- 1Nuclear Research and consultancy Group (NRG), Petten, Netherlands
- 2International Atomic Energy Agency (IAEA), Vienna International Centre, Vienna, Austria
The reactivity worth of many fission product samples was measured in fast spectrum conditions in the STEK facility during the early 1970s. These results were then used to improve and validate nuclear data evaluations for fission products, but in the last 2 decades STEK has not been used: the nuclear data evaluations for fission products in the current versions of libraries like ENDF/B or JEFF have not been tested against STEK results. Here we argue that the STEK data are still valuable, because there is no other data set that can replace them, and the interest in fast spectrum conditions is picking up strongly of late. It should be considered to evaluate whether a benchmark can be defined for the International Reactor Physics Experiments Handbook.
1 Introduction
There is an increasing effort underway to design new nuclear reactors (IAEA 2022). While many initiatives involve designs that are evolutionary in that they are largely based on the designs of the currently operational reactor fleet, it is noticeable that there are serious developments towards fast spectrum reactors. Some of these are developed in the Generation-IV framework, while more recently a strong push towards small modular reactors (SMR) has given rise to new fast spectrum designs (IAEA 2020; OECD 2021).
This leads to a situation where there is a need for experimental data to support such designs. Given that the defining feature of these designs is their fast neutron spectrum, the cross section data in this spectrum warrant special attention. The last period during which there was a similar need was, arguably, the 1970s–1980s, when fast breeder reactors were under design.
In that period the STEK experiment was performed in Petten, the Netherlands. The experiments were performed with a reactor that consisted of a thermal spectrum outer ‘driver’ zone, coupled to a fast spectrum inner zone. In the middle of the inner zone was an experimental facility in which a sample could be moved into and out of the reactor core. In this way the reactivity worth was measured for many samples, among which many fission product samples, in five different spectrum conditions.
The STEK results were used for the development of various nuclear data library versions at the time: JEF-1 (Janssen et al., 1986), JEF-2.2 (Dietze et al., 2001) and JENDL-3.2 (NEA 2001) are examples of this. Since then several newer versions of the same libraries were released, but the use of the STEK experiments during library development was discontinued without other integral data being used instead.
Because of the recent developments in fast reactor designs, we argue that the results of the STEK experiments are still (or again) valuable today, together with other measurement data of the same period such as RRR/SEG (Rossendorf, Germany). In 2003 all available information on the STEK experiments, including more than 25 technical reports, was sent to the OECD/NEA Databank, where it was digitized and archived under the name NEA-1714 IRPhE/STEK (OECD/NEA, 2022). In the current way of working in the international reactor physics field, the measurements should be evaluated in the framework of the International Reactor Physics Experiments Handbook. Once that is done the experimental results would become more easily available to whoever needs them to support their fast spectrum reactor design. Such benchmark definitions of past measurement campaigns would allow progress in the field before new integral experiments are performed.
In this paper a high level description is given of the STEK facility (Section 2), together with an overview of the measurements results that were obtained (Section 3). The role that these results have played in the past in nuclear data developments is reviewed (Section 4), to clarify the potential of this set of measurements to improve the quality of future nuclear data for fission products, especially for fast spectrum conditions.
2 The STEK facility
The STEK facility was built around 1970 in the framework of the cooperation on fast breeder reactor development between (then Western) Germany and the Benelux countries (Belgium, Netherlands and Luxemburg). The main objective was to perform integral measurements of fission product cross sections. These cross sections were determined from central reactivity worth measurements, using an oscillator technique. The zero power reactor consisted of a thermal spectrum driver zone on the outside and a fast spectrum inner center of the core.
The central fast zone, in a cylindrical inner tank of 1,060 mm diameter and 1,275 mm height, had fuel elements on a rectangular grid surrounded by rows of lead and graphite assemblies, acting as reflector and buffer zones. The fast fuel assemblies were stacks of high enriched (90 wt%) uranium and graphite platelets, held together by aluminum boxes. The uranium platelets had areas of 50.63 × 50.63 mm2 or 43.0 × 43.0 mm2, thickness of 1.4 mm or 0.7 mm, and a 15 μm nickel coating.
Depending on the number and type of the platelets, different levels of spectrum hardness could be realized by changing the ratio of carbon atoms to 235U atoms (C/U). The fuel elements with high C/U ratio contained less fissile material than the ones with low C/U, and therefore, for the higher C/U cases, more fuel elements were needed to achieve criticality. In total five different cores were built, with C/U ratios ranging from C/U = 11 for the fastest spectrum to C/U = 72 for the least fast spectrum. The number of fuel elements varied from 49 for the fastest spectrum core to 129 for the core with the least fast spectrum. The number of graphite and lead elements varied accordingly, from 88 lead elements and 104 graphite elements for the fastest spectrum core to 56 and 56 elements for the core with the least fast spectrum.
