the energy released by fusion reactions is carried by neutrons in the form of kinetic energy. This energy must first be converted into thermal energy, which is achieved through the use of a blanket. Neutrons are slowed down as they pass through the blanket, which heats a coolant that flows through it. After this stage, power conversion takes place as in conventional power plants by means of a secondary coolant that actionates one or more turbines. Notable exceptions are direct electricity generation for p-11B reactions (Yoshikawa et al., 1991) and direct ejection of high-energy particles for space propulsion (Razin et al., 2014).
Estimating the cost of future fusion reactors is a challenging task for both analysts and companies. Nevertheless, there are no doubts that fusion reactors (and fusion power plants) will require huge investments. Preliminary estimations for the conceptual design of ARC resulted in a total gross cost of approximately $5.5B (Sorbom et al., 2015). Similarly, the US NASEM report sets as target $5–6B for a fusion pilot plant (NASEM, 2021). The cost estimate for ITER based on the 2001 design was €5B (ITER, 2023), but the cost increased up to €20B4, while some of the member countries are arguing that the final cost will be even higher (Kramer, 2018). Major drivers for fusion reactor cost are the large size of the plant and the amount of raw materials required (steel, concrete, etc.), need for specific components for which standardised production is not yet available (e.g., superconducting magnets, vacuum vessel, etc.), and one can reasonably expect a strong contribution from the capital cost (i.e., high interests on debt) due to the financial risk associated to commercial fusion projects [as expected for similar, innovative technologies like IV generation fission reactors (Mignacca and Locatelli, 2020)].
In this section we present the most relevant commercial projects in nuclear fusion from organizations working toward a demonstrator. The projects are classified according to the plasma confinement approach (MCF, ICF, MTF) and have been selected from the main database on nuclear fusion projects [e.g., (IAEA, 2021)]. A large fraction of the technical details reported within the projects have been published in peer reviewed journals. Whenever data could not be found in the scientific literature, we omitted it or we clearly stated that the source is the company itself. Since not all the projects have a unique name, the subsections are named after the company or the institution, and in each subsection they are listed in alphabetical order.
Magnetic fields are a natural candidate for plasma confinement, because the electrically charged ions and electrons that make up the plasma interact with the magnetic field lines and follow well defined orbits, avoiding contact with the reactor walls. For this reason, one of the key enabling technologies for MCF is that of superconducting coils. A superconductor can carry several orders of magnitude more current density than a normal metal, and do so without any dissipation. However, the cables must be kept at cryogenic temperatures and have limitations on the maximum current and magnetic field they can withstand (Figure 3).
FIGURE 3. Whole wire critical current densities as a function of applied magnetic field for the superconducting materials currently considered for the design of fusion reactors. It should be noted that two sets of data are presented for REBCO tapes (parallel and perpendicular applied field) since their properties are strongly anisotropic. Data from (Parrell et al., 2004; Boutboul et al., 2006; Braccini et al., 2010).
The properties of the first generation of commercial superconducting cables (low-temperature superconductors NbTi and Nb3Sn) allowed the development of large tokamak projects such as EAST (Wu and the EAST team, 2007), ITER (Romanelli et al., 2012), and KSTAR (Lee et al., 2001). Since the reactor cost increases with the reactor size, compact reactors have gained popularity in the last decade. However, there are three drawbacks when decreasing the reactor size. First, the confinement time is proportional to the reactor major radius (Costley, 2016). Larger reactors achieve better confinement (keeping all the other reactor parameters fixed). Second, the total fusion power is directly proportional to the plasma volume, hence to the reactor size. Third, the wall and exhaust loading increase at fixed total thermal power, requiring the first wall and divertor materials to withstand very high particle and heat fluxes (Knaster et al., 2016), and introducing challenges in the design of the cooling systems (Ferrero et al., 2022). While the last issue requires advances in material science and engineering, the first two issues can be overcome by better performing superconductors that can produce stronger magnetic fields. For this reason, the improvement of HTS tape technology recently opened the way to cheaper, smaller scale, projects often financed by private companies or single universities, dramatically enhancing the dynamics of the research field. Tokamak, spherical tokamak, stellarator, field-reversed configuration (FRC) and Z-pinch are among the most studied devices for MCF.
Tokamaks have been studied since the 50s (Barbarino, 2020) as MCF devices. The plasma is confined within the tokamak by a combination of magnetic fields generated by superconducting coils (whose dimensions can be significantly large depending on the radius of the machine) surrounding the torus, and an electric current flowing through the plasma itself. External current drive (e.g., radiofrequency systems or neutral beam injection) may be exploited to operate a steady-state tokamak-based power plant, but efficiency improvements in external current drive systems are required. Alternatively, the plasma current is produced by a central solenoid (with the plasma working as the secondary side of a transformer) which inevitably leads to pulsed operations. There are several tokamaks in operation around the world, aiming at demonstrating working principles of fusion technologies and at investigating plasma parameters. Some of the most notable ones are: JET (Joint European Torus) in the United Kingdom (Pamela et al., 2003); ASDEX Upgrade in Germany (Stroth et al., 2013); DIII-D in the US (Luxon, 2002); EAST (Experimental Advanced Superconducting Tokamak) in China (Wu and the EAST team, 2007); KSTAR (Korea Superconducting Tokamak Advanced Research) in the Republic of Korea (Lee et al., 2001); T-15 and T-10 in Russia (Smirnov, 2009); JT-60SA in Japan (Kamada et al., 2013). The most relevant projects for commercial fusion based on tokamaks are reported in the following.
The affordable, robust, compact (ARC) reactor, originally proposed by the Plasma Science and Fusion Center (PFSC) at MIT (Sorbom et al., 2015; Kuang et al., 2018) and under development by Commonwealth Fusion Systems 5 is a compact tokamak featuring HTS, a liquid immersion blanket (LIB) and a replaceable vacuum vessel. The main idea behind this project is to reduce the size of a fusion reactor thanks to a compact and modular structure, thus decreasing construction and maintenance costs. The ARC reactor will have a major radius of 3.3 m and generates a fusion power of 525 MWth in a plasma volume of ∼ 140 m3. Such a high power density can be achieved thanks to the implementation of REBCO (rare earth barium copper oxide) HTS. Power extraction and tritium breeding is fulfilled by a liquid breeding blanket consisting of a low-pressure, slow-flowing lithium beryllium fluoride molten salt (FLiBe) contained in a tank that surrounds the vacuum vessel. A TBR
A broad class of fusion power plants goes under the name of DEMO. DEMO designs follow the path depicted by ITER (International Thermonuclear Experimental Reactor) to achieve MCF with large-sized devices. Scaling ITER technologies to a fusion power plant requires significant efforts, and additional components (e.g., a breeding blanket). ITER will indeed test some of these components prior to their deployment on DEMO design [e.g., the test blanket modules (Giancarli et al., 2012)]. Different DEMO conceptual designs have been proposed by the partner countries in the ITER project, namely, EU DEMO (European Union) (Federici et al., 2018), JA DEMO (Japan) (Tobita et al., 2019), K-DEMO (Republic of Korea) (Kim et al., 2015), and CFETR (China) (Wan et al., 2017). Multiple options for the breeding blanket technology have been investigated and are being tested (water-cooled lead-lithium, dual coolant lead-lithium, helium-cooled pebble bed, etc.), whereas low temperature superconducting magnets (Nb3Sn) are the baseline technology for all the designs, but HTS tapes are also being considered for some magnet subsystems. The thermal power production of such large-scale devices is order of few gigawatts (1,500–2000 MWth for the JA DEMO, 2000 MWth for EU DEMO, 2000–3,000 MWth for K-DEMO,
Stellarators are the second most studied type of MCF reactors. Similar to tokamaks they use magnetic coils to confine the plasma in a toroidal region, but no electric currents are driven in the plasma, thus removing the need for a central solenoid. The result is that a single coil system does the work of poloidal and toroidal field coils, and the device is inherently steady-state. This simplification comes at the price of a nonplanar, twisty shape of the coils composing a stellarator: the geometry is complex and the construction extremely challenging. However, this design provides an exceptional intrinsic plasma stability and the possibility of continuous operation. This approach has been investigated in public projects aimed at demonstrating the working principles, the main ones being Wendelstein 7-X in Germany, the Helically Symmetric Experiment (HSX) in the US, and the Large Helical Device in Japan (Boozer, 2021). Recently, private companies also started developing the stellarator concept in France (Renaissance Fusion) and United States (Type One Energy and Princeton Stellarators) with ambitious goals that require huge innovations in some fields, especially related to the complex production of nonplanar coils on a large scale. It should be mentioned that, despite their complexity, stellarator coils are usually smaller than tokamak coils. In-factory production and shipping to the reactor site seems a viable approach for stellarators, rather than on-site manufacturing with the additional costs.
Princeton Stellarators 6 intends to utilize arrays of planar HTS magnets instead of the complexly shaped three dimensional coils required in all other proposed stellarator architectures, strongly simplifying the manufacturing. No public timeline is available.
Renaissance Fusion 7 intends to develop a segmented stellarator design in which the building block are cylindrical tubes on which the HTS is directly deposited and patterned and that is internally covered by a liquid metal (Li and LiH) layer that provides neutron shielding, heat removal and tritium breeding (Clery, 2022). They aim at achieving a first demonstrator of the building block by 2024, allowing the realization of a high field (10 T) stellarator able to produce net fusion energy by 2025. The final goal is to realize a machine able to produce electricity (Qe > 1) in the early 2030s.
Type One Energy 8 focuses on achieving a strong optimization of the plasma stability by employing high performance computing methods and on the development of a comprehensive platform (called NEBULA) for advanced additive manufacturing of HTS magnet assemblies (Clery, 2022). They propose this manufacturing route as a way to dramatically speed up the building times of fusion reactors, aiming at a target of 2 years for complete plant installation.
