- 1Civil, Maritime and Environmental Engineering, University of Southampton, Southampton, United Kingdom
- 2Department of Applied Sciences, University of the West of England, Bristol, United Kingdom
Microbial fuel cells (MFC) are an emerging green technology which offers several comparative advantages over other technologies for utilizing biomass. It is a technology that treats (cleans) wet organic waste, converting chemical energy to electricity that is used for connected peripherals and target applications. The main advantage is the technology’s ability to utilise wet biomass in suspension or in solution (i.e., too wet to burn) and change the biomass directly into bioenergy in the form of electricity. All other technologies either combust the biomass directly (e.g., wood fuel) or change the biomass into refined fuels which are then combusted or fed to chemical fuel cells to generate heat or electricity. Excluding methane production from biomass, and fermentation leading to hydrogen production, all other biomass/biofuel technologies utilize dry plant matter, which mainly consists of cellulose or lignocellulose and they cannot directly utilize sludge or slurries of organic detritus material. The substrates used for MFCs are not traditionally made into organic fuels, as with other biomass technologies, but are used directly as fuel, recasting the “waste” suspensions and solutions, and promoting them into fuels themselves. To a stack of MFCs, a polluted river, landfill leachate or farmland run-off, can all be reassigned as fuel. This wet fuel is widespread around the planet, the amounts found and the energy contained within are significant, and the cost as a fuel is close to zero. This review gives a general overview of biomass energy along with extraction techniques and compares advantages and disadvantages of MFCs with other biomass technologies for producing electrical energy.
Introduction
Plants and photosynthetic microorganisms are examples of autotrophs that use sunlight to make their own organic molecules by fixing carbon dioxide. Because they make their own food by photosynthesis, they are the main form of primary producers of biomass, acting as the conduit for energy and bio-matter to enter food chains or webs (Mestre et al., 2022). A food chain describes which species feed off which other species whilst a food web describes the food chains that contribute to making a whole ecosystem. The position of a species within an ecosystem is referred to as a trophic level in the web or food chain. The base of the trophic pyramid is made from primary producers, these being autotrophic species that are nearly always photosynthetic (plants, bacteria or algae). The next level above the primary producers are the primary consumers, typically herbivores. Further levels include secondary and then tertiary consumers typically omnivores and/or carnivores, with the top level being termed the apex predators. Each food chain is typically composed of five or six trophic levels. Trophic level zero refers to species that do not fit into the existing trophic levels. These species are the detritivores or scavengers. They play a vital role in the flow of carbon and energy through an ecosystem by decomposing dead organisms into their constituent organic or inorganic materials, which become available as nutrients to the primary producers. The detritivores or scavengers include earthworms, termites, and millipedes although most decomposers are fungi, protozoa and bacteria. Bacteria are the energy conversion drivers inside microbial fuel cells (MFCs), which when employed as integrated living power sources in robots (Ieropoulos, Greenman and Melhuish, 2010), introduce a new hybrid system, i.e., bio-robots or “Symbots,” on trophic level zero.
Allometric scaling
All lifeforms are subject to allometric scaling, which also determines their position in an ecosystem. With respect to primary and secondary biomass, smaller organisms (whether multicellular or unicellular) grow faster than larger lifeforms. The study of the laws of growth rate versus size originate from the allometric formula by Otto Snell (Snell, 1892) although the term “allometric scaling” was introduced by Huxley and Teissier (1936) following the work of Kleiber (1932). The concept of allometry extends to all forms of life, although the relationship between growth rate and size does not apply uniformly, in that the relationship for prokaryotes is superlinear, for protists is linear and for metazoa is sublinear (Delong et al., 2010). As recently reported, allometric scaling can also be applied to hybrid systems, i.e., non-living vessels containing living microorganisms; here we make specific reference to Microbial Fuel Cells (Greenman and Ieropoulos, 2017). Allometric scaling is the metric by which ecosystems operate and its significance will also be discussed in the Conclusion section.
With regard to biomass transformation, a wide range of processes can be applied to plants, wood and waste including direct combustion, co-firing, gasification, pyrolysis, fermentation and anaerobic decomposition. However, all processes involving heat or combustion are inefficient if the biomass is too wet, and has been the motivation for developing efficient biotransformation techniques, which are listed and discussed below.
Biomass and biofuels
Biomass generation removes an equivalent amount of CO2 from the atmosphere to that emitted from combustion, which means that in theory, this is truly a net-zero process (World Bioenergy Association, 2019). Fossil fuel combustion for heat, electricity, and transportation fuels, contributed ca. 80% of global GHG emissions in 2017 (World Bioenergy Association, 2019). Bioenergy production worldwide reached nearly 584 TWh in 2020 (Black et al., 2021). Globally in 2019, bioenergy accounted for about 11.6% of total energy consumption. With regard to the bioenergy-biomass sector, the largest source is from burning wood (or wood related products, wood chips, sawdust, coppiced branches, recycled cardboard and paper) allowing energy to be extracted by combustion with oxygen, from small fires to large incinerators. The energy is released in the form of heat, which is converted to electricity through inefficient generators. Nevertheless, all biomass reserves are potentially renewable, remove CO2 and produce O2, although slow growing tree species are not efficient for biomass growth, as their primary purpose is fruit production for human and animal consumption. Micro-algae, microbial sludge and microbial cultures have much higher productivity per unit of biomass than types of grass or plant and are totally renewable. Plants, wood, and waste are the most common forms of biomass material for producing energy. These types of biomass feedstock can be combusted to create heat (direct energy) which via steam production (as the driving force for turbines) can be used to produce electricity. These biomass substrates can also be processed into biofuels (indirect energy).
Biomass mainly in the form of plants and algae rather than dead animal matter, is perhaps the only renewable source that can be converted into ethanol and/or biodiesel. These two biofuels—currently produced by gasification in the US, Austria and Sweden-are currently used for transportation. Based mainly on the biomass, biofuels are classified broadly into three major generations (Lee and Lavoie, 2013). Biofuels of the first-generation come from the fermentation of carbohydrates (sugar beet, sugarcane, corn starch or wheat) to give bioethanol, an alcoholic fuel that when refined can be used directly in a conventional chemical fuel cell to produce electricity or serve as an additive to gasoline. The problem with first-generation fuels is that they are made from biomass that is generally edible by humans or animals. Ethanol requires significant land use; an acre of corn, for example, produces ∼400 gallons of ethanol but limits the use of this land to only corn, which in turn implies intensive use of pesticides. Biodiesel is produced from oily plants and seeds, including soy, palm and canola. Alternatively, used edible oils, such as vegetable oil or recycled cooking fat, can also be used following esterification and transesterification using methanol.
Second-generation biofuels, can be produced from non-food-based biomass such as lignocellulosic agricultural waste and municipal waste. This is an advantage shadowed by economic uncertainty and technical complexity of the hydrolysis process required to overcome the chemical barriers and structural rigidity of lignocellulosic biomass. Lignin and cellulose have been reported as co-substrates in Microbial Fuel Cells for energy and biofuel generation, which gives the technology a competitive advantage and provides a possible alternative to the aforementioned challenges. When using the right bacteria, the otherwise recalcitrant lignocellulose is efficiently broken down.