Surrounding the inner, fast spectrum zone was an annular tank, made of aluminum, with an outer diameter of 2,140 mm and a height of 1,360 mm. The ring is subdivided in four equal sectors, each comprising of about 80° of the annulus. These four sectors were separated by four graphite blocks, through which beam tubes gave access to the central part. In each of the four sectors 10 plate type fuel elements were placed, moderated by water. These elements, with a cross section of 77 × 150 mm2, consisted of boxes in which 5 to 16 fuel plates were placed. The plates were made of high enriched (90 wt%) UAl4 with Al cladding. The number of plates per elements depended on the composition of the fast, central zone. The radial reflector outside the annular tank consisted of water.
In the center of the fast zone a normal fuel element could be replaced by a square guide tube, in which a special oscillator element was placed, connected to a driving system to move the element with a velocity in the range of 1–10 m/min. The effective length of the guide tube was 1,200 mm, penetrating the core from top to bottom. In this guide tube the 2,600 mm long oscillator element could be moved up and down. The oscillator element was subdivided into 53 compartments by stainless steel-304 partitions. Most of the compartments were filled with fast fuel material, except for compartments 15 and 41, which were alternately in the center of the core during oscillation measurements. One of the two compartments contained a sample, whereas the other compartment was empty. In order to reduce the (small) effect of the reactor and the oscillation element not being fully symmetric in the axial direction, two measurements were performed for each sample: one with the sample in compartment 15, and the other measurement with the sample in compartment 41. Also, measurements for a dummy reference sample were performed, with the reference sample having the same composition and weight as the packing of the original sample. The distance between compartments 15 and 41 was 1,248 mm.
A photo of STEK is shown in Figure 1. The annular tank with the thermal spectrum driver zone can be seen clearly, as well as the cylindrical, fast spectrum inner zone containing a rectangular grid with fuel elements. Also visible, in the center of the facility, sticking out at the top, is the rectangular guide tube for the oscillator element. More detailed (schematic) core maps of the inner core region of the STEK-500 and STEK-4000 configurations are shown in Figure 2.
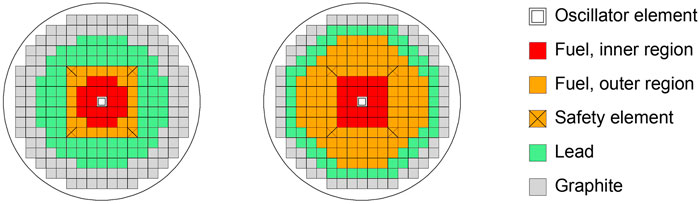
FIGURE 2. A schematic core map of the inner core regions of STEK-500 (left) and STEK-4000 (right). The outside “driver” fuel zone with a thermal spectrum is not visible in this picture, beyond the outer ring.
The neutron spectrum in the center of the five different core configurations was recently calculated using MCNP models made by Da Cruz et al. (2013). The results are shown in Figure 3. The fastest spectrum, for the configuration labeled STEK-500, the neutron flux starts to drop precipitously below approximately 10 keV, with virtually no flux left below 0.1 keV. For the most thermal spectrum, STEK-4000, the flux drops significantly only below 1 keV, and the spectrum extends down to thermal energies, around 0.3 eV. It is this wide range of neutron spectra that makes the STEK measurements almost unique.
3 Measurement data
3.1 Measurements and analysis
Fission reaction rate measurements were performed in all five core configurations, in a location very close to the center of the core. These reactions were measured with fission chambers for the nuclides 233U, 238U, 232Th, 237Np, 239Pu, 240Pu, and 242Pu. The results were reported as reaction rate ratios relative to the 235U fission reaction rate. For 235U and 238U also reaction rate traverses were measured, both in the axial and radial directions. These measurements enabled a characterization of the neutron spectrum, as well as a determination of the 235U fission rate as part of the normalization of the reactivity worths to be measured.
For the reactivity worth measurements, the neutron flux level was measured by one or more compensated ionization chambers located in the region around the thermal core, either in the water reflector or in one of the beam tubes. Another chamber, used for verification of the validity of point reactor kinetics, was located in the fast zone. The output voltages were converted to pulse trains, which were counted in a buffer register in time intervals adjustable from 0.25 to 4 s. The total number of pulses per time interval and the position of the measuring element were stored.
The inverse kinetic method was used to calculate the reactivity for each time interval. The reactivity was calculated on-line as a function of time by solving the inverse kinetics equations, based on the measured neutron flux time history. The movement of the oscillator element was analyzed in different phases of the movement. After a number of sample oscillations the program performed a final calculation to determine the average reactivity difference, including statistical uncertainty, between the positions “IN” (the sample in the center of the core) and “OUT” (the sample completely out of the core). A correction was performed for the linear component of a possible reactor drift.