A spherical tokamak is a modification of the tokamak design in which an extremely small aspect ratio is used, allowing to reduce the size of the hole of the torus as much as possible. The result is a plasma shape that is almost spherical, reminding a cored apple (Stambaugh et al., 1998). The magnetic field is generated by a set of external coils that surround the plasma, and the resulting shape improves the plasma confinement efficiency. The shape of spherical tokamaks leaves little room for a breeding blanket on the inboard side, and shielding the inner legs of the toroidal field coils becomes harder than in a classical tokamak. HTS magnet technology also in this case provides the most promising route to compact, cost-effective fusion energy with this design. Experimental spherical tokamaks operating all over the World includes The National Spherical Torus eXperiment-Upgrade (NSTX-U) in the US (Menard et al., 2017), QUEST in Japan (Takase et al., 2022) and MAST-U and START in the United Kingdom (Sykes et al., 1999). Relevant projects are carried on by Tokamak Energy and UKAEA (United Kingdom Atomic Energy Agency).
Tokamak Energy 9 has been working on this design for over a decade, realizing tabletop fusion test devices (Sykes, 2015). The ST25 was the first (spherical) tokamak using entirely HTS magnets, while the ST40 achieves a toroidal field of 3 T using cooled copper and aims to demonstrate burning plasma condition parameters (Gryaznevich and Asunta, 2017; Dnestrovskij et al., 2019; Hong, 2022) (the company announced achieving a plasma temperature of 100 million K and a triple product of 6 ⋅ 1018 keV s m−3 in 2022), while HTS magnets with the required performance are being developed in parallel (Prager, 2019). These two advancement paths should converge in the ST80-HTS device that should be complete in 2026, as a last step before the first pilot plant (ST-E1) that is thought to deliver electricity in the early 2030s.
STEP (Spherical Tokamak for Energy Production) is a spherical tokamak project proposed by UKAEA10 within the United Kingdom strategy for fusion commercialization (Wilson et al., 2020). The project leverages the previous experience of the United Kingdom on tokamaks (e.g., JET) and spherical tokamaks [e.g., START and MAST (Sykes et al., 1999)]. A possible strategy for STEP foresees a first prototype, SPR, built with known technologies (e.g., using low temperature superconducting magnets instead of HTS), with the possibility to upgrade the machine with HTS once such technology becomes more mature. A pre-conceptual design of the breeding blanket has been proposed by Fradera et al. (2021) exploiting molten FLiBe (LiF-BeF2) encapsulated in pebbles packed together to form multiple breeder units. Other blanket concepts might be under investigation, and a UKAEA team recently published a tool for preconceptual optioneering of STEP breeding blanket (Merriman et al., 2023). STEP roadmap can be divided in three stages (Wilson et al., 2020): develop an integrated conceptual design for SPR (2019–2024); develop the engineering design of SPR (2024–2032); construction and commissioning of SPR to deliver electricity (2032–2040).
Other MCF configurations have been investigated in the past, and some companies based the design of their devices on configurations different from tokamaks, stellarators or spherical tokamaks. Among them, Z-pinch, fusors, field-reversed configurations, and spheromaks are exploited for novel desings.
Field-reversed configurations are compact toroidal-symmetric configurations characterized by the presence of solely a poloidal magnetic field, without toroidal field magnets and central solenoid. The configuration is called field-reversed because a toroidal electric current is induced inside the plasma, which generates a poloidal magnetic field reversed from the externally applied magnetic field. Although theoretical models predict strong instabilities in FRC, small-scale experiments demonstrated a plasma stability far better than expected. This, and the simpler systems required to generate a FRC if compared to tokamaks or stellarators, have made the FRC a configuration worth of interest (Freidberg, 2007). The main applications of this technology are fusion power generation and space propulsion. It should be noted that FRC can be used for both MCF and MTF.
Spheromaks are similar to FRC, yet a toroidal field is present. However, the toroidal field does not require dedicated coils, making the spheromak a simple system. As for the FRC, system simplicity is outbalanced by stability issues.
CTFusion (United States) designed Dynomak, a high-beta spheromak reactor, following the HIT-SI (steady, inductive, helicity injected torus) experiment at the University of Washington (Sutherland et al., 2014). This reactor is designed to use imposed-dynamo current drive, which enables a stable spheromak configuration. Other important technologies included in the design are HTS tapes and a liquid breeding blanket. The choice to use HTS for the superconducting equilibrium coils is dictated by the need to reduce cooling power and to reduce the size of the system. Zirconium hydride (ZrH2) will be used for neutron shielding, aiming at magnet lifetime of more than 30 years. The liquid breeding blanket is made of FLiBe. The reactor has a dual chamber system where FLiBe flows and a tritium breeding ratio greater than 1.1 should be achieved. Supercritical carbon dioxide will be used as a secondary coolant to feed a Brayton cycle for energy production. Dynomak has a major radius of 3.75 m and is designed to generate a fusion power of 1953 MWth, resulting in an expected electrical power production of 1,000 MWe (Sutherland et al., 2014).
The Horne technologies company is following the road of high beta, fusor-like machines [a design based on the inertial electrostatic confinement, exploiting electric fields to confine the plasma instead of magnetic fields (Miley and Murali, 2014)] combined with HTS (REBCO), for terrestrial and space purposes. The company was founded in 2008 and details on their design are not available in literature, but it claims to have achieved the first world’s superconducting, high beta plasma research device in operation and the first use of REBCO wires for plasma confinement. A second generation device has been targeted online by the company for 2022, with the aim of improving coil structures, cryogenic cooling and diagnostics, reaching a 50 keV plasma temperature and performing experiments of optimization; anyway at the present time (2023) there is no updated public information about that prototype.
The Compact Fusion Reactor (CFR) design proposed by the development program of Lockheed Martin 11 (California, United States) aims to overcome the main problems of fusion devices by operating at a high beta (ratio between plasma pressure and magnetic pressure). The confinement would be guaranteed by a combination of magnetic mirrors and cusp confinement (Freidberg, 2007) that leverages on plasma diamagnetism, generating the magnetic fields with superconductive coils. The design foresees a zero net plasma current, identified by the company as a main reason for plasma instability. Prototypes have been realized to test the plasma behaviour in such a configuration, as the T4B experiment. No timeline is available for CFR.
The Direct Fusion Drive (DFD) proposed by Princeton Fusion Systems 12 (United States) is a compact fusion engine for space propulsion. The goal of DFD is to enable exploration missions in the Solar System (Razin et al., 2014). The engine is designed to use D-3He fuel, exploiting a FRC to confine the plasma within a linear solenoid coil. The DFD concept is based on a direct propulsion system, in which a large fraction of the energy from the fusion reactions is directly transferred to the edge plasma ions that are then used as propellant, rather than being indirectly transferred to a propellant through the heating of the surrounding structure (Razin et al., 2014). This allows for a more efficient conversion of fusion energy into propulsion. Nevertheless, the reactor has to be specifically designed to produce the high-energy ion beam and to direct it outside the reactor, a feature that has not been addressed by fusion reactors for terrestrial applications. Waste heat from bremsstrahlung and plasma synchrotron radiation is designed to power a thermodynamic cycle that powers the auxiliary systems and the spacecraft itself. In this regard the DFD would be highly effective because it provides both propulsion and electricity production.
TAE technologies 13 is one of the largest privately-funded fusion research companies, founded in 1998 and located in southern California (United States); at the present time (2023) they have online their fifth generation experimental device, C-2W (also known as “Norman”), the largest compact-toroid device in the world. The approach followed by the company is a magnetic confinement based on a FRC, coupled with a neutral beam injection system for the plasma heating and current drive. The declared final goal for what concerns the fuel is eventually to use advanced solutions like p-11B or D-3He to avoid the generation of fast neutrons and consequently reduce issues related to radiation damage and material activation. It must be noticed, however, that the fusion cross sections for such reactions are dramatically lower than the D-T one at the currently affordable plasma temperature, so that the generation and maintenance of such a fusion environment appears to be non trivial nowadays (Ongena, J., 2015). The usage of a machine-learning framework for experimental optimization and other technical improvements allowed C-2W to reach better results than his predecessor C-2U, generating plasmas sustained in steady-state for up to 30 ms, with an electron temperature Te > 500 eV (Gota et al., 2021).
The reactor proposed by Zap Energy 14 (United States) aims to avoid expensive and complex issues related to magnetic confinement in classical tokamaks reconsidering the Z-pinch layout [a confinement layout in which the compression of the plasma is obtained by the magnetic field generated by the plasma current itself, directed in the z-direction (Freidberg, 2007)] stabilizing the plasma column with sheared flow for a time long enough to allow fusion reactions to occur in a D-T plasma. The concept has been tested in the FuZe experiment, consisting in a 50 cm long assembly region for the pinch formation, preceded by a 100 cm coaxial plasma accelerator. The pinch formation has been observed in the deflagration discharge mode (Stepanov et al., 2020) and stability for 20 microsecond has been reached with a pinch current of 100–200 kA approaching a Te of 1 keV (McLean et al., 2017). The final reactor should be designed for a pulsed operation, generating 10 plasma pulses per second, including a breeding system for the in-plant tritium production, generating 50 MWe of electrical power in a device with a width of 3 m. Superconductive magnets are not foreseen in this design to reduce the cost, and a single zap energy power station should house many cores, exploiting modularity.
Inertial confinement fusion exploits extremely high compressions of solid targets to bring the fuel up to ignition conditions (n ∼ 1025m−3). Although some concepts exploit non-thermal acceleration of fuel ions up to suitable energies for fusion reactions, the most common approach is based on spherical pellets heated uniformly by laser beams. The energy burst ablates the outermost layer of the sphere, generating an inward shock wave that compresses the fuel, with a consequent fuel temperature increase up to 1–10 keV. The process takes place in extremely short times
First Light Fusion 15 (United Kingdom) approach to ICF exploits a high velocity projectile (instead of laser beams) to compress the fuel target to fusion conditions. The projectiles are accelerated in a gas-gun, while the target is embedded in a plastic block. Preliminary tests to validate the working principle have been performed on D-D targets during an experimental campaign that comprised 21 shots (UKAEA, 2022). Measurements from the experimental campaign provide evidence of fusion reactions taking place during the experiment. Furthermore, two machines (M3 and BGF) aimed at validating the accelerating system technology have been commissioned and built. The power plant will exploit D-T fuel and foresees a liquid metal (natural Li) first wall, and a classical thermodynamic cycle to produce electricity. Operations will take place in pulsed mode, with an expected power output of 150 MWe. No public timeline has been released by the company.