The costs associated with bioethanol production depend mainly on the costs of feedstock substrates which give different yields depending on (a) the species of microbes chosen for the fermentation reactions (b) the addition of cellulase for enzymatic hydrolysis of cellulose into sugars, and (c) the nature of the pre-treatment (e.g., yield of sugars available for fermentation). Most current ethanol production is based on corn, starch and sugars but their use may not be desirable due to their edible value. In contrast, the straw of rice, wheat and corn as well as bagasse are now more commonplace because they contain cellulose, hemicellulose and lignin rather than edible sugars. In theory, there is sufficient rice straw in the world to produce 205 billion litres of bioethanol per year. Physicochemical treatment may include milling, grinding, cutting, thermal heating, microwaving, steam explosion, pyrolysing, chemical pre-treatment, wet oxidation, acid or alkali pre-treatment and the current cost of ethanol is $3.93/gallon, compared to $4.70/gallon for petrol (Sondhi et al., 2020).
Algae
The third generation of biofuels is based on photosynthetic organisms that do not require land but live in water, hydroponically, producing new or “primary” biomass. Microalgae can thrive in non-fresh i.e., salty, brackish water, contaminated with municipal, agricultural, industrial or even nuclear run-off. Microalgae are multiple times more efficient than terrestrial crops used for producing fuel. Third generation biofuels are also favoured because of the high growth rate of microalgae (vis allometric scaling) compared to any larger terrestrial plant; only microalgae can double their biomass every 1–2 days. The productivity is high compared to even the fastest of grass species. Moreover, they can grow in lagoons in marginal areas, which does not impose on the use of arable land for the production of food. The problem with microalgae lies when one needs a particular strain or species, especially strains that have been genetically modified (GM). Examples include strains capable of producing a high lipid content to make bio-diesel, and strains capable of synthesizing sugars, lactate and food supplements such as astaxanthin via photosynthesis (Shah et al., 2016). In these cases, they must be grown as a strict monoculture (i.e., be free of contamination) and (in GM strains) following lipid extraction, their DNA must be rendered before safe disposal or further utilization of cell debris. These steps can be very energy intensive, adding to the costs of production however, not entirely impossible. Sun et al. (2020) reported high growth rates for Synechococcus elongatus UTEX 2973, in photobioreactor volumes ranging from 50 mL to 100 L under non-sterile conditions, without contamination.
Algae (macro- and micro-algae) carry enormous potential for bio (mass)-energy. Seaweed or Chlorella, photosynthesise up to 30x faster than crops used for food or fuel, and do not impose on either land or freshwater. Although algae release carbon dioxide when burned, the amount is never more than what they fixed from carbon dioxide in the first place and the biomass can be replenished as a living organism through cultivation, releasing oxygen and absorbing pollutants and this would require less land than that needed for harvesting corn. Algae’s high-value lipids can be converted to biofuel, which has been the main objective in the race for alternative fuels, necessitating genetic modification of certain species for ever higher lipid content. Algal growth requires carbon dioxide, making the process an excellent CO2 scrubber. When algae are put under certain stress conditions to produce the desired by-product (for example, Haematococcus pluvialis producing astaxanthin; Shah et al., 2016), this comes at a high cost and still quite far from the current price of biodiesel (not from algae) of $5.34/gallon (US DoE, 2022).
Algal biodiesel (third-generation) is derived from microalgae or photosynthetic bacteria and has been considered as a viable option to the problem of energy insecurity and climate change and removing the need for fossil fuels. This is particularly important when taking into account that for every tonne of algal biomass produced, approximately 1.83 tonnes of carbon dioxide are fixed, whereas petroleum diesel carries a massive negative balance; this is a significant competitive advantage in the context of GHG externalities (Um and Kim, 2009).
The commercialisation of algal biomass production via aquafarming faces significant economic challenges. The current annual production is around 38 million litres (Karthikeyan et al., 2020). Co-production of microalgae is possible, whilst treating wastewater, or for biogas upgrading, and whilst producing value-added products (VAPs) including exopolysaccharides, protein or the pharmaceutical, astaxanthin. This can drastically reduce biodiesel production costs. For example, the co-production of astaxanthin and biolipids for diesel can reduce the cost of biodiesel production from $3.90 to $0.54/L (Rafa et al., 2021). The economic analysis reveals that although there are technical challenges, the strategy is cost-effective; both feasible and profitable. The cost of producing microalgal biodiesel can be lowered to $0.73/kg dry weight when cultivated in wastewater and $0.54/L when co-produced with astaxanthin (Rafa et al., 2021). Microalgae-based value-added products are estimated to rise to $53.43 billion in 2026 (Rahman, 2020). The recent (2022–2023) increase in global fossil fuel prices is helping to reduce the gap in production costs that persist between biodiesel and petroleum diesel.
Biomass alongside other combustible renewable sources are promising alternatives, being the fourth largest energy source behind fossil fuels (Lam et al., 2019; Hoang et al., 2022), with a good capacity to address global energy needs (Azevedo et al., 2019). On the other hand, various types of biodiesel (Mofijur et al., 2021), mostly produced from biomass sources via transesterification (Hazrat et al., 2022), make up >80% of total biofuel production (Yin et al., 2020), but the real advantages can be gained from microalgal produced biodiesel. This is due to the microorganisms’ ability to double biomass, through efficient sunlight, CO2 and water utilisation that results in rapid rates of lipid accumulation for harvesting all year-round (Mubarak et al., 2019; Yin et al., 2020). Table 1 shows the advantages and challenges of microalgae-based fuel.
Cost reduction in microalgal biodiesel production are constantly developing whether these are improvements in bioreactor design, optimisation of the physicochemical conditions for cultivation, choice in the strains and species that are supplied as inoculum and insights into the processes and factors that affect yield, efficient harvesting and extraction methods (Peng et al., 2020; Ananthi et al., 2021; Rafa et al., 2021). Although many cost reduction strategies have been applied to algal cultivation which can make up a significant proportion of the cost, other areas, particularly pre-treatment, harvesting and dewatering have also been considered (Kang et al., 2019). Scientists worldwide have been elaborating the case for fuels derived from microalgae to close knowledge gaps; in particular Um and Kim (Um and Kim, 2009) and Rafa et al. (Rafa et al., 2021).
Biomethane from biomass
When biomass decays anaerobically it has the tendency to produce (bio)-methane, following a chain of reactions involving initially hydrolysis, then acidogenesis and acetogenesis and finally methanogenesis; this biomethane can replace methane obtained from fossil fuels. However, methane is a very potent greenhouse gas and any leakage to the atmosphere is a problem. The first description of an anaerobic methane digester was in 1859, more than 160 years ago, in India (Marsh, 2008). Despite it being one of the earliest methods for producing a biofuel, it is not classed as a first-generation biofuel because it does not utilise edible plants. It can however be classed as a second-generation biofuel because it uses biomass sources such as lignocellulosic agricultural and municipal waste which are non-food-based. Anaerobic digesters were originally designed for sewage sludge and manure, but units nowadays operate with two or more types of feedstocks, e.g., dairy manure mixed with grass and corn (found on the land) which can significantly increase gas production (Ma et al., 2017) as can the addition of kitchen grease (fats and oils) collected from restaurants or household waste. Animal fat and abattoir waste can also be used.