Normalization of the measured reactivity worths was done by measuring reactivity effect of ‘standard materials’. These materials were selected based on the requirements that their cross sections must be known accurately, and the energy dependence of their cross sections should be relatively similar to the energy dependence of the cross sections of the samples. The materials used were 235U, boron, and 252Cf.
The measurement errors were reported to be, in general, composed of a systematic error ≤2.1% and a statistical error ≤2.0%.
3.2 Reported results for reactivity worth.
Results were reported for a large collection of samples, with masses typically in the order of 1–10 g of the element of interest. The majority of the samples contained a fission product, either as a natural element or in enriched form. Many samples were highly hygroscopic and had to be dried and packed carefully since any water contamination would have given a large reactivity disturbance in the STEK cores.
The fission product samples in the form of natural elements were Zr, Nb, Mo, Rh, Pd, I, Cs, La, Ce, Pr, Nd, Eu, and Tb.
The fission product samples in enriched form were samples with high content of 90Zr, 91Zr, 92Zr, 96Zr, 92Mo, 94Mo, 95Mo, 96Mo, 97Mo, 98Mo, 100Mo, 99Tc, 101Ru, 102Ru, 104Ru, 104Pd, 105Pd, 106Pd, 107Pd, 108Pd, 110Pd, 109Ag, 111Cd, 128Te, 130Te, 129I, 131Xe, 135Cs, 142Ce, 142Nd, 143Nd, 144Nd, 145Nd, 146Nd, 148Nd, 150Nd, 147P.m., 147Sm, 148Sm, 149Sm, 150Sm, 151Sm, 152Sm, 154Sm, 153Eu, 156Gd, and 157Gd.
A special sample was zirconium with 20% 93Zr, produced by irradiation in the High Flux Reactor (also in Petten). Various other samples were measured, sometimes to correct for the chemical composition of the samples with fission products. A typical example was cesium, which was available as CsCl2. A correction for the reactivity effect due to chlorine could be obtained via separate measurements of the effects of Pb and PbCl2.
For example, various samples of 103Rh in the mass range of 1–10 g were measured (for reference: the amount of 103Rh in spent fuel is typically of the order of 0.5 g per kg of fuel). The reactivity effect of a sample of 1.1 g was reported to decrease from −1.46 pcm/g in STEK-4000 (the least hard spectrum) to −0.30 pcm/g in STEK-500 (the hardest spectrum). These values were reported as a fraction relative to a reference sample, and the value for the reference sample was given in units of 10–4 βeff/g. The values were translated here to units of pcm/g for convenience of reading, but the uncertainty of the converted values has not been evaluated and is therefore not stated here.
4 Use in nuclear data evaluation
The STEK measurements are particularly important for validation, and improvement, of fission product nuclear data evaluations in the fast range. The main difference with nuclear reactions in thermal spectra is that the peak of the spectra of Figure 3, say between 100 keV and 2 MeV is in the region where inelastic neutron scattering plays an important role, as it is a competing channel for neutron capture. Hence, via inelastic scattering the neutrons will lose energy in a different way than they would do in a thermal spectrum. The importance of this effect was recognized by the nuclear data community, and the Working Party on Evaluation Coordination (WPEC) launched a special subgroup (SG10) for this (NEA 2001).
Validation using the STEK data showed the calculated sample reactivity worths are in reasonable to good agreement (20%) with those measured at STEK for strong absorbers but that for weak absorbers some anomalous results were obtained. It was considered that these problems probably came from poor inelastic scattering data. After this validation exercise, OECD/NEA launched two other international nuclear data efforts: WPEC SG17 (NEA 1998), which assessed the status of pseudo-fission products cross sections for fast spectra, and SG-21 (NEA 2004), on a comparison between, and SG-23 (NEA 2009) for the evaluation of, neutron data files for fission products. The latter data files have found their way in several international nuclear data libraries like ENDF/B, JEFF and JENDL, but a proper validation with integral data in the fast neutron range has not been carried out since then, to our knowledge.
Moreover, in the past 2 decades the evaluation methods for fission products have been improved considerably, leading to better founded nuclear data libraries and a more routinely use of powerful optical models for scattering and non-approximate coupled-channels methods for inelastic reactions, mainly thanks to a significant increase in computer power and automation of evaluation software. Current nuclear data evaluators are confident that improved nuclear data files, and this time with covariance information, can be produced to answer new requests from fast reactor technology. Nuclear data evaluations and the associated covariance adjustment could repeated with a perturbation method (Dragt et al., 1977) or with modern Total Monte Carlo techniques. An issue is which type of simulation will be used given the small reactivity worth per sample, which makes validation by a Monte Carlo code tedious.