Marvel Fusion 16 (Germany) aims at achieving p-11B fusion reactions exploiting high energy lasers. The laser system is based on the established technology used in operating experimental facilities, such as the National Ignition Facility. However, p-11B fusion reactions require much more demanding conditions to take place. At the present time no roadmap or power plant design are available.
Magnetized target fusion combines the MCF and ICF approaches to achieve fusion reactions in a medium-density plasma [e.g., 2 ⋅ 1023cm−3 (Laberge, 2019)] at temperatures comparable to those in MCF
Any decrease in plasma volume driven by a linear compression ratio C increases the density by C3 and temperature by C2. Therefore, even a small compression ratio can bring the plasma to ignition conditions. The presence of an external magnetic field (which is absent in ICF) confines the plasma for times long enough to produce net fusion energy. From a technological point of view, MTF does not require high energy lasers or complex magnet systems, thus resulting in simpler systems than ICF or MCF. Nevertheless, MTF is not exempt from criticality due to the complexity in integrating two different confinement approaches. Some companies are developing quite different designs based on MTF, namely, General Fusion, Helion Energy and HB11 Energy.
General Fusion 17 is a company based in Canada pursuing MTF by a mechanically driven liquid metal liner. The working principle is the following: plasma is produced in a magnetized Marshall gun and injected in a cavity whose walls are made of liquid metal (Mossman et al., 2022). The liquid metal walls are kept in position by setting the liquid metal in rotation inside the reactor chamber. Mechanical pistons surround the chamber and compress the cavity in short times (∼ 100 ms). Once the compression starts, the plasma is injected into the cavity. A linear compression ratio of
HB11 Energy 18 pursues non-thermal p-11B fusion by a combination of ultra-high acceleration of plasma blocks in a solid target and ultra-high magnetic fields (∼10 kT) by high-power lasers. The solid target is a small cylinder of hydrogen and boron placed in a capacitive coil. Two lasers are required to reach ignition conditions. A first laser hits the target, while a second laser produces the ultra-high magnetic field by interaction with the capacitor coil (Fujioka et al., 2013). Several experiments have been carried out to investigate the basic principles of non-thermal fusion (Belyaev et al., 2005), the interaction between PW-scale lasers with fuel blocks (Margarone et al., 2022) and the optimal scheme (bulk vs. pitcher-catcher) (Willingale et al., 2011), and the existence of an avalanche reaction in the fuel target (Hora et al., 2015). Direct electricity generation is foreseen by decelerating the alpha particles exploiting a high voltage spherical shell in which the target and the coil are located. No fusion power plant is planned for the near future, but a roadmap highlighting the most important steps (e.g., numerical analysis of capacitor coil magnetic field, behavior of materials under kT magnetic fields, improvement of PW-class lasers) to achieve power production from p-11B fusion reactions has been developed (Hora et al., 2017).
Helion Energy 19 is based in the U.S. and is designing FRC devices (Slough et al., 2011) as modules of an MTF reactor to produce fusion energy from D-D and D-3He reactions. The company built and tested 6 prototypes in the previous 10 years based on the IPA (Inductive Plasmoid Accelerator) design (Slough, 2009). The baseline design exploits a linear device to accelerate two FRC plasmoids against each other. The collision compresses the plasma up to fusion conditions for a short time. After the compression phase, the gasses are exhausted by means of two divertors located at the extremities of the linear device (Slough et al., 2011). A key feature of the fusion machines proposed by Helion energy is the use of D-D fuel instead of D-T fuel. The D-D reaction has indeed a lower yield of fast neutrons. Half of the D-D reactions produce a fast neutron at 2.45 MeV (and a 3He nucleus) instead of the 14 MeV neutron produced by D-T reactions. Nevertheless, the remaining D-D reactions produce tritium, which can then react with a deuteron in a D-T reaction. The 3He produced during operations is expected to be used as fuel for D-3He reactions, which produce no neutrons. In such a way, fast neutron production is minimized, but not completely avoided. A fusion power plant based on this concept could be able to produce 25 MWe (50 MWe at 2 Hz) according to the company. No timeline has been published by the company.
Despite the fact that this review focuses on the private sector, and that certainly private initiative and funds played a pivotal role in this revival and increase of dynamism in the nuclear fusion field, we want to stress that publicly funded research and projects are the basis on which these new opportunities stand, and that the vast majority of the people involved in these companies come from the academic world, where they built the skills that are currently employing for this commercial-oriented research. The NIF and ITER are two notable examples that contribute to advancements in nuclear fusion from distinct perspectives. The NIF has recently achieved ignition for the first time, providing a valuable scientific contribution to ICF research and demonstrating that ignition can be achieved by this confinement approach (Bishop, 2022; Zylstra et al., 2022). ITER has shed light on the many challenges that arise when large-scale science projects, based on conceptual design, are translated into real engineering systems. The lessons learnt on management, collaboration between universities and private partners, technology and knowledge transfer (Puliga et al., 2020) have been successfully implemented by some of the commercial fusion companies (e.g., those companies that were born as university spin-offs and maintained a tight collaboration with universities). In this regard, capitalizing scientific, technical, and management knowledge might be crucial for these private companies to attract and justify investments, and to provide an additional revenue stream (e.g., patents, intellectual property rights, etc.). Most of these companies show the traits of knowledge management leaders, such as the presence of knowledge champions (e.g., collaborators from universities) coupled with top management, a holistic perspective (strategic, technological, organizational), an effective communication with the public, and an attitude towards continuous learning and innovation (Skyrme and Amidon, 1997). All these features are fundamental for companies working in a field where most of the assets are still intangible.
Private companies can also benefit from public-funded research at a more fundamental level. The experience from past experiments ranges through many applications [e.g., from the Molten Salt Reactor Experiment (Haubenreich and Engel, 1970) at ORNL to the liquid lead-bismuth eutectic at ENEA (Foletti et al., 2006)], providing a solid background for different designs. Current and future experiments (e.g., those within the EUROfusion program) on materials, tritium handling, tritium extraction, etc. will tackle critical topics for the successful development of fusion energy, allowing private companies to focus on design-related issues instead of broader, fundamental topics.
In the remainder of this section we discuss topics that we deem of high interest at this stage of development of commercial fusion projects, namely, financing fusion energy (and possible synergies between public and private sectors), the geographical distribution of the companies, the technological maturity and the criticality of the designs, the timelines proposed for commercialization of fusion reactors, and the impact of fusion power plants on the energy sector.
The huge investments (∼ $4.8B (FIA, 2022)—Table 1) made in the private fusion sector in the last years are just a first step towards the successful development of commercial fusion projects, considering that ∼ $4.7B (i.e., 98% of the total investment) come from the private sector (FIA, 2022). Even larger investments will be needed in the forthcoming years. Figure 4 shows a conceptual, business-oriented, collaborative model between private companies, governments and public institutions to advance fusion research, and build and operate fusion reactor demonstrators and, ultimately, FPPs (Fusion Power Plants). We exploited the 4-A framework for energy security (Availability, Accessibility, Acceptability, Affordability) proposed by the Asia Pacific Energy Research Centre to enhance energy security and sustainable development (APERC, 2007). Each block in Figure 4 represents an action that can be undertaken by the players (private companies, governments and public institutions, or both). Resource availability accounts for both natural resources and artificial resources (i.e., tritium). Governments must ensure that natural resources and tritium are available and accessible to develop critical technologies, to manufacture components and to operate the fusion reactors. Tritium reserves must be carefully assessed and monitored to guarantee that multiple prototypes/demonstrators can operate with D-T fuels. Most of the public and private investments go to R&D at this stage. This requires private companies to maintain the appeal for the investors. Although the ultimate goal is the production of electricity to the grid, private companies may apply their scientific and technological expertise (e.g., plasma physics, material science, nuclear engineering - more in general, their IP) to side markets (e.g., medical technology, robotics, superconducting technology) to get short-term returns (Alhamdan et al., 2022). Spin-offs can be created to better exploit commercially the IP rights resulting from R&D. Private companies may benefit from spin-offs by adding an additional revenue stream, while public institutions (e.g., research laboratories or universities) may benefit from a tighter coupling between research and industry, and additional funds. Additional support from governments may come from energy policies. Governments involvement have been fundamental in time-intensive and capital-intensive sectors because it can provide an insurance, and mitigate the risk, for private investments in that sector (Alhamdan et al., 2022). As the experience with nuclear fission plants demonstrates, engagement with the public and with the political spheres is mandatory to guarantee public acceptance of a technology. Communicating effectively safety-related topics to the population, and providing a basic background for decision-makers are two key actions that can be undertaken by the governments and public institutions, supported by experts from the private sector. Lastly, government agencies must establish a regulatory framework to build and operate fusion reactors.
TABLE 1. Claimed funding for fusion companies in 2022 (FIA, 2022).
FIGURE 4. Conceptual model for synergic collaborations between private companies, governments and public institutions. The flow diagram is based on the 4-A framework for energy security. Each block represents an action that can be undertaken by private companies (light red), governments and/or public institutions (light blue), or both (light blue/light red). The ultimate goal is to build and operate a fusion reactor demonstrator and then an FPP, but funding and R&D play a crucial role to achieve this goal.
It is clear that governments’ involvement is fundamental to keep funding public institutions (part of which collaborates with private companies), to establish a regulatory framework for fusion power plants, and to coordinate private initiatives at national and international level. The US showed its intention to develop a plan to build a pilot plant starting from the 2019 NASEM (National Academies of Sciences, Engineering and Medicine) report (NASEM, 2019), which has been followed by the 2021 consensus status report (NASEM, 2021), and by the 2022 White House summit on developing a bold vision for commercial fusion energy. The plan foresees a strong collaboration between US DOE (Department Of Energy), public institutions, and private companies, including government investments to support public-private partnerships. A similar approach based on public-private partnerships is followed by the United Kingdom, with the UKAEA collaborating with private companies (UKAEA, 2023a; UKAEA, 2023b; UKAEA, 2023c). The EU provides funding to fusion research and institutions through Horizon Europe and the Euratom Research and Training Programme. While private companies may be partner of public institutions and research centers which benefit from the funding scheme, no direct investment plans to private fusion companies are used in the EU.