Microbial anaerobic digestion produces methane and carbon dioxide that can be purified into biosynthetic natural gas. This can then be pressurised into compressed natural gas (CNG) or liquefied natural gas (LNG) for use in vehicles or injected into the pipeline network. In general terms, methanogenesis is a slow process, requiring a retention time of 14–20 days in an anaerobic digester. The process is sensitive to both high and low pH levels, with the optimum being between pH 6.5 and pH 8.0, as well as to many other inhibitors, especially oxygen. Substrates must have a high carbon to nitrogen (C:N) ratio, which usually results in N-rich feedstock having to be mixed with substrates high in C. Conversion yields can be up to 75% (usually stays between 60% and 70%) of which 50%–75% is CH4, 25%–45% CO2, 2%–8% water vapour and traces of H2S, H2, N2, NH3, and O2. This biogas mixture will require reforming to remove particularly H2S and siloxanes, unless it is being used for heat, following combustion. Reformed gas can then be converted to electricity using steam generation (40%) or methane fuel cells (45%). The effluent from anaerobic digestion still contains high COD and therefore requires further treatment and the process requires energy input for pumping water and gas compression. According to Bhatt et al. (2020), producing biomethane from carboxylate utilisation can be equal to or greater than biogas yield, whilst this is comparable with that of chemical plants, making this economically viable and environmentally friendly as it is a waste treatment process.
At present (2023) the price of biomethane can actually be 30% lower than the current natural gas pricing. Biomethane can be produced starting from €55/MWh, whereas natural gas costs are around €80/MWh, without considering CO2 prices (European Biogas Association, 2023). As a renewable gas this will likely remain cheaper than natural gas in the short- and long-term. Methane is a very potent greenhouse gas, more than 80 times the warming power of carbon dioxide over the first 20 years after it reaches the atmosphere, so methane emissions (via fossil fuel oil and gas companies) are problematic in a world trying to be carbon neutral. The problem of methane emissions is not restricted to the fossil fuel industries and some research has looked into the characterisation and assessment of GHG emissions from typical operational biomethane facilities (Adams and McManus, 2019). This has revealed that there is a wide degree of variability in potential emission sources throughout the supply chain and there are several reasons for this. These include anything from weather, geological conditions, all the way to farming practice and experimental/measurement error. Biomethane emissions occur from crops, fertiliser production and application, the latter resulting in N2O emission, crop yield, methane leakage, electricity use, and diesel use. It is therefore important for biomethane to be utilised locally, where it is produced (just like hydrogen) to avoid high complex systems of high maintenance thereby rendering biomethane a viable, low carbon fuel.
Hydrogen
The majority of hydrogen production-as much as 95% - is still predominantly produced from sources derived from fossil-based fuels, although there is a wider range of green sources that it could be produced from [Ferraren-De Cagalitan and Abundo, 2021). A large part of the industrial hydrogen is obtained from steam-methane reforming (SMR), while oil and coal gasification (CG) follow closely behind. The production costs are between 2 and 3 $/kg. These methods of producing hydrogen also generate greenhouse gases, which are the main drivers of climate change. Alternative methods of producing hydrogen arise from biological processes—hence the name “biohydrogen”—but as with any new technology, this is currently a more expensive process, costing between 3.7 and 7.02 $/kg for photofermentation and MEC respectfully (Ferraren-De Cagalitan and Abundo, 2021).
Biohydrogen production
Sustainable, carbon-neutral hydrogen fuel cells are only possible if they are supplied with renewable hydrogen (Taibi et al., 2018). As with algal biofuel, hydrogen production rates should be economically comparable with other sources of hydrogen. There is a number of different ways of producing biohydrogen, including dark- and photo-fermentation, direct and indirect biophotolysis and via microbial electrolysis cells (MECs) and microbial electrosynthesis cells (MES) (Vasiliadou et al., 2018). The majority of the relevant literature is on bio-hydrogen from MECs. This is a good way of recovering nutrients from waste streams in the cathode chamber, by applying an external potential (using a power source) to recombine electrons and protons, coming from the anode, to H2 with the help of a catalyst. In some cases, phototrophic bacteria are used, which generate true bio-hydrogen when switching from the Krebs cycle to the Calvin cycle; this is also a neat way of nitrogen removal (Vasiliadou et al., 2018). An electrode potential still needs to be applied in order to help accelerate the otherwise sluggish reactions (by comparison).
In photo-fermentation, strains of photosynthetic purple non-sulphur bacteria are employed to convert added organic acids (carboxylate anaerobic fermentation) to CO2 and H2 under N-limited nutrient conditions (Sinha and Pandey, 2011; Sağır and Hallenbeck, 2019; Weber and Lipman, 2019). The naturally occurring organisms used include Rhodobacter, Rhodobium, Rhodopseudomonas, and Rhodospirillum strains, which are capable of transforming a whole list of substrates into H2 across a wide range of light conditions (Das et al., 2014). Photofermentation (unlike biophotolysis) does not generate oxygen which inhibits the H2 production. The yield of hydrogen is comparable to that of biophotolysis although this depends on the design of the photo-fermenter, light intensity, types of medium substrates and species of microorganism (Sağır and Hallenbeck, 2019).
Dark fermentation produces hydrogen in the absence of light. It has been known for many years that Escherichia coli and other facultative anaerobes (Alcaligenes, Enterobacter and Citrobacter) and some strictly anaerobic Clostridium species can ferment sugar substrates into short chain fatty acids including formic acid, which in turn is split by the formate hydrogen lyase (FHL) complex to produce hydrogen (Yoshida et al., 2005). It is well established that the highest theoretical yield for hydrogen is 4 mol of H2 per mole of glucose, as shown below.
Green algae and cyanobacteria can utilise light to break up water into its constituent components O2 and H2, via direct or indirect biophotolysis. Direct biophotolysis occurs when green algae such as Chlamydomonas reinhardtii or subspecies of the Synechocystis cyanobacterium, photosynthesise, i.e., when oxygen is also produced (Azwar et al., 2014), which can be explosive. Indirect biophotolysis is possible whereby certain strains of cyanobacteria can be grown through two distinct stages (Huesemann et al., 2010) a first stage of photosynthesis where carbon dioxide and water are converted into organic molecules some of which are used to make new cell material and oxygen which is evolved. A second phase (which is light independent) is then capable of breaking down the organic molecules into hydrogen, carbon dioxide and other soluble metabolites (Weber and Lipman, 2019). The advantage of the indirect method is that the hydrogen is free of oxygen, whereas the disadvantages are, firstly, the low amounts of H2 produced by this method and secondly, the need for more complex (and more expensive) two phase cultivation systems (Ferraren-De Cagalitan and Abundo, 2021).
Microbial carboxylates
Carboxylates is the collective term for short chain fatty acids including acetate, propionate, lactate and butyrate. These are the intended “products” of the system and can be separated and used as feedstocks for other chemical conversions into a wide range of useful products (e.g., plastics and liquid fuels). The advantages of the carboxylate system are that they can cope with a wide range of organic wastes (Agler et al., 2011) as the inputs and the fermentation steps are far more rapid per gram of mixture than methane production. The challenge is in finding a way to select against methanogens and other “slow” pathways in order to maximise hydrolysis and primary fermentation steps. In conventional fermentation this is achieved by: 1) controlling hydrogen gas concentrations in the liquid phase (dissolved hydrogen); by keeping them low there is no methanogenesis. 2) by removing acetate or higher acid products as soon as they are formed (by continuous flow electro-osmosis or other membrane techniques); this removes the primary substrates (acetate) for methane production. Another method for controlling methanogenesis is to introduce oxygen at low partial pressures which inhibits methanogenesis but has little effect on fermentation to acids by facultative anaerobes. The pH control may also be critical since hydrolysis of polymers is less efficient at low pH levels. All the above factors influence the acetate flux of the system.