The definition of a high-quality integral benchmark for fast neutrons would surely motivate the nuclear data file projects that reside under WPEC to invest effort into new fission product evaluations. The construction of the integral benchmark and new nuclear data libraries could progress in parallel in the coming years.
5 Discussion
The STEK measurement results provide an interesting opportunity for checking nuclear data evaluations of fission products. Yet to our knowledge this has not been done for evaluations in the current versions of libraries like ENDF/B or JEFF, most probably because the measurement results are not in a form that makes it easy to perform such a check. Therefore, if there is sufficient interest in fission product worths in fast spectrum conditions, it should be evaluated whether the STEK measurements can be converted into a benchmark for the International Reactor Physics Experiments (IRPhE) Handbook.
An important part of such an evaluation is the availability of information on the measurements, and the accuracy of the results. In the case of STEK, it should also be discussed how the complex geometry can be dealt with, because the geometrical complexity of the facility does not lend itself easily to deterministic calculations. Yet on the other hand, many the measured fission product worths were of the order of a couple of pcm (‘pour-cent-mille’, 10–5), which is very hard to calculate with Monte Carlo techniques.
Data availability statement
Publicly available datasets were analyzed in this study. This data can be found here: OECD/NEA Data Bank, www.oecd-nea.org/tools/abstract/detail/nea-1714.
Author contributions
All authors listed have made a substantial, direct, and intellectual contribution to the work and approved it for publication.
Conflict of interest
SM is currently employed by NRG and AK was employed by NRG until 2015.
Publisher’s note
All claims expressed in this article are solely those of the authors and do not necessarily represent those of their affiliated organizations, or those of the publisher, the editors and the reviewers. Any product that may be evaluated in this article, or claim that may be made by its manufacturer, is not guaranteed or endorsed by the publisher.
References
Da Cruz, D. F., Sciolla, C. M., and Rochman, D. A. (2013). STEK experiment – opportunity for validation of fission products nuclear data. Trans. ANS 108, 701–703.
Dietze, K., Ishikawa, M., and Rimpault, G. (2001). Integral test of neutron data and comparison of codes by Re-analysis of the SEG and STEK experiments. JEFF/DOC-861.
Dragt, J. B., Dekker, J. W. M., Gruppelaar, H., and Janssen, A. J. (1977). Methods of adjustment and error evaluation of neutron capture cross sections; application to fission product nuclides. Nucl. Sci. Eng. 62, 117–129. doi:10.13182/nse77-3
IAEA (2022). Advanced reactors information system (ARIS). Available at: https://aris.iaea.org/(Accessed October 6, 2022).
IAEA (2020). Advances in small modular reactor technology developments - a supplement to: IAEA advanced reactors information system. (ARIS) - 2020 Edition, IAEA Report.
Janssen, A. J., Gruppelaar, H., Heijboer, R. J., Karouby-Cohen, N., Martin-Deidier, L., Rimpault, G., et al. (1986). Integral-data test of JEF-1 fission product cross sections. Jef. Rep. 5. ECN-176.
NEA (2004). Assessment of neutron cross sections for the bulk of fission products, OECD/NEA Report NEA/WPEC-21.
NEA (2001). Evaluation method of inelastic scattering cross-sections for weakly absorbing fission-product nuclides, OECD/NEA Report NEA/WPEC-10.
NEA (1998). Status of pseudo-fission-product cross-sections for fast reactors, OECD/NEA Report NEA/WPEC-17.
OECD/NEA (2022). IRPhE/STEK, reactor physics experiments from fast-thermal coupled facility. Available at: www.oecd-nea.org/tools/abstract/detail/nea-1714 (Accessed October 6, 2022).
Keywords: fast spectrum, cross section, fission product, benchmark, reactor
Citation: van der Marck S and Koning A (2023) STEK: A potential fast spectrum benchmark for fission product cross sections. Front. Energy Res. 11:1085857. doi: 10.3389/fenrg.2023.1085857
Received: 31 October 2022; Accepted: 03 February 2023;
Published: 14 February 2023.
Edited by:
John Darrell Bess, JFoster & Associates, LLC (JFA), United StatesReviewed by:
Larry Greenwood, Pacific Northwest National Laboratory (DOE), United StatesDavid Brown, Brookhaven National Laboratory (DOE), United States
Mark Stoyer, Lawrence Livermore National Laboratory (DOE), United States
Copyright © 2023 van der Marck and Koning. This is an open-access article distributed under the terms of the Creative Commons Attribution License (CC BY). The use, distribution or reproduction in other forums is permitted, provided the original author(s) and the copyright owner(s) are credited and that the original publication in this journal is cited, in accordance with accepted academic practice. No use, distribution or reproduction is permitted which does not comply with these terms.
*Correspondence: Steven van der Marck, dmFuZGVybWFyY2tAbnJnLmV1