The geography of privately funded projects is depicted in Figure 5. All the private companies are based in less than 10 countries. Figure 5 should not be interpreted as a measure of the technological (or scientific) maturity of a given country in the field of nuclear fusion. Yet, it highlights how commercial fusion is also strongly influenced by the socioeconomic context and by the inclination towards entrepreneurship of researchers and investors. This fact clearly emerges by the comparison with Figure 6. Fusion (experimental) devices, which usually belongs to public research institutions, are well widespread among rich countries. The absence of private projects in most of these countries can be related to the strong involvement of the public sector in commercial nuclear fusion projects (e.g., China), to the focus towards a single, huge international project (e.g., EU countries), or lack of political and financial interest towards commercial fusion. Furthermore, Figures 5, 6 highlight the absence of nuclear fusion projects and devices beyond rich countries. This raises questions on the accessibility of nuclear fusion technologies in developing countries. The development of commercial fusion projects is often characterized by significant investments, access to advanced technologies and experimental facilities, and the presence of a strong industrial sector to meet the demand for components and services. Many developing countries may not possess one or more of these requirements, creating a barrier for these countries to enter the commercial fusion field. However, coordination and mediation from a third party, coupled with financial resources, can effectively spread fusion technologies to countries beyond those depicted in Figure 5. This pathway has already been explored and implemented for nuclear fission power plants and has been demonstrated to be an effective means of providing access to this technology in developing countries (Omoto, 2005; Fischer et al., 2018; Alam et al., 2019).
FIGURE 5. Geography of private company involved in commercial fusion projects. Most of the companies are located in the US, and, more in general, in Western countries. DEMO designs have not been considered in this map.
FIGURE 6. Geography of public institutions involved in nuclear fusion research. Public institutions are more widespread if compare to private companies in Figure 5. Data from (IAEA, 2021).
As a consequence of the recent revived interest and consequent blooming of start-ups and companies active in the nuclear fusion field, some of the projects listed above are extremely young (less than 5 years), with a thin structure and limited working force, whereas some have a long track record of studies and prototypes. This alone does not give an indication of the disruptive potential of a company, but the promises of extremely fast development should be weighted against this, despite all the enthusiasm. For instance, even well-established technologies may experience long delays (i.e., years) related to “conventional” issues such as construction or design changes [e.g., nuclear power plants (Alsharif and Karatas, 2016; Eash-Gates et al., 2020)]. The roadmaps proposed by private companies are in general way shorter than the ones indicated by the public research institutes [e.g., DEMO roadmap (Romanelli et al., 2012)]; on the one hand, this can be due to the need for collecting private funds, and on the nature itself of private projects. While the interest of the public research is in general on the knowledge itself, private research is focused on obtaining a commercially deliverable device as soon as possible. In a public research facility many different experiments from many different research groups must be scheduled during the operation time; while all of them are surely important for the enhancement of our comprehension on different aspects of nature, not all of them actually lead to a technical enhancement in the short period. Despite the high financial risk associated to commercial fusion at the current stage, attracting funds is inevitably much easier for private companies that target commercialization than public institutions. Moreover, large public research programmes like ITER/DEMO involve major international collaborations; different components or subsystems of the same machine must be designed and realized in different countries, involving an effort in standardization and possibly delays in the overall projects. Private companies can instead leverage on smaller teams and more effective project management and financial structures to target shorter roadmaps and sooner milestones. While private companies have benefited from the past scientific achievements, public institutions may learn alternative project management approaches from these companies. We believe it is worth noting that doubts have been expressed on these private projects from the scientific standpoint (Jassby, 2022). The absence of experimental results from private projects has been interpreted by some authors as a red flag, especially if compared to the huge investments raised for these projects. While the lack of reported experimental results is indeed true for most of the companies, which base their design on scientific concepts that, although very promising, have not yet been demonstrated for large scale devices, or strongly rely on numerical simulations, the validity of the project should not be judged by these factors alone. Nuclear fusion is a capital intensive field, and an ambitious goal such as developing and building a fusion power plant requires large investments from the very early stages of technology development (i.e., from a TRL = 1–2). The technological maturity issue is addressed in the next subsection (4.4).
Within Table 2 we attempt to give an indication of the maturity of each project. It is not possible to assign a precise overall TRL value because each company focuses on several aspects each at a very different stage of advancement, and it should also be bore in mind that we rely on publicly available data, whereas on several aspects the companies have the obvious interest and necessity to not disclose details of their research. In addition to the maturity level, we also attempt to identify the technical bottlenecks of these projects that at the moment pose a limit to their development. These critical steps, if overcome, will represent a major contribution to the overall technological advancement, often with positive repercussions in other fields.
TABLE 2. Technological maturity and main critical steps associated to each commercial fusion project.
Most of the companies are designing, building and testing proof of concepts. This is particularly true for companies relying on established physical processes and devices, such as tokamaks and stellarators. As for the pathway depicted by ITER and DEMOs, scaling up the prototypes to power plant devices will require significant effort and dedicated R&D on critical components. Possible criticalities have a different spectrum depending on the stage of the fusion project. Physics limitations might be encountered by those projects that are still validating the scientific foundations or the key technologies (e.g., HB11 Energy, Reinessance Fusion, Lockheed Martin, etc.). As the technological maturity increases, the criticalities become more specific and engineering-related. Plasma confinement for long pulses (MCF), vacuum vessel and blanket material capabilities to survive cyclical, thermomechanical stresses in a corrosive and radioactive environment (MCF), superconducting magnets lifetime (MCF and MTF), lasers power and efficiency (ICF, MTF), and shot frequency (ICF and MTF) are all engineering issues that have to be addresses and overcome to achieve a commercial fusion reactor. It is clearly premature to question the economical viability (i.e., the electricity cost) of fusion power plants, regardless of the confinement approach.
As a final word of caution, we would like to remind that technological and scientific progress do not follow a straight path, but rather rely on failures and mistakes to learn and improve. At this stage of great enthusiasm for fusion development it is important to be aware that between current efforts and successes and the achievement of fusion devices on the grid it is reasonable to expect a few snags and delays. A large fraction of the proposed design for on-grid fusion reactors will probably never reach the stadium of prototypes, leading to the convergence of the efforts on few solutions, according to the natural selection of technologies. The relative large number of different designs currently under investigation is probably indicative of the immaturity of the fusion in general, in particular considering that the debate is still on the more convenient physical process to exploit (e.g., MCF vs. ICF vs. MTF) and not just on engineering details. This situation can be clearly understood comparing fusion with a well-established technology like fission: while fission reactors can differ on many details, the fundamental physical processes exploited by more or less all the available facilities are the same; conversely in the first period of the human flight era, airplanes and aerostatates coexisted and it was not clear what the winning technology would have been. Also between the surviving leader solutions, the first test generation devices will probably undergo major failures in the first period of operations; while this is reasonable and historically documented for all the main technological innovations (e.g., airplanes development) it could constitute an issue in raising funds, being perceived as a tout-court failure of the research program, in particular after an aggressive advertising campaign with optimistic and bombastic claims. Lastly, the design and the business models of private companies will need to adapt to the future grid structure, which will inevitably different from the current grid structure. Some of the private projects will inevitably fail, still providing useful outcomes and insights for the advancement of nuclear fusion science and technologies. Private investments are enabling a simultaneous exploration of different options; this pathway would have never been possible to follow by public projects alone, and it should be considered an added value brought by private companies in nuclear fusion.
SM, FDL, DP, and DT, wrote the first draft of the manuscript. All authors contributed to the article and approved the submitted version.
FCL and DT acknowledge the support by the Italian Ministry of Education, University, and Research through Project PRIN HIBiSCUS, 201785KWLE. DT also acknowledges support by the “Programma Operativo Nazionale (PON) Ricerca e Innovazione 2014–2020”. COST Action CA19108 (Hi-SCALE), is also acknowledged.
FCL, DT, FDL, RT, and SM declare that they have an open commercial contract with Eni S.p.A. who is also a major shareholder of CFS. DT has a research grant co-funded by Eni S.p.A. FL (FDL) and DP have a PhD scholarship co-funded by Eni S.p.A.
The remaining authors declare that this the research was conducted in the absence of any commercial or financial relationships that could be construed as a potential conflict of interest.
All claims expressed in this article are solely those of the authors and do not necessarily represent those of their affiliated organizations, or those of the publisher, the editors and the reviewers. Any product that may be evaluated in this article, or claim that may be made by its manufacturer, is not guaranteed or endorsed by the publisher.
1Strictly speaking, the nucleons appearing through Eqs 1–5 are reactants. However, the fusion community often refers to those as fuel because they fuel fusion reactors. This nomenclature is used also in this work.
2The process involves a nuclear fission reaction but it is usually considered among fusion reactions.
3The reader should be aware that the Lawson Criterion here reported is derived for D-T plasmas, and it can be formulated in different ways depending on the applications (e.g., magnetic confinement fusion, inertial confinement fusion) and on the reactants.
4A non-negligible fraction of this cost increase is due to inflation. €5B in 2001 corresponds to €8.5B in 2023.
6https://www.princetonstellarators.energy/
8https://www.typeoneenergy.com/
9https://www.tokamakenergy.co.uk/
11https://www.lockheedmartin.com/en-us/products/compact-fusion.html
12https://www.princetonfusionsystems.com/
13https://tae.com/fusion-power/
15https://firstlightfusion.com/
19https://www.helionenergy.com/
Adams, R., Alexander, R., Chapman, J., Fincher, S., Hopkins, R., Philips, A., et al. (2003). Conceptual design of in-space vehicles for human exploration of the outer planets. Tech. Rep. NASA/TP-2003-212691. Available at: https://ntrs.nasa.gov/citations/20040010797 (Accessed 05 02, 2023).