The polymeric carbohydrates, lipids, proteins and nucleic acids, of animal tissues, plant or microbial cells are broken down into smaller cell-permeable organic molecules by hydrolytic enzymes that are (a) found widespread in microbes and (b) most often secreted as extracellular enzymes into the external environment by the bacteria that are involved. The metabolites from the breakdown of polymeric substrates are used by heterotrophic species in the synthesis of structural polymers (minor usage) and for synthesising NADH and ATP (major usage), obtained either by fermentation (in an anaerobic environment), anaerobic or aerobic respiration, depending on the microbial species and the presence of oxygen or other end-terminal electron acceptors (such as NO3− or SO42−).
All organic feedstocks or wastes can be best described with regard to their principal carbon-energy polymeric components which can be generally classified as polysaccharides, proteins, glycoproteins, lipids, phospholipids and nucleic acids. This implies the presence of a plethora of corresponding hydrolytic depolymerising enzymes, including amylases, pectinases, chitinases, cellulases, proteases (endopeptidases, aminopeptidases and carboxypeptidases), sialidases, glycosidases, pentosidases, lipases, phospholipases, esterases, DNAses and RNAses.
Microbial fuel cells technology
The MFC is a platform technology capable for converting wet biomass directly into electricity. With appropriate redox control, the technology can simultaneously facilitate green chemistry, whilst also treating (cleaning) the waste that is used fuel. In a way, the technology bridges the gap with the aforementioned industrial processes, thereby offering significant value-add. In their simplest form, MFCs contain two electrodes, with an electrochemical bridge/membrane between them. This facilitates the movement of ions that are dragged during charge (e−) transfer from the anode (e− source) to the cathode (e− sink). The standard half equations that characterise the reactions in the two half-cells are:
Based on electron transfer by microorganisms, MFCs can be mediator-based and mediator-less. Based on transfer of protons, or other ions from the anode to the cathode and vice versa, MFCs can be membrane-based and membrane-less. Some MFCs are truly membrane-less and rely on sedimentation and the formation of redox gradients whilst others (termed ‘single chamber MFC’) are not really membrane-less, but they have very thin membrane open-to-air cathodes without a cathodic chamber; the membrane in this case forms part of the cathode. Figure 1 illustrates the MFC principle of operation in a general, non-container-confined manner diagram.
MFC containers can be of cuboid, cylindrical (tubular), “H”-shaped, flat, large, small or micro-scale embodiment and depending on the bacterial species used, oxidising agents (in the form of O2, SO42−, NO3−) can also be used as end-terminal electron acceptors, thereby widening the types of reaction that can take place and therefore the types of by-product that can be generated. MFC technology has a number of major advantages over conventional remediation treatments of pollutants including 1) adaptation to a wide range of pollutants, organic and inorganic 2i) reduced sludging 3) requires zero energy input, unless special conditions require this 4) has not gas treatment requirements 5) can be operated at ambient temperatures, 6) residence time (HRT) is in hours rather than days. It is a fast-growing field, as evidenced by the increasing number of publications globally, especially those focussing on different types of polluted wastewater. A recent review by Mandal and Das (2018) covers 25 different types of wastewater ranging from municipal/domestic wastewater, recalcitrant pharmaceutical industrial effluent, steroidal drug production wastewater and petrochemical industry wastewater, which implies the removal of antibiotics, synthetic dyes, toluene, polycyclic aromatic hydrocarbons and emerging contaminants, amongst many others.
Depending on the type of cathode used, water can be abstracted from wastewater following treatment and can also be synthesised as a result of the oxygen reduction reaction (ORR) at the cathode, which can be a source of clean, not-potable water. When configured as stacks, MFCs can function as domestic electrical generators for powering small devices, for example, small portable electronic devices like mobile phones, small lamps, computers, electronic toys, batteries and supercapacitor charging machines, fridges and self-feeding robots. The higher the steady state power output, the wider the potential applications.
Aqueous suspensions (sludge) or solutions (sugar) cannot be combusted. However, these ubiquitous solvents are still suitable fuels for microbial fuel cells.
Other types of microbial electrochemical technologies: Microbial electrolysis cell
As already mentioned, protons (or other cations) and electrons recombine at the cathode along with an oxidising agent to close the circuit but under the right conditions, protons and electrons associate to form H2 at the cathode half-cell (Hua et al., 2019). The performance of the MEC depends upon the types of microorganisms in the anodic and cathodic chambers, the type of feedstock substrate, the applied voltage and the nature of the electrode materials. The presence of methanogens must be discouraged since they compete for substrate in the anode and consume hydrogen at the cathode. Methanogenesis can be chemically inhibited, although this will increase operational cost and complexity, so more work needs to be done to improve methane suppression whilst increasing the H2 yield. The MEC architecture and the cost of materials also determine the economic success and operation of the MECs.
MECs require energy input to generate hydrogen from organic matter. Ideally the electrical power can be supplied by a renewable source such as an MFC or solar panels. Electroactive microorganisms in the anode consume an organic energy source and release electrons and protons. This creates a potential of up to 0.3 V which is used to generate electricity in a conventional MFC. In contrast with an MFC, an additional voltage from an outside source is supplied to a working MEC. The combined voltage (providing the cathodic catalysts are suitable), is sufficient to reduce protons (H+) and therefore create H2. The total electrical power that has to be supplied to a MEC is less than for electrolysis of water because a high fraction of the energy required for this reduction is derived from organic fuel via microbial activity at the anode. For hydrogen production, values between 1 and 4 m3H2/m3d have been achieved when 0.8 V was externally supplied (Kadier et al., 2016). The amount of hydrogen production depends on the type of organic substrates employed. Acetic and lactic acid achieve the highest efficiency (82%), whilst the values for glucose or non-pretreated cellulose are significantly lower (63%). It should be noted that the efficiency of hydrogen production by conventional electrolysis of water is only 60%–70%. Therefore, the MEC can produce 144% more energy than they consume in the form of external electrical inputs.
The high costs of the conventional catalytic cathode material (platinum) is between 47% and 85% of the total costs, so this must be overcome (Rozendal et al., 2008). A much cheaper option is to use biocathodes which are made from less expensive materials colonised by microbial species that can use the electrons to produce H2 (Croese et al., 2011). The grouping of species which are naturally photofermentative and can produce H2 (yet do not produce O2) might be an interesting combination. An MEC design that can incorporate both high H2 yields and low costs is very much needed for upscale purposes.
Microbial desalination cells
A microbial desalination cell (MDC) has similarities to MFCs, but the main operation is in situ desalination of salt-water in a third chamber sandwiched between the anode and the cathode. The central chamber interfaces the anode and cathode with a cationic and anionic exchange membrane (or bipolar membranes), respectively. This allows mineral ions in the central chamber (e.g., Na+ and Cl−) to migrate into the cathode or anode respectively, depending on their charge.
Photo-microbial fuel cells
The type of bioelectrochemical system that combines photosynthesis and electricity generation is known as a photo-microbial fuel cell (PMFC) (an alternative term used is bio-photovoltaics). Such devices utilise the microalgae in the cathodic chamber where they grow and utilise the carbon dioxide produced by the microorganisms in the anodic chamber. They also produce oxygen which improves the cathode electrical output. Such PMFC may be useful in the future to provide a significant resolution of both environmental and energy crises at the same time. Both the MDC and the PMFC continue to produce usable amounts of electricity whilst functioning in desalination or recycling of oxygen and carbon dioxide. Neither of these technologies require an external source of electricity.