Agostini, P., Angiolini, M., Alberghi, C., Candido, L., Capogni, M., Capone, M., et al. (2021). SORGENTINA-RF project: Fusion neutrons for 99 Mo medical radioisotope: SORGENTINA-RF. Eur. Phys. J. Plus136, 1140. doi:10.1140/epjp/s13360-021-02111-6
Aime, P., Gajeri, M., and Kezerashvili, R. Y. (2021). Exploration of trans-neptunian objects using the direct fusion drive. Acta Astronaut.178, 257–264. doi:10.1016/j.actaastro.2020.09.022
Alam, F., Sarkar, R., and Chowdhury, H. (2019). Nuclear power plants in emerging economies and human resource development: A review. Energy Procedia160, 3–10. doi:10.1016/j.egypro.2019.02.111
Alhamdan, A., Halem, Z., Hernandez, I., Lo, A. W., Singh, M., and Whyte, D. (2022). Financing fusion energy. Available at SSRN 4301605. doi:10.2139/ssrn.4301605
Alsharif, S., and Karatas, A. (2016). A framework for identifying causal factors of delay in nuclear power plant projects. Procedia Eng.145, 1486–1492. doi:10.1016/j.proeng.2016.04.187
Andreani, R., and Gasparotto, M. (2002). Overview of fusion nuclear technology in Europe. Fusion Eng. Des.61-62, 27–36. doi:10.1016/S0920-3796(02)00110-2
APERC (2007). Quest for energy security in the 21st century: Resources and constraints. Tokyo, Japan: Asia Pacific Energy Research Centre. Available at: https://aperc.or.jp/file/2010/9/26/EWG2006_5_Energy+Security+in+the+21st+Century.pdf (Accessed 05 02, 2023).
Atkinson, R. d. E., and Houtermans, F. G. (1929). Zur frage der aufbaumöglichkeit der elemente in sternen. Z. für Phys.54, 656–665. doi:10.1007/BF01341595
Bae, J. W., Peterson, E., and Shimwell, J. (2022). ARC reactor neutronics multi-code validation. Nucl. Fusion62, 066016. doi:10.1088/1741-4326/ac5450
Banacloche, S., Gamarra, A. R., Lechon, Y., and Bustreo, C. (2020). Socioeconomic and environmental impacts of bringing the sun to Earth: A sustainability analysis of a fusion power plant deployment. Energy209, 118460. doi:10.1016/j.energy.2020.118460
Barbarino, M. (2020). A brief history of nuclear fusion. Nat. Phys.16, 890–893. doi:10.1038/s41567-020-0940-7
Belyaev, V., Matafonov, A., Vinogradov, V., Krainov, V., Lisitsa, V., Roussetski, A., et al. (2005). Observation of neutronless fusion reactions in picosecond laser plasmas. Phys. Rev. E72, 026406. doi:10.1103/PhysRevE.72.026406
Bishop, B. (2022). National ignition facility achieves fusion ignition. Available at: https://www.llnl.gov/news/national-ignition-facility-achieves-fusion-ignition (Accessed 05 02, 2023).
Boozer, A. H. (2021). Stellarators as a fast path to fusion. Nucl. Fusion61, 096024. doi:10.1088/1741-4326/ac170f
Boutboul, T., Le Naour, S., Leroy, D., Oberli, L., and Previtali, V. (2006). Critical current density in superconducting Nb-Ti strands in the 100 mt to 11 t applied field range. IEEE Trans. Appl. Supercond.16, 1184–1187. doi:10.1109/TASC.2006.870777
Braccini, V., Xu, A., Jaroszynski, J., Xin, Y., Larbalestier, D. C., Chen, Y., et al. (2010). Properties of recent IBAD–MOCVD coated conductors relevant to their high field, low temperature magnet use. Supercond. Sci. Technol.24, 035001. doi:10.1088/0953-2048/24/3/035001
Brown, D. A., Chadwick, M., Capote, R., Kahler, A., Trkov, A., Herman, M., et al. (2018). ENDF/B-VIII. 0: The 8th major release of the nuclear reaction data library with CIELO-project cross sections, new standards and thermal scattering data. Nucl. Data Sheets148, 1–142. doi:10.1016/j.nds.2018.02.001
Bruzzone, P., Fietz, W. H., Minervini, J. V., Novikov, M., Yanagi, N., Zhai, Y., et al. (2018). High temperature superconductors for fusion magnets. Nucl. Fusion58, 103001. doi:10.1088/1741-4326/aad835
Bykovskiy, N., Uglietti, D., Bruzzone, P., and Sedlak, K. (2022). Co-wound superconducting wire for quench detection in fusion magnets. IEEE Trans. Appl. Supercond.32, 1–5.
Cipiti, B. B., and Kulcinski, G. L. (2005). The production of 13n using beam-target D-3He fusion reactions. Fusion Sci. Technol.47, 1245–1249. doi:10.13182/FST05-A858
Clery, D. (2022). Twisty device explores alternative path to fusion. Sci. (New York, NY)377, 1132–1133. doi:10.1126/science.ade7849
Costley, A. (2016). On the fusion triple product and fusion power gain of tokamak pilot plants and reactors. Nucl. Fusion56, 066003. doi:10.1088/0029-5515/56/6/066003
Crabb, T. M., and Jacobs, M. K. (1992). “Synergism of He-3 acquisition with lunar base evolution,” in The Second Conference on Lunar Bases and Space Activities of the 21st Century (NASA. Johnson Space Center), 475.
Creely, A., Greenwald, M., Ballinger, S., Brunner, D., Canik, J., Doody, J., et al. (2020). Overview of the SPARC tokamak. J. Plasma Phys.86. doi:10.1017/S0022377820001257
Dabiri, A. (1988). An overview of D-3He fusion reactors. Nucl. Instrum. Methods Phys. Res. Sect. A Accel. Spectrom. Detect. Assoc. Equip.271, 71–78. doi:10.1016/0168-9002(88)91127-8
Dnestrovskij, A. Y., Connor, J. W., and Gryaznevich, M. P. (2019). On the confinement modeling of a high field spherical tokamak ST40. Plasma Phys. Control. Fusion61, 055009. doi:10.1088/1361-6587/ab0bf8
Drake, B. G., Hoffman, S. J., and Beaty, D. W. (2010). “Human exploration of Mars, design reference architecture 5.0,” in 2010 IEEE Aerospace Conference (IEEE), 1–24.
Dyson, G. (2002). Project orion: The true story of the atomic spaceship. Henry Holt and Co.ISBN 0-8050-5985-7.
Eash-Gates, P., Klemun, M. M., Kavlak, G., McNerney, J., Buongiorno, J., and Trancik, J. E. (2020). Sources of cost overrun in nuclear power plant construction call for a new approach to engineering design. Joule4, 2348–2373. doi:10.1016/j.joule.2020.10.001
Federici, G., Bachmann, C., Barucca, L., Biel, W., Boccaccini, L., Brown, R., et al. (2018). DEMO design activity in Europe: Progress and updates. Fusion Eng. Des.136, 729–741. doi:10.1016/j.fusengdes.2018.04.001
Ferraris, M., Salvo, M., Casalegno, V., Ciampichetti, A., Smeacetto, F., and Zucchetti, M. (2008). Joining of machined SiC/SiC composites for thermonuclear fusion reactors. J. Nucl. Mater.375, 410–415. doi:10.1016/j.jnucmat.2008.02.020
Ferrero, G., Meschini, S., and Testoni, R. (2022). A preliminary CFD and tritium transport analysis for ARC blanket. Fusion Sci. Technol.78, 617–630. doi:10.1080/15361055.2022.2096365
FIA (2022). The global fusion industry in 2022. Available at: https://www.fusionindustryassociation.org/about-fusion-industry (Accessed 05 02, 2023).
Fischer, D. X., Prokopec, R., Emhofer, J., and Eisterer, M. (2018). The effect of fast neutron irradiation on the superconducting properties of rebco coated conductors with and without artificial pinning centers. Supercond. Sci. Technol.31, 044006. doi:10.1088/1361-6668/aaadf2
Foletti, C., Scaddozzo, G., Tarantino, M., Gessi, A., Bertacci, G., Agostini, P., et al. (2006). ENEA experience in LBE technology. J. Nucl. Mater.356, 264–272. doi:10.1016/j.jnucmat.2006.05.020
Fradera, J., Sádaba, S., Calvo, F., Ha, S., Merriman, S., Gordillo, P., et al. (2021). Pre-conceptual design of an encapsulated breeder commercial blanket for the STEP fusion reactor. Fusion Eng. Des.172, 112909. doi:10.1016/j.fusengdes.2021.112909
Freidberg, J. P. (2007). Plasma physics and fusion energy. Cambridge University Press. doi:10.1017/CBO9780511755705
Fujioka, S., Zhang, Z., Ishihara, K., Shigemori, K., Hironaka, Y., Johzaki, T., et al. (2013). Kilotesla magnetic field due to a capacitor-coil target driven by high power laser. Sci. Rep.3, 1–7. doi:10.1038/srep01170
Fujita, T., Hatae, T., Oikawa, T., Takeji, S., Shirai, H., Koide, Y., et al. (1998). High performance reversed shear plasmas with a large radius transport barrier in JT-60U. Nucl. fusion38, 207. doi:10.1088/0029-5515/38/2/305
Ge, M., Friedrich, J., and Vigna, L. (2020). 4 charts explain greenhouse gas emissions by countries and sectors. Available at: https://www.wri.org/insights/4-charts-explain-greenhouse-gas-emissions-countries-and-sectors (Accessed 05 02, 2023).