Hydrogen fuel cells
Hydrogen fuel cells carry a high potential for the future energy needs of our civilisation. As already mentioned, if hydrogen comes from renewables, of which there are abundant resources, then it can be a truly clean source of energy. However, the main challenge of the technology is the flammability of H2 when mixed in either air (4%–74%) or pure O2 (4%–94%), under atmospheric pressure; hydrogen will also escape from most containment vessels resulting in leaks (Rhodes, 2016). The above make storage, containment, and transportation of hydrogen, challenging which can be overcome if H2 is used as it is being produced. High cost of materials and production, as it is common with other technologies, are still to be addressed. Hydrogen is an important topic for national governments and the European Commission in particular, giving emphasis to bio-hydrogen and novel/sustainable ways of production. Under the current climate and war in Ukraine there needs to be a real shift from current practices/systems and this shift needs to be more decentralised to eliminate dependency on mainstream pipelines that can easily be turned off; biohydrogen is one way of achieving this. There are fundamental challenges in storage and containment which in turn implies local and immediate consumption to avoid having to store it. Hydrogen fuel cells are now becoming more commonplace for powering transportation (trains, boats, buses and cars) and they are also being tested on aircraft.
Biomass and the environment
The carbon cycle describes how carbon is distributed around our planet, on land, in water and in the atmosphere and explains the processes that involve carbon exchange between the atmosphere, hydrosphere, biosphere, and lithosphere. As carbon dioxide (and to a lesser extent carbon monoxide, methane and volatile carbon compounds), the carbon helps to regulate the amount of sunlight that enters the planet’s atmosphere. The process by which carbon dioxide is exchanged from atmosphere to the hydrosphere and biosphere is by photosynthesis via plants, macro- and micro-algae or photosynthetic bacteria including cyanobacteria. It is exchanged between the land or water biosphere back to the atmosphere or lithosphere through food chains via decomposition, plant, animal or bacterial respiration, and human activity. Carbon (in the form of organic compounds) can be absorbed by soil from plants via root deposits (rhizodeposition) or by waste products from animals (urine or excrement) or when organisms die and are decomposed. Under the right conditions, carbon can reach the lithosphere via fossilisation, which turns decomposing biomass into peat or coal or petroleum, before re-entering the atmosphere via natural or anthropogenic combustion. Between periods of exchange, carbon is sequestered, or stored either as fossil fuels or as chalk or limestone. The fossil fuel carbon cycle is distinct from the exchange events between biosphere and atmosphere which are caused through respiration, fermentation or natural oxidation. The former has a half-life of many millions of years whilst the latter cycling is more immediate and is sometimes referred to as the immediate carbon cycle. When fossil fuels are exploited, their carbon content (which may have taken millions of years to produce) is released into the atmosphere over a relatively short period of time, mainly just a few hundred years. This overburdens the planets atmosphere. In comparison with fossil fuels, biomass carbon exchange can continue via the immediate carbon cycle. In order for humans to effectively allow Earth to continue the carbon cycle process they must rely more on the immediate carbon cycle and leave fossil fuels alone. Moreover, biomass use and soil conditioning have to be sustainably balanced to take into account the rate of carbon sequestration in—for example, - trees and plants.
Humans and biomass
Plants absorb carbon dioxide by photosynthesis and the amount they absorb is greater than or at least equal to that produced via conventional fuel processing and usage.
The energy content of biofuels is of some interest. With the exception of biohydrogen (which has the highest energy content per kg), all the biofuels are carbon-based. Table 2 presents a list of all the main biofuels. The fossil fuels (petrol, diesel fuel, crude oil, natural gas and coal) are also included for comparison. Because of the significant water content of wet fuels their energy content per kg will be less than that of wet grass, and significantly less, possibly by as much as 90% than the combustible biofuels. The relationship between the water content of sewage sludge and calorific value is shown in Table 3. The higher the water content, the lower the calorific value, per unit of mass.
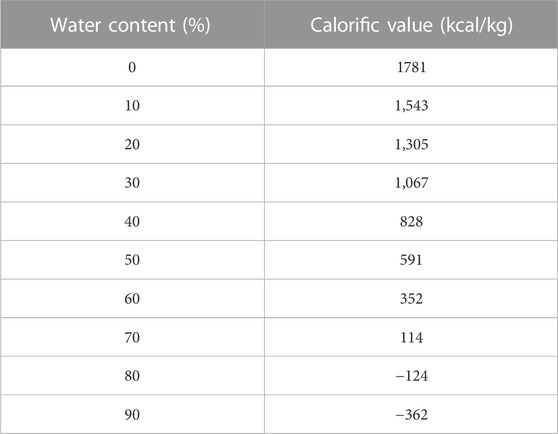
TABLE 3. The relationship of water content and calorific value (Li et al., 2012).
The world’s top ten biofuel crops in order of extent per annum are: switchgrass, wheat, sunflower, cottonseed oil, soy, jatropha, palm oil, sugar cane, canola and corn. The planet as a whole, produces billions of tonnes of wet waste detritus each year from natural estuarine flow, from run off from rotting processes (leaf litter, humus, compost, etc.). Organic biomass is an interesting proposition since globally, it is the most abundant type of fuel. There is more produced “naturally” each year across the planet than the total amounts of fossil fuel abstracted and combusted by humans. The planet produces more than enough biomass to replace fossil fuels entirely. There is no shortage of potential fuel or feedstock that a technology such as MFCs can utilise, including sewage, sludge, farm manure, landfill leachate, black- brown- and white-water run-off, mine water run-off, algal and seaweed waste and river sludge.
The overall biomass composition of the planet (all forms of life) is thought to be ≈ 550 gigatons of carbon (Gt C) (Bar et al., 2018). Plants, the dominant kingdom, are ≈450 Gt C and are mainly terrestrial, whilst animals, at around 2 Gt C, are mainly marine. With regard to microorganisms, these are predominantly located in deep subsurface environments with bacteria (at ≈70 Gt C) outnumbering the archaea (≈7 Gt C) (Bar et al., 2018), and fungi thought to be around 12 Gt C. In terms of annual productivity of biomass (i.e., new biomass per year) then the total annual primary production is thought to be just over 100 billion tonnes C/yr, (Field et al., 1998).
It is expected that by 2050, approximately half of the world’s energy demand/consumption (about 400 EJ/yr) could be met by biomass and that 60% of the world’s electricity market could be supplied by renewables. In energy terms, the production of cereals (−40 EJ), crop residues (−60 EJ), pasture (−75 EJ) and industrial roundwood (−20 EJ) was substantially less than the extraction/production of fossil fuels: gas, (−70 EJ), coal (−40 EJ) and oil (−170 EJ), (IEA, 2021). If wet organic matter (sludges) were regarded as a biofuel it would even be possible to meet all the world’s demand for energy.
The microbes in the MFC (the anodic biofilms) have high affinity metabolic systems for the transport and uptake of nutrient substrates. For example, Escherichia coli (considered to be a typical heterotrophic fermentative species) has a reported ks value of 5.4 μM for acetate (Gimenez et al., 2003) and 20 μM for the transport of glucose (Hunter and Kornberg, 1979). For the anodophilic Geobacter sulferreducens, the Km value for acetate has been reported to range from 2.6 to 0.42 mM acetate (Korth et al., 2020). The ks value gives the concentration of substrate that can produce half the maximum rate of substrate uptake. It should be noted that microbial cells (and therefore microbial fuel cells) continue to take up substrates at even lower concentrations than indicated by the ks value, albeit at low transport rates. The high affinity transport systems for microbes ensure that MFC and related technology (MDC and PMFC) can efficiently clean up waste streams in addition to producing electricity and can manage to produce electricity from very diluted feedstocks (e.g., estuarine river water). Table 4 below summarises the advantages and disadvantages, as well as the key biological mechanisms at play for the different types of biofuel and biotechnology included in this review. The purpose of this summary is to enable readers to more easily see how biology-based solutions compare and how well (or not) they can serve a particular purpose or address a specific problem. As can be seen, BESs show important advantages over existing, much more mature technologies but are still lagging in terms of commercialisation. Although generally, this is not unusual for nascent technologies, human factors i.e., politics, personal preferences/agenda, lobbying, seem to be affecting progress more than other technologies by comparison; this is currently an unexplored research area.
The International Renewable Energy Agency (Irena, 2022) have described the need for a growth in production and use of modern bioenergy in order for humanity to make the critical changes required for ensuring global energy transition from the current situation (82% use of fossil fuels; 18% renewables) with low, to net zero carbon emission scenarios. Bioenergy currently contributes the largest share (two-thirds) of renewables utilisation worldwide, when including the traditional use of biomass. To meet the “1.5°C climate goal scenario,” bioenergy production would need to increase significantly by 2050. Achieving this goal may be challenging without the deployment of sustainable biomass for different purposes since the current deployment of bioenergy remains well below what is needed to achieve the energy transition. This is where collective and interconnected approaches that may include the use of MFCs, can really make a difference, since if appropriately engineered, the outcome will always be greater than the sum of the individual components—a principle well proven in our natural ecosystems.
The vast majority of people on the planet still rely on the traditional (yet inefficient) use of biomass for cooking and heating, affecting health and gender inequality, while leading to deforestation in many areas of the world and adding to climate change. Bioenergy is a multidisciplinary complex area, involving a wide range of stakeholders and issues; more than most other forms of renewable energy. Bioenergy interacts with many sectors, including agriculture, forestry, water industry, waste management and environmental protection. It can have positive impacts if the supply chain is managed well, or potentially negative impacts if the supply chain is poorly managed or indeed bioenergy is abused. The potential sustainability risks of the bioenergy supply chain and its deployment are linked to land use, air pollution, water and soil quality, biodiversity, competition with food supply, and effects on indigenous communities and smallholders.
This is by no means a solved problem, and there is still a lot of work to be done. Typically, over 50% of biomass is likely to be water which has to be extracted to make biofuels. Some estimates suggest that transporting biomass over distances of 100 miles or so from the processing plant is not economically efficient. In addition to carbon dioxide, burning biomass releases nitrogen oxides, carbon monoxide, particulates, and other pollutants. Unless there is use of special technology to capture and recycle the pollutants, burning biomass can create smog and atmospheric pollution as bad as, or even exceeding that of fossil fuels. Biotransformation of biomass however does not produce the same harmful by-products; this is where smart solutions exploiting microbial transforming power can be extremely valuable.
Conclusion
Despite the high water content of sewage sludge, its energy content is comparable with many types of fuel (ranges from 8 to 21 MJ/kg, depending on water content and origin) (Singh et al., 2020). With appropriate treatment using MFCs, municipal sewage sludge can be utilised efficiently and the sludge can be considered as a source of energy even if highly diluted. For high-demand systems the greater the dilution of the sludge the higher the number of MFC stacks required for full utilisation. The finding that small MFCs are more power dense than large volume systems is important and in line with natural ecosystems (for example, electric eel or mammalian blood circulation). A large stack can be built from a few large volume MFCs, or it can be built from a much larger number of small-scale MFCs. Empirical research suggests that the latter strategy will be more successful, especially when considering essential voltage increases and energy density at the small scale, which is in line with allometric scaling in natural systems but also with the approaches adopted for other technologies such as photovoltaics. MFCs work with live microorganisms, which have their own circadian rhythm. This is an important trait of the technology, yet it is often wrongly compared with other forms of electricity sources, such as chemical fuel cells or batteries that are governed by fast-rate chemical reactions, which in turn implies high instantaneous power output for a finite/short period of time. This is one important reason why microbial electrochemical technologies cannot be directly compared to chemical or other abiotic electrochemical systems, as they operate on different levels: high energy for microbial electrochemical technologies vs. high power for chemical or electrochemical systems. When technologies such as MFC stacks are employed, then this allows for vertical stacking, much like vertical farming, thereby getting around the problem of using a large footprint. Because MFCs can “treat” organic sludge and lower the BOD as well as generate electricity this serves a justifiable purpose to start developing large scale stacks. To fully extract the energy content of the waste-stock fuel the MFC or PMFC have to be stacked using cascades of 7–9 individual units (empirical finding) in order to fully reduce the BOD to an acceptable level. In a cascade, the fuel is sequentially treated and the treatment time to allow for full hydrolysis and digestion of microbial biomass can be competitively short, with enrichment of the most efficient microflora. The hydraulic retention time within the cascades can be appropriately tuned to suit the composition of the incoming feedstock, rendering the MFC/BES technology suitable for both highly concentrated and dilute waste streams. For very dilute feedstocks the uptake and utilisation of substrates depends upon the affinity values (ks) of the microbial species the macromolecular structure, the size of molecules and the concentration of the substrate being digested. The supply rate of substrate is also important, and this can be actively controlled by changing the speed of the feedstock pump, or passively by introducing flow-restrictors or even dynamically by changing the volume of the anodic chambers in situ, using soft or compliant materials. With the exception of hydrogen, nearly all fuels used on the planet are carbon-based. In the near future fossil fuels will be phased out leaving only the biofuels made from primary plants and microbes. Because these are part of the immediate carbon cycle, if used responsibly they will not contribute to planetary overload of methane or carbon dioxide, allowing the climate to rebalance in time, hopefully before all lifeforms become extinct.
Author contributions
All authors listed have made a substantial, direct, and intellectual contribution to the work and approved it for publication.
Acknowledgments
II is a Bill & Melinda Gates Foundation grantee (INV-042655). Some of the reported findings have been produced as part of the EU COST Action PHOENIX, CA-19123.
Conflict of interest
The authors declare that the research was conducted in the absence of any commercial or financial relationships that could be construed as a potential conflict of interest.
Publisher’s note
All claims expressed in this article are solely those of the authors and do not necessarily represent those of their affiliated organizations, or those of the publisher, the editors and the reviewers. Any product that may be evaluated in this article, or claim that may be made by its manufacturer, is not guaranteed or endorsed by the publisher.
References
Adams, P. W. R., and McManus, M. C. (2019). Characterisation and variability of greenhouse gas emissions from biomethane production via anaerobic digestion of maize. J. Clean. Prod. 218, 529–542. doi:10.1016/j.jclepro.2018.12.232
Agler, M. T., Wrenn, B. A., Zinder, S. H., and Angenent, L. T. (2011). Waste to bioproduct conversion with undefined mixed cultures: The carboxylate platform. Trends Biotechnol. 29, 70–78. doi:10.1016/j.tibtech.2010.11.006
Alternative Fuels Data Center Fuel Properties Comparison (2022). US department of energy, energy efficiency and renewable energy, Energy's vehicle technologies Office.