Genta, G., and Kezerashvili, R. Y. (2020). Achieving the required mobility in the solar system through direct fusion drive. Acta Astronaut.173, 303–309. doi:10.1016/j.actaastro.2020.04.047
Giancarli, L., Abdou, M., Campbell, D., Chuyanov, V., Ahn, M., Enoeda, M., et al. (2012). Overview of the ITER TBM program. Fusion Eng. Des.87, 395–402. doi:10.1016/j.fusengdes.2011.11.005
Gota, H., Binderbauer, M., Tajima, T., Smirnov, A., Putvinski, S., Tuszewski, M., et al. (2021). Overview of C-2W: High temperature, steady-state beam-driven field-reversed configuration plasmas. Nucl. Fusion61, 106039. doi:10.1088/1741-4326/ac2521
Greenwald, M., Gwinn, D., Milora, S., Parker, J., Parker, R., Wolfe, S., et al. (1984). Energy confinement of high-density pellet-fueled plasmas in the Alcator C tokamak. Phys. Rev. Lett.53, 352. doi:10.1103/PhysRevLett.53.352
Griffiths, T., Pearson, R., Bluck, M., and Takeda, S. (2022). The commercialisation of fusion for the energy market: A review of socio-economic studies. Prog. Energy 4 (4), 042008. doi:10.1088/2516-1083/ac84bf
Grove, D., Arunasalam, V., Bol, K., Bretz, N., Brusati, M., Cohen, S., et al. (1977). Experimental results of the PLT tokamak. Plasma Phys. Control. Nucl. Fusion Res.1, 21–32.
Gryaznevich, M., and Asunta, O. (2017). Overview and status of construction of ST40. Fusion Eng. Des.123, 177–180. doi:10.1016/j.fusengdes.2017.03.011
Guo, D., Zang, H., He, C., Zhang, P., Li, T., Cao, X., et al. (2015). Preliminary studies on the emulation of 14MeV neutron irradiation in sic with heavy ions. Fusion Eng. Des.100, 274–279. doi:10.1016/j.fusengdes.2015.06.085
Hartwig, Z. S., Vieira, R. F., Sorbom, B. N., Badcock, R. A., Bajko, M., Beck, W. K., et al. (2020). VIPER: An industrially scalable high-current high-temperature superconductor cable. Supercond. Sci. Technol.33, 11LT01. doi:10.1088/1361-6668/abb8c0
Haubenreich, P. N., and Engel, J. (1970). Experience with the molten-salt reactor experiment. Nucl. Appl. Technol.8, 118–136. doi:10.13182/NT8-2-118
Hawryluk, R., Batha, S., Blanchard, W., Beer, M., Bell, M., Bell, R., et al. (1998). Fusion plasma experiments on TFTR: A 20 year retrospective. Phys. Plasmas5, 1577–1589. doi:10.1063/1.872825
Heller, R., Blanchier, P., Fietz, W. H., and Wolf, M. J. (2019). Quench analysis of the HTS crossconductor for a toroidal field coil. IEEE Trans. Appl. Supercond.29, 1–11. doi:10.1109/TASC.2019.2917154
Hong, S.-H. (2022). A review of DEMO reactor concepts: Open questions and issues. AAPPS Bull.32, 1–15. doi:10.1007/s43673-022-00040-9
Hora, H., Korn, G., Giuffrida, L., Margarone, D., Picciotto, A., Krasa, J., et al. (2015). Fusion energy using avalanche increased boron reactions for block-ignition by ultrahigh power picosecond laser pulses. Laser Part. Beams33, 607–619. doi:10.1017/S0263034615000634
Hora, H., Eliezer, S., Kirchhoff, G., Nissim, N., Wang, J., Lalousis, P., et al. (2017). Road map to clean energy using laser beam ignition of boron-hydrogen fusion. Laser Part. Beams35, 730–740. doi:10.1017/S0263034617000799
IAEA (2021). Fusion device information system - FusDIS (2021). Data retrieved from IAEA Fusion Portal, https://nucleus.iaea.org/sites/fusionportal/Pages/FusDIS.aspx.
Ihli, T., Basu, T., Giancarli, L., Konishi, S., Malang, S., Najmabadi, F., et al. (2008). Review of blanket designs for advanced fusion reactors. Fusion Eng. Des.83, 912–919. doi:10.1016/j.fusengdes.2008.07.039
Iliffe, W., Peng, N., Brittles, G., Bateman, R., Webb, R., Grovenor, C., et al. (2021). In-situ measurements of the effect of radiation damage on the superconducting properties of coated conductors. Supercond. Sci. Technol.34, 09LT01. doi:10.1088/1361-6668/ac1523
ITER (2023). ITER cost. Available at: https://www.iter.org/FAQ#collapsible_5 (Accessed 04 05, 2023).
Jassby, D. (2022). The quest for fusion energy. Available at: https://inference-review.com/article/the-quest-for-fusion-energy (Accessed 01 19, 2023).
JET Team (1992). Fusion energy production from a deuterium-tritium plasma in the JET tokamak. Nucl. Fusion32, 187. doi:10.1088/0029-5515/32/2/I01
Kamada, Y., Barabaschi, P., and Ishida, S. (2013). Progress of the JT-60SA project. Nucl. Fusion53, 104010. doi:10.1088/0029-5515/53/10/104010
Kim, Y., Kim, W., and Kim, M. (2014). An international comparative analysis of public acceptance of nuclear energy. Energy Policy66, 475–483. doi:10.1016/j.enpol.2013.11.039
Kim, K., Im, K., Kim, H., Oh, S., Park, J., Kwon, S., et al. (2015). Design concept of K-DEMO for near-term implementation. Nucl. Fusion55, 053027. doi:10.1088/0029-5515/55/5/053027
Kishimoto, H., Ishida, S., Kikuchi, M., and Ninomiya, H. (2005). Advanced tokamak research on JT-60. Nucl. Fusion45, 986. doi:10.1088/0029-5515/45/8/026
Knaster, J., Moeslang, A., and Muroga, T. (2016). Materials research for fusion. Nat. Phys.12, 424–434. doi:10.1038/nphys3735
Kovari, M., Coleman, M., Cristescu, I., and Smith, R. (2017). Tritium resources available for fusion reactors. Nucl. Fusion58, 026010. doi:10.1088/1741-4326/aa9d25
Kramer, D. (2018). ITER disputes DOE’s cost estimate of fusion project. Phys. Today16. doi:10.1063/PT.6.2.20180416a
Kuang, A., Cao, N., Creely, A. J., Dennett, C. A., Hecla, J., LaBombard, B., et al. (2018). Conceptual design study for heat exhaust management in the arc fusion pilot plant. Fusion Eng. Des.137, 221–242. doi:10.1016/j.fusengdes.2018.09.007
Kulcinski, G., and Schmitt, H. H. (1988). “The moon: An abundant source of clean and safe fusion fuel for the 21st century,” in Lunar helium-3 and fusion power (NASA, Lewis Research Center).
Laberge, M. (2019). Magnetized target fusion with a spherical tokamak. J. Fusion Energy38, 199–203. doi:10.1007/s10894-018-0180-3
Lee, G., Kwon, M., Doh, C., Hong, B., Kim, K., Cho, M., et al. (2001). Design and construction of the KSTAR tokamak. Nucl. Fusion41, 1515. doi:10.1088/0029-5515/41/10/318
Leung, K.-N., Leung, J. K., and Melville, G. (2018). Feasibility study on medical isotope production using a compact neutron generator. Appl. Radiat. Isotopes137, 23–27. doi:10.1016/j.apradiso.2018.02.026
Luxon, J. (2002). A design retrospective of the DIII-D tokamak. Nucl. Fusion42, 614. doi:10.1088/0029-5515/42/5/313
Margarone, D., Bonvalet, J., Giuffrida, L., Morace, A., Kantarelou, V., Tosca, M., et al. (2022). In-target proton–boron nuclear fusion using a PW-class laser. Appl. Sci.12, 1444. doi:10.3390/app12031444
Martelli, E., Del Nevo, A., Arena, P., Bongiovì, G., Caruso, G., Di Maio, P., et al. (2018). Advancements in DEMO WCLL breeding blanket design and integration. Int. J. Energy Res.42, 27–52. doi:10.1002/er.3750
McCrory, R., Bahr, R., Betti, R., Boehly, T., Collins, T., Craxton, R., et al. (2001). OMEGA ICF experiments and preparation for direct drive ignition on nif. Nucl. Fusion41, 1413. doi:10.1088/0029-5515/41/10/309
McLean, H. S., Higginson, D., Schmidt, A., Tummel, K., Shumlak, U., Nelson, B., et al. (2017). “A reactor development scenario for the fuze sheared-flow stabilized z-pinch,” in APS Division of Plasma Physics Meeting 2017.
Mehlhorn, T., Cipiti, B., Olson, C., and Rochau, G. (2008). Fusion–fission hybrids for nuclear waste transmutation: A synergistic step between gen-IV fission and fusion reactors. Fusion Eng. Des.83, 948–953. doi:10.1016/j.fusengdes.2008.05.003
Menard, J., Allain, J., Battaglia, D., Bedoya, F., Bell, R., Belova, E., et al. (2017). Overview of nstx upgrade initial results and modelling highlights. Nucl. Fusion57, 102006. doi:10.1088/1741-4326/aa600a
Merriman, S., Abraham, J., Bailey, J., Billingsley, J., Edwards, B., Hagues, J., et al. (2023). Integrated simulation for the preconceptual optioneering of the step breeder blanket design. Fusion Eng. Des.189, 113423. doi:10.1016/j.fusengdes.2023.113423
Meschini, S. (2021). Sustainability of compact, high magnetic field fusion reactors: Environment, society and economy. FRESENIUS Environ. Bull.30, 5974–5984.
Mignacca, B., and Locatelli, G. (2020). Economics and finance of molten salt reactors. Prog. Nucl. Energy129, 103503. doi:10.1016/j.pnucene.2020.103503
Miley, G. H., and Murali, S. K. (2014). Inertial electrostatic confinement (IEC) fusion, Fundamentals and Applications. Springer.