Ananthi, V., Raja, R., Carvalho, I. S., Brindhadevi, K., Pugazhendhi, A., and Arun, A. (2021). A realistic scenario on microalgae based biodiesel production: Third generation biofuel. Fuel 284, 118965. doi:10.1016/j.fuel.2020.118965
Azevedo, S. G., Sequeira, T., Santos, M., and Mendes, L. (2019). Biomass-related sustainability: A review of the literature and interpretive structural modeling. Energy 171, 1107–1125. doi:10.1016/j.energy.2019.01.068
Azwar, M. Y., Hussain, M. A., and Abdul-Wahab, A. K. (2014). Development of biohydrogen production by photobiological, fermentation and electrochemical processes: A review. Renew. Sustain. Energy Rev. 31, 158–173. doi:10.1016/j.rser.2013.11.022
Bar, Y. M., Phillips, R., and Milo, R. (2018). The biomass distribution on. Earth. Proc. Natl. Acad. Sci. 115, 6506–6511.
Bhatt, A. H., Ren, Z., and Tao, L. (2020). Value proposition of untapped wet wastes: Carboxylic acid production through anaerobic digestion. iScience 23, 101221. doi:10.1016/j.isci.2020.101221
Black, R., Cullen, K., Fay, B., Hale, T., Lang, J., Mahmood, S., et al. (2021). “Taking stock: A global assessment of net zero targets,” in Energy & climate intelligence unit and oxford net zero.
Croese, E., Pereira, M. A., Euverink, G. J. W., Stams, A. J. M., and Geelhoed, J. S. (2011). Analysis of the microbial community of the biocathode of a hydrogen-producing microbial electrolysis cell. Appl. Microbiol. Biotechnol. 92, 1083–1093. doi:10.1007/s00253-011-3583-x
Das, D., Khanna, N., and Dasgupta, C. N. (2014). “Biohydrogen production,” in CRC press taylor & francis group (2014). First Edition, 55–79.
Delong, J. P., Okie, J. G., Moses, M. E., Sibly, R. M., and Brown, J. H. (2010). Shifts in metabolic scaling, production, and efficiency across major evolutionary transitions of life. Proc. Natl. Acad. Sci. 107, 12941–12945. doi:10.1073/pnas.1007783107
Engineering ToolBox (2001). Engineering ToolBox. [online] Available at: https://www.engineeringtoolbox.com (Accessed October 25, 2022).
European Biogas Association (2023). A way out of the EU gas price crisis with biomethane. Available at: https://www.europeanbiogas.eu/(Accessed January 23, 2023).
Ferraren-De Cagalitan, D. D. T., and Abundo, M. L. S. (2021). A review of biohydrogen production technology for application towards hydrogen fuel cells. Renew. Sustain. Energy Rev. 151, 111413. doi:10.1016/j.rser.2021.111413
Field, C. B., Behrenfeld, M. J., Randerson, J. T., and Falkowski, P. (1998). Primary production of the biosphere: Integrating terrestrial and oceanic components. Science 281, 237–240. doi:10.1126/science.281.5374.237
Gimenez, R., Nunez, M. F., Badia, J., Aguilar, J., and Baldoma, L. (2003). The Gene yjcG, co-transcribed with the gene acs, encodes an acetate permease in Escherichia coli. J. Bacteriol. 185, 6448–6455. doi:10.1128/jb.185.21.6448-6455.2003
Greenman, J., and Ieropoulos, I. A. (2017). Allometric scaling of microbial fuel cells and stacks: The lifeform case for scale-up. J. Power Sources 356, 365–370. doi:10.1016/j.jpowsour.2017.04.033
Hazrat, M. A., Rasul, M. G., Khan, M. M. K., Ashwath, N., Fattah, I. M. R., Ong, H. C., et al. (2022). Biodiesel production from transesterification of Australian Brassica napus L. Oil: Optimisation and reaction kinetic model development. Environ. Dev. Sustain. doi:10.1007/s10668-022-02506-0
Hoang, A. T., Huang, Z. H., Nižetić, S., Pandey, A., Nguyen, X. P., Luque, R., et al. (2022). Characteristics of hydrogen production from steam gasification of plant-originated lignocellulosic biomass and its prospects in Vietnam. Int. J. Hydrogen Energy 47, 4394–4425. doi:10.1016/j.ijhydene.2021.11.091
Hua, T., Li, S., Li, F., Zhou, Q., and Ondon, B. S. (2019). Microbial electrolysis cell as an emerging versatile technology: A review on its potential application, advance and challenge. J. Chem. Technol. Biotechnol. 94, 1697–1711. doi:10.1002/jctb.5898
Huesemann, M. H., Hausmann, T. S., Carter, B. M., Gerschler, J. J., and Benemann, J. R. (2010). Hydrogen generation through indirect biophotolysis in batch cultures of the nonheterocystous nitrogen-fixing cyanobacterium Plectonema boryanum. Appl. Biochem. Biotechnol. 162, 208–220. doi:10.1007/s12010-009-8741-6
Hunter, I. S., and Kornberg, H. L. (1979). Glucose transport of Escherichia coli growing in glucose-limited continuous culture. Biochem. J. 178, 97–101. doi:10.1042/bj1780097
Huxley, J. S., and Teissier, G. (1936). Terminology of relative growth. Nature 137, 780–781. doi:10.1038/137780b0
IEA (2021). Key world energy statistics 2021. Paris: IEA. License: CC BY 4.0 Available at: https://www.iea.org/reports/key-world-energy-statistics-2021.
IRENA (2022). Bioenergy for the energy transition: Ensuring sustainability and overcoming barriers. Abu Dhabi: International Renewable Energy Agency. ISBN: 978-92-9260-451-6. Available at: https://www.irena.org//media/Files/IRENA/Agency/Publication/2022/Aug/IRENA_Bioenergy_for_the_transition_2022.pdf?rev=875a997481f04168b17499f1e5dc1473 (Accessed January 19, 2023).
Kadier, A., Kalil, M. S., Abdeshahian, P., Chandrasekhar, K., Mohamed A,Azman, N. F., et al. (2016). Recent advances and emerging challenges in microbial electrolysis cells (MECs) for microbial production of hydrogen and value-added chemicals. Renew. Sustain. Energy Rev. 61, 501–525. doi:10.1016/j.rser.2016.04.017
Kang, S., Heo, S., and Lee, J. H. (2019). Techno-economic analysis of microalgae-based lipid production: Considering influences of microalgal species. Ind. Eng. Chem. Res. 58, 944–955. doi:10.1021/acs.iecr.8b03999
Karthikeyan, S., Periyasamy, M., and Prathima, A. (2020). Biodiesel from microalgae: Environmental Aspects. Mater. Today Proc. 33, 3664–3667. doi:10.1016/j.matpr.2020.05.779
Korth, B., Kretzschmar, J., Bartz, M., Kuchenbuch, A., and Harnisch, F. (2020). Determining incremental coulombic efficiency and physiological parameters of early stage Geobacter spp. Enrich. Biofilms. PLoS ONE 15 (6), e0234077.