Molodyk, A., Samoilenkov, S., Markelov, A., Degtyarenko, P., Lee, S., Petrykin, V., et al. (2021). Development and large volume production of extremely high current density YBa2Cu3O7 superconducting wires for fusion. Sci. Rep.11, 1–11. doi:10.1038/s41598-021-81559-z
Monsler, M. J., Hovingh, J., Cook, D. L., Frank, T. G., and Moses, G. A. (1981). An overview of inertial fusion reactor design. Nucl. Technol. - Fusion1, 302–358. doi:10.13182/FST81-A19936
Morley, N., Abdou, M., Anderson, M., Calderoni, P., Kurtz, R., Nygren, R., et al. (2006). Overview of fusion nuclear technology in the US. Fusion Eng. Des.81, 33–43. doi:10.1016/j.fusengdes.2005.06.359
Mossman, A., Laberge, M., Reynolds, M., Howard, S., McNally, C., Carbajal, L., et al. (2022). “Magnetized target fusion using mechanically-driven liquid metal liner,” in 64th annual meeting of the APS division of plasma physics, Spokane, Washington, October 17–21, 2022.
Mukhovatov, V., Shimomura, Y., Polevoi, A., Shimada, M., Sugihara, M., Bateman, G., et al. (2003). Comparison of iter performance predicted by semi-empirical and theory-based transport models. Nucl. fusion43, 942. doi:10.1088/0029-5515/43/9/318
Muroga, T., Gasparotto, M., and Zinkle, S. (2002). Overview of materials research for fusion reactors. Fusion Eng. Des.61-62, 13–25. doi:10.1016/S0920-3796(02)00219-3
NASEM (2019). Final report of the committee on a strategic plan for US burning plasma research. Whashington, DC: National Academies Press. doi:10.17226/25331
NASEM (2021). Bringing fusion to the us grid. Washington, DC: The National Academies Press. doi:10.17226/25991
Nicholas, T., Davis, T., Federici, F., Leland, J., Patel, B., Vincent, C., et al. (2021). Re-examining the role of nuclear fusion in a renewables-based energy mix. Energy Policy149, 112043. doi:10.1016/j.enpol.2020.112043
O’Shea, P., Laberge, M., Donaldson, M., Delage, M., Mossman, A., Reynolds, M., et al. (2018). “Magnetized target fusion at general fusion: An overview,” in 60th Annual Meeting of the APS Division of Plasma Physics (Portland, Oregon, USA), 5–9.
Oliphant, M. L. E., Harteck, P., and Rutherford, E. (1934). Transmutation effects observed with heavy hydrogen. Proc. R. Soc. Lond. Ser. A, Contain. Pap. a Math. Phys. Character144, 692–703.
Omoto, A. (2005). Nuclear power for sustainable development and relevant IAEA activities for the future. Prog. Nucl. Energy47, 16–26. doi:10.1016/j.pnucene.2005.05.056
Ongena, J., Koch, R., Wolf, R., and Zohm, H. (2016). Magnetic-confinement fusion. Nat. Phys.12, 398–410. doi:10.1038/nphys3745
Ongena, J. (2015). Fusion: A true challenge for an enormous reward. EPJ Web Conf.98, 05004. doi:10.1051/epjconf/20159805004
Otuka, N., Dupont, E., Semkova, V., Pritychenko, B., Blokhin, A., Aikawa, M., et al. (2014). Towards a more complete and accurate experimental nuclear reaction data library (EXFOR): International collaboration between nuclear reaction data centres (NRDC). Nucl. Data Sheets120, 272–276. doi:10.1016/j.nds.2014.07.065
Pamela, J., Solano, E. R., and Contributors, J. E. (2003). Overview of JET results. Nucl. Fusion43, 1540. doi:10.1088/0029-5515/43/12/002
Parish, T. A., and Davidson, J. W. (1980). Reduction in the toxicity of fission product wastes through transmutation with deuterium-tritium fusion neutrons. Nucl. Technol.47, 324–342. doi:10.13182/NT80-A32436
Park, J. H., Pereslavtsev, P., Konobeev, A., and Wegmann, C. (2021). Statistical analysis of tritium breeding ratio deviations in the demo due to nuclear data uncertainties. Appl. Sci.11. doi:10.3390/app11115234
Parrell, J. A., Field, M. B., Zhang, Y., and Hong, S. (2004). Nb3Sn conductor development for fusion and particle accelerator applications. AIP Conf. Proc.711, 369–375. doi:10.1063/1.1774590
Pashchenko, A., Wienke, H., and Ganesan, S. (1996). Fendl: International reference nuclear data library for fusion applications. J. Nucl. Mater.233-237, 1601–1606. doi:10.1016/S0022-3115(96)00153-5
Peacock, N., Robinson, D., Forrest, M., Wilcock, P., and Sannikov, V. (1969). Measurement of the electron temperature by thomson scattering in tokamak t3. Nature224, 488–490. doi:10.1038/224488a0
Pearson, R. J., Antoniazzi, A. B., and Nuttall, W. J. (2018). Tritium supply and use: A key issue for the development of nuclear fusion energy. Fusion Eng. Des.136, 1140–1148. doi:10.1016/j.fusengdes.2018.04.090
Post, R. F. (1976). Nuclear fusion. Annu. Rev. Energy1, 213–255. doi:10.1146/annurev.eg.01.110176.001241
Prager, S. C. (2019). Nuclear fusion power – An overview of history, present and future. Int. J. Adv. Netw. Monit. Controls4, 1–10. doi:10.21307/ijanmc-2019-064
Puliga, G., Manzini, R., Lazzarotti, V., and Batistoni, P. (2020). Successfully managing SMEs collaborations with public research institutes: The case of ITER fusion projects. Innovation22, 353–376. doi:10.1080/14479338.2019.1685889
Razin, Y. S., Pajer, G., Breton, M., Ham, E., Mueller, J., Paluszek, M., et al. (2014). A direct fusion drive for rocket propulsion. Acta Astronaut.105, 145–155. doi:10.1016/j.actaastro.2014.08.008
Robbins, W. (1991). “An historical perspective of the NERVA nuclear rocket engine technology program,” in Conference on Advanced SEI Technologies, 3451.
Romanelli, F., Barabaschi, P., Borba, D., Federici, G., Horton, L., Neu, R., et al. (2012). Fusion electricity: A roadmap to the realization of fusion energy. Available at: https://core.ac.uk/reader/53866130. (Accessed 04 26, 2023).
Rowcliffe, A., Garrison, L., Yamamoto, Y., Tan, L., and Katoh, Y. (2018). Materials challenges for the fusion nuclear science facility. Fusion Eng. Des.135, 290–301. doi:10.1016/j.fusengdes.2017.07.012
Şahin, S., and Übeyli, M. (2004). Lwr spent fuel transmutation in a high power density fusion reactor. Ann. Nucl. Energy31, 871–890. doi:10.1016/j.anucene.2003.11.003
Sawan, M., and Abdou, M. (2006). Physics and technology conditions for attaining tritium self-sufficiency for the dt fuel cycle. Fusion Eng. Des.81, 1131–1144. doi:10.1016/j.fusengdes.2005.07.035
Segantin, S., Testoni, R., and Zucchetti, M. (2019). The lifetime determination of ARC reactor as a load-following plant in the energy framework. Energy Policy126, 66–75. doi:10.1016/j.enpol.2018.11.010
Segantin, S., Testoni, R., and Zucchetti, M. (2020). ARC reactor - neutron irradiation analysis. Fusion Eng. Des.159, 111792. doi:10.1016/j.fusengdes.2020.111792
Sharma, A. R., Chandra, D., Chaturvedi, S., Ganesan, S., and Wienke, H. (2001). Re-investigations of integral neutron multiplication experiments with 14 mev neutrons in lead. Fusion Eng. Des.55, 501–512.
Shiraga, H., Fujioka, S., Nakai, M., Watari, T., Nakamura, H., Arikawa, Y., et al. (2011). Fast ignition integrated experiments with Gekko and LFEX lasers. Plasma Phys. Control. Fusion53, 124029. doi:10.1088/0741-3335/53/12/124029
Skyrme, D., and Amidon, D. (1997). The knowledge agenda. J. Knowl. Manag.1, 27–37. doi:10.1108/13673279710800709
Slough, J., Votroubek, G., and Pihl, C. (2011). Creation of a high-temperature plasma through merging and compression of supersonic field reversed configuration plasmoids. Nucl. Fusion51, 053008.
Slough, J., Pancotti, A., Kirtley, D., Pihl, C., and Pfaff, M. (2012). Nuclear propulsion through direct conversion of fusion energy: The fusion driven rocket. Tech. Rep. HQ-E-DAA-TN33791. Available at: https://www.nasa.gov/directorates/spacetech/niac/2012_Phase_II_fusion_driven_rocket/(Accessed 04 26, 2023).
Slough, J. (2009). Final report on the magnetized target fusion collaboration. Tech. Rep. DOE/ER–54753. Univeristy of Washington. Available at: https://inis.iaea.org/search/search.aspx?orig_q=RN:45031556 (Accessed 04 26, 2023).
Slough, J. T. (2018). The fusion driven rocket: Nuclear propulsion through direct conversion of fusion energy. Tech. rep. Available at: https://ntrs.nasa.gov/citations/20190001178 (Accessed 04 26, 2023).
Smirnov, V. (2009). Tokamak foundation in USSR/Russia 1950–1990. Nucl. fusion50, 014003. doi:10.1088/0029-5515/50/1/014003
Sorbom, B., Ball, J., Palmer, T., Mangiarotti, F., Sierchio, J., Bonoli, P., et al. (2015). Arc: A compact, high-field, fusion nuclear science facility and demonstration power plant with demountable magnets. Fusion Eng. Des.100, 378–405. doi:10.1016/j.fusengdes.2015.07.008
Stacey, W. M., Mandrekas, J., Hoffman, E. A., Kessler, G. P., Kirby, C. M., Mauer, A. N., et al. (2002). A fusion transmutation of waste reactor. Fusion Sci. Technol.41, 116–140. doi:10.13182/FST02-A207
Stambaugh, R. D., Chan, V. S., Miller, R. L., and Schaffer, M. J. (1998). The spherical tokamak path to fusion power. Fusion Technol.33, 1–21. doi:10.13182/FST33-1
Stepanov, A. D., Shumlak, U., McLean, H. S., Nelson, B. A., Claveau, E. L., Forbes, E. G., et al. (2020). Flow z-pinch plasma production on the fuze experiment. Phys. Plasmas27, 112503. doi:10.1063/5.0020481
Stodiek, W. (1985). Experiments on the st tokamak. Nucl. fusion25, 1161. doi:10.1088/0029-5515/25/9/028
Stroth, U., Adamek, J., Aho-Mantila, L., Äkäslompolo, S., Amdor, C., Angioni, C., et al. (2013). Overview of ASDEX upgrade results. Nucl. Fusion53, 104003. doi:10.1088/0029-5515/53/10/104003
Sutherland, D., Jarboe, T., Morgan, K., Pfaff, M., Lavine, E., Kamikawa, Y., et al. (2014). The dynomak: An advanced spheromak reactor concept with imposed-dynamo current drive and next-generation nuclear power technologies. Fusion Eng. Des.89, 412–425. doi:10.1016/j.fusengdes.2014.03.072
Sviatoslavsky, I. (1988). “Processes and energy costs for mining lunar helium-3,” in Lunar helium-3 and fusion power (NASA, Lewis Research Center).
Sykes, A.the START team, the NBI teamthe MAST teamthe Theory team (1999). The spherical tokamak programme at culham. Nucl. Fusion39, 1271. doi:10.1088/0029-5515/39/9Y/305
Sykes, A. (2015). “Compact tokamak fusion,” in 2015 IEEE 15th International Conference on Environment and Electrical Engineering (EEEIC), 401–403. doi:10.1109/EEEIC.2015.7165195
Takase, Y., Ejiri, A., Fujita, T., Hanada, K., Idei, H., Nagata, M., et al. (2022). Overview of coordinated spherical tokamak research in Japan. Nucl. Fusion62, 042011. doi:10.1088/1741-4326/ac29cf
Tanaka, S. (2006). Overview of research and development activities on fusion nuclear technologies in Japan. Fusion Eng. Des.81, 13–24. doi:10.1016/j.fusengdes.2005.07.025
Teyber, R., Weiss, J., Marchevsky, M., Prestemon, S., and van der Laan, D. (2022). Current distribution monitoring enables quench and damage detection in superconducting fusion magnets. Sci. Rep.12, 22503. doi:10.1038/s41598-022-26592-2
TFR Group (1985). TFR, the tokamak of fontenay-aux-roses. Nucl. Fusion25, 1025. doi:10.1088/0029-5515/25/9/005
Thonemann, P., Butt, E., Carruthers, R., Dellis, A., Fry, D., Gibson, A., et al. (1958). Controlled release of thermonuclear energy: Production of high temperatures and nuclear reactions in a gas discharge. Nature181, 217–220.
Tobita, K., Utoh, H., Hiwatari, R., Miyoshi, Y., Tokunaga, S., Sakamoto, Y., et al. (2019). Conceptual design of Japan’s fusion DEMO reactor (JADEMO) and superconducting coil issues. J. Phys. Conf. Ser.1293, 012078. doi:10.1088/1742-6596/1293/1/012078
Torsello, D., Casalegno, V., Divitini, G., Ghigo, G., Gerbaldo, R., Fracasso, M., et al. (2022a). Triple ion beam irradiation of glass-ceramic materials for nuclear fusion technology. J. Nucl. Mater.567, 153783. doi:10.1016/j.jnucmat.2022.153783
Torsello, D., Gambino, D., Gozzelino, L., Trotta, A., and Laviano, F. (2022b). Expected radiation environment and damage for ybco tapes in compact fusion reactors. Supercond. Sci. Technol.36, 014003. doi:10.1088/1361-6668/aca369
U.S. Geological Survey (2023). Mineral commodity summaries, 2023. Government Printing Office. doi:10.3133/mcs2023
Uglietti, D. (2019). A review of commercial high temperature superconducting materials for large magnets: From wires and tapes to cables and conductors. Supercond. Sci. Technol.32, 053001. doi:10.1088/1361-6668/ab06a2
UKAEA (2022). Review of First Light Fusion Ltd’s experimental report ‘validate production of neutrons from gas-gun driven targets’. Tech. rep. FTBU-EXT-RPT-000010. Available at: https://firstlightfusion.com/assets/uploads/images/WP4_report_i1.pdf. Accessed: 2023-01-19.
UKAEA (2023a). First light fusion to build demonstration facility at ukaea’s culham campus. Available at: https://www.gov.uk/government/news/first-light-fusion-to-build-demonstration-facility-at-ukaeas-culham-campus (Accessed 04 05, 2023).
UKAEA (2023b). General fusion’s fusion energy demonstration receives consent at ukaea’s culham campus. Available at: https://ccfe.ukaea.uk/general-fusions-fusion-energy-demonstration-receives-consent-at-ukaeas-culham-campus/(Accessed 04 05, 2023).
UKAEA (2023c). Tokamak energy’s new advanced fusion prototype to be built at ukaea’s culham campus. Available at: https://ccfe.ukaea.uk/tokamak-energys-new-advanced-fusion-prototype-to-be-built-at-ukaeas-culham-campus/(Accessed 04 05, 2023).
Un, (2015). The 17 goals. Available at: https://sdgs.un.org/goals (Accessed 04 05, 2023).
Wan, Y., Li, J., Liu, Y., Wang, X., Chan, V., Chen, C., et al. (2017). Overview of the present progress and activities on the CFETR. Nucl. Fusion57, 102009. doi:10.1088/1741-4326/aa686a
Wang, Q., Liu, J., Zheng, J., Qin, J., Ma, Y., Xu, Q., et al. (2021). Progress of ultra-high-field superconducting magnets in China. Supercond. Sci. Technol.35, 023001. doi:10.1088/1361-6668/ac3f9b
Willingale, L., Petrov, G., Maksimchuk, A., Davis, J., Freeman, R., Joglekar, A., et al. (2011). Comparison of bulk and pitcher-catcher targets for laser-driven neutron production. Phys. Plasmas18, 083106. doi:10.1063/1.3624769
Wilson, H., Chapman, I., Denton, T., Morris, W., Patel, B., Voss, G., et al. (2020). “STEP—On the pathway to fusion commercialization,” in Commercialising fusion energy (IOP Publishing), 2053-2563, 8–1–8–18. doi:10.1088/978-0-7503-2719-0ch8
Wolf, M. J., Heller, R., Fietz, W. H., and Weiss, K.-P. (2019a). Design and analysis of HTS subsize-conductors for quench investigations towards future HTS fusion magnets. Cryogenics104, 102980. doi:10.1016/j.cryogenics.2019.102980
Wolf, R., Alonso, A., Äkäslompolo, S., Baldzuhn, J., Beurskens, M., Beidler, C., et al. (2019b). Performance of Wendelstein 7-X stellarator plasmas during the first divertor operation phase. Phys. Plasmas26, 082504. doi:10.1063/1.5098761
Wu, S.the EAST team (2007). An overview of the east project. Fusion Eng. Des.82, 463–471. doi:10.1016/j.fusengdes.2007.03.012
Yoshikawa, K., Noma, T., and Yamamoto, Y. (1991). Direct-energy conversion from high-energy ions through interaction with electromagnetic fields. Fusion Technol.19, 870–875. doi:10.13182/FST91-A29454
Youssef, M. Z., and Abdou, M. A. (1986). Uncertainties in prediction of tritium breeding in candidate blanket designs due to present uncertainties in nuclear data base. Fusion Technol.9, 286–307. doi:10.13182/FST86-A24716
Zappatore, A., Heller, R., Savoldi, L., Wolf, M., and Zanino, R. (2020). A new model for the analysis of quench in HTS cable-in-conduit conductors based on the twisted-stacked-tape cable concept for fusion applications. Supercond. Sci. Technol.33, 065004. doi:10.1088/1361-6668/ab895b
Zhai, Y., van der Laan, D., Connolly, P., and Kessel, C. (2021). Conceptual design of HTS magnets for fusion nuclear science facility. Fusion Eng. Des.168, 112611. doi:10.1016/j.fusengdes.2021.112611
Zucchetti, M., Di Pace, L., El-Guebaly, L., Han, J.-H., Kolbasov, B., Massaut, V., et al. (2013). Recent advances in fusion radioactive material studies. Fusion Eng. Des.88, 652–656. doi:10.1016/j.fusengdes.2013.02.050
Keywords: fusion energy, commercial fusion, fusion technologies, magnetic confinement, inertial confinement, magnetized target fusion
Citation: Meschini S, Laviano F, Ledda F, Pettinari D, Testoni R, Torsello D and Panella B (2023) Review of commercial nuclear fusion projects. Front. Energy Res. 11:1157394. doi: 10.3389/fenrg.2023.1157394
Received: 02 February 2023; Accepted: 18 May 2023;
Published: 07 June 2023.
Edited by:
Srinivasan Ganesan, Ex-Bhabha Atomic Research Centre, IndiaReviewed by:
Rudrodip Majumdar, National Institute of Advanced Studies, IndiaCopyright © 2023 Meschini, Laviano, Ledda, Pettinari, Testoni, Torsello and Panella. This is an open-access article distributed under the terms of the Creative Commons Attribution License (CC BY). The use, distribution or reproduction in other forums is permitted, provided the original author(s) and the copyright owner(s) are credited and that the original publication in this journal is cited, in accordance with accepted academic practice. No use, distribution or reproduction is permitted which does not comply with these terms.
*Correspondence: Bruno Panella, YnJ1bm8ucGFuZWxsYUBwb2xpdG8uaXQ=
Disclaimer: All claims expressed in this article are solely those of the authors and do not necessarily represent those of their affiliated organizations, or those of the publisher, the editors and the reviewers. Any product that may be evaluated in this article or claim that may be made by its manufacturer is not guaranteed or endorsed by the publisher.
Research integrity at Frontiers
Learn more about the work of our research integrity team to safeguard the quality of each article we publish.