Lam, S. S., Tsang, Y. F., Yek, P. N. Y., Liew, R. K., Osman, M. S., Peng, W., et al. (2019). Co-Processing of oil palm waste and waste oil via microwave Co-torrefaction: A waste reduction approach for producing solid fuel product with improved properties. Process. Saf. Environ. Prot. 128, 30–35. doi:10.1016/j.psep.2019.05.034
Lee, R. A., and Lavoie, J-M. (2013). From first-to third-generation biofuels: Challenges of producing a commodity from a biomass of increasing complexity. Anim. Front. 3, 6–11. doi:10.2527/af.2013-0010
Li, Y., Wang, H., Zhang, J., Wang, J., and Ouyang, L. (2012). The industrial practice of Co-processing sewage sludge in cement kiln. Sci. 16, 628–632. doi:10.1016/j.proenv.2012.10.086
Ma, G., Neibergs, J. S., Harrison, J. H., and Whitefield, E. M. (2017). Nutrient contributions and biogas potential of co-digestion of feedstocks and dairy manure. J. Waste Manag. 64, 88–95. doi:10.1016/j.wasman.2017.03.035
Mandal, S. K., and Das, N. (2018). Application of microbial fuel cells for bioremediation of environmental pollutants: An overview. J. Microbiol. Biotechnol. Food Sci. 7, 437–444. doi:10.15414/jmbfs.2018.7.4.437-444
Mestre, F., Gravel, D., García-Callejas, D., Pinto-Cruz, C., Matias, M. G., and Araújo, M. B. (2022). Disentangling food-web environment relationships: A review with guidelines. Basic Appl. Ecol. 61, 102–115.
Mofijur, M., Siddiki Sk, Y. A., Shuvho Md, B. A., Djavanroodi, F., Fattah I-M, R., Ong, H. C., et al. (2021). Effect of nanocatalysts on the transesterification reaction of first, second and third generation biodiesel sources- A mini-review. Chemosphere 270:128642, doi:10.1016/j.chemosphere.2020.128642
Mubarak, M., Shaija, A., and Suchithra, T. V. (2019). Flocculation: An effective way to harvest microalgae for biodiesel production. J. Environ. Chem. Eng. 7, 103221. doi:10.1016/j.jece.2019.103221
National Renewable Energy Laboratory National renewable energy laboratory. Available at: www.nrel.gov/publications.
Peng, L., Fu, D., Chu, H., Wang, Z., and Qi, H. (2020). Biofuel production from microalgae: A review. Environ. Chem. Lett. 18, 285–297. doi:10.1007/s10311-019-00939-0
Rafa, N., Ahmed, S. F., Badruddin, I. A., Mofijur, M., and Kamangar, S. (2021). Strategies to produce cost-effective third-generation biofuel from microalgae. Front. Energy Res. 9 (749968), 10. doi:10.3389/fenrg.2021.749968
Rahman, K. M. (2020). “Food and high value products from microalgae: Market opportunities and challenges,” in Microalgae biotechnology for food, health and high value products. Editors M. A. Alam, J. L. Xu, and Z. Wang (Singapore: Springer Nature), 3–28.
Rozendal, R. A., Hamelers, H. V. M., Rabaey, K., Keller, J., and Buisman, C. J. N. (2008). Towards practical implementation of bioelectrochemical wastewater treatment. Trends Biotechnol. 26, 450–459. doi:10.1016/j.tibtech.2008.04.008
Sağır, E., and Hallenbeck, P. C. (2019). “Photofermentative hydrogen production,” in Biomass, biofuels, biochemicals: Biohydrogen. Second Edition (Elsevier), 141–157.
Shah, M. M. R., Liang, Y., Cheng, J. J., and Daroch, M. (2016). Astaxanthin-producing green microalga Haematococcus pluvialis: From single cell to high value commercial products. Front. Plant Sci. 7, 531. doi:10.3389/fpls.2016.00531
Singh, V., Phuleria, H. C., and Chandel, M. K. (2020). Estimation of energy recovery potential of sewage sludge in India: Waste to watt approach. J. Clean. Prod. 276, 122538. doi:10.1016/j.jclepro.2020.122538
Sinha, P., and Pandey, A. (2011). An evaluative report and challenges for fermentative biohydrogen production. Int. J. Hydrogen Energy 36, 7460–7478. doi:10.1016/j.ijhydene.2011.03.077
Snell, O. (1892). Die Abhängigkeit des Hirngewichts von dem Körpergewicht und den geistigen Fähigkeiten. Arch. Psychiatr. 23, 436–446. doi:10.1007/bf01843462
Sondhi, S., Kaur, P. S., and Kaur, M. (2020). Techno-economic analysis of bioethanol production from microwave pretreated kitchen waste. SN Appl. Sci. 2, 1558. doi:10.1007/s42452-020-03362-1
Sun, T., Zhang, Y., Zhang, C., Wang, H., Pan, H., Liu, J., et al. (2020). Cyanobacteria-based bio-oxygen pump promoting hypoxia-resistant photodynamic therapy. Front. Bioeng. Biotechnol. 8, 237–311. doi:10.3389/fbioe.2020.00237
Taibi, E., Miranda, R., Vanhoudt, W., Winkel, T., Barth, F., and Lanoix, J. C. (2018). Hydrogen from renewable power: Technology outlook for the energy transition. IRENA, Policy Paper. September. Available at: https://www.h2knowledgecentre.com/content/policypaper1306 (Accessed January 19, 2023).
Um, B-H., and Kim, Y-S. (2009). Review: A chance for korea to advance algal-biodiesel technology, J. Industrial Eng. Chem. 15, 1–7. doi:10.1016/j.jiec.2008.08.002
US DoE (2022). Energy efficiency & renewable energy, alternative fuel price report. Available at: https://afdc.energy.gov/fuels/prices.html (Accessed November 5, 2022).
Vasiliadou, I. A., Berná, A., Manchon, C., Melero, J. A., Martinez, F., Esteve-Nuñez, A., et al. (2018). Biological and bioelectrochemical systems for hydrogen production and carbon fixation using purple phototrophic bacteria. Front. Energy Res. 6, 107. doi:10.3389/fenrg.2018.00107
Weber, A. Z., and Lipman, T. E. (2019). Fuel cells and hydrogen production. New York, NY: Springer, 1–8.Fuel cells and hydrogen production: Introduction
World Bioenergy Association (2019). Global bioenergy statistics. Available at: http://www.worldbioenergy.org/uploads/191129%20WBA%20GBS%202019_HQ.pdf (Accessed November 04, 2022).
Yin, Z., Zhu, L., Li, S., Hu, T., Chu, R., Mo, F., et al. (2020). A comprehensive review on cultivation and harvesting of microalgae for biodiesel production: Environmental pollution control and future directions. Technol 301, 122804. doi:10.1016/j.biortech.2020.122804
Keywords: microbial fuel cells, biomass, bioenergy, clean water, environmentally friendly
Citation: Ieropoulos I and Greenman J (2023) The future role of MFCs in biomass energy. Front. Energy Res. 11:1108389. doi: 10.3389/fenrg.2023.1108389
Received: 26 November 2022; Accepted: 06 March 2023;
Published: 22 March 2023.
Edited by:
Uwe Schröder, University of Greifswald, GermanyReviewed by:
Sunil A. Patil, Indian Institute of Science Education and Research Mohali, IndiaFeng Zhao, Institute of Urban Environment (CAS), China
Copyright © 2023 Ieropoulos and Greenman. This is an open-access article distributed under the terms of the Creative Commons Attribution License (CC BY). The use, distribution or reproduction in other forums is permitted, provided the original author(s) and the copyright owner(s) are credited and that the original publication in this journal is cited, in accordance with accepted academic practice. No use, distribution or reproduction is permitted which does not comply with these terms.
*Correspondence: Ioannis Ieropoulos, aS5pZXJvcG91bG9zQHNvdG9uLmFjLnVrJiN4MDIwMGE7