- 1Department of Biology, College of Natural Sciences, Kyungpook National University, Daegu, Republic of Korea
- 2School of Life Sciences, BK21 FOUR KNU Creative BioResearch Group, Kyungpook National University, Daegu, Republic of Korea
- 3Advanced Bio-Resource Research Center, Kyungpook National University, Daegu, Republic of Korea
Microalgae are versatile, profitable, and promising sources of bioenergy and high-value products, having various applications in the biotechnology industry. Herein, G. emersonii KNUA204 was isolated from Ulleungdo Island, South Korea, and exposed to stressors, i.e., MgCl2 (75 and 150 mM) and NaCl (200 and 400 mM), to investigate improvement in its biomass productivity and feasibility of the application of biomass. Treatment with mild MgCl2 (75 mM) afforded the highest specific growth rate (μ = 0.13 d−1), dry cell weight (3 g L−1), and total carbohydrate content (29.87%). Although all salt treatments decreased chlorophyll and carotenoid contents, treatment with high NaCl concentration (400 mM) afforded the highest zeaxanthin content (0.3 mg g−1). The proximate and ultimate analyses of biomass following treatment with 150 mM MgCl2 revealed 93.85% volatile matter and 22.55 MJ kg−1 calorific value, respectively, indicating that Graesiella emersonii KNUA204 can be potentially used as bioenergy feedstock. The biodiesel quality was established based on the fatty acid methyl ester profiles, and MgCl2 treatment increased the cetane number more than the control. Therefore, the treatment of G. emersonii KNUA204 with MgCl2 during cultivation could provide a microalgae-based bioenergy feedstock with high productivity.
1 Introduction
The climate crisis and fossil fuel depletion have increased the demand for sustainable energy and stimulated interest in bioenergy, especially because alternative energy sources are urgently needed worldwide to reduce environmental pollution (Sarkodie et al., 2019; Londoño-Pulgarin et al., 2021; Abbasi et al., 2022). Efforts to achieve carbon neutrality by fixing renewable carbon constitute an effective strategy to develop the full potential of the bioenergy industry (Su et al., 2017; Li et al., 2020). Microalgae exhibit a short cultivation period, high biomass productivity relative to land area, non-competition with crops, and the ability to fix carbon via photosynthesis (De Bhowmick et al., 2019; Musa et al., 2019; Yin et al., 2020; Shanmugam et al., 2021). Consequently, they have emerged as a third-generation energy source through industrial-scale cultivation and appropriate refinery processes (Jambo et al., 2016; Saad et al., 2019; Abbasi et al., 2021; Ananthi et al., 2021). Microalgae can produce various high-value compounds, including lipids, polysaccharides, pigments, and bioactive products (Michalak and Chojnacka, 2015; Deviram et al., 2020; Castillo et al., 2021; Wu et al., 2021). Extensive research has been conducted to obtain high-efficiency biomass to ensure more production of these high-value compounds (Yu et al., 2015; Gomaa et al., 2016; Sun et al., 2018; Shahid et al., 2020; Sivakaminathan et al., 2020).
Salinity constitutes a major stress factor used in research regarding microalgae productivity and high-value compound production (Chen et al., 2017; Liyanaarachchi et al., 2021; Ren et al., 2021). Salt exposure of cells activate complex physiological and biochemical mechanisms, such as the recovery of turgor pressure, regulation of ion uptake and release via cell membranes, and accumulation of osmotic pressure–protective solutes and stress proteins (Hejazi and Wijffels, 2004; Ishika et al., 2018; Figler et al., 2019; Shetty et al., 2019). Therefore, salt stress can increase microalgal growth and lipid content alongside the production of several bioactive compounds (von Alvensleben et al., 2016; Kim et al., 2021; Mohseni et al., 2021). Various studies have reported that salt stress generally increases lipid accumulation, with the proportion of saturated fatty acids increasing and that of polyunsaturated fatty acids decreasing. However, other studies have revealed that under high salt stress conditions, the fatty acids in membrane lipids become unsaturated, resulting in an increase in the proportion of unsaturated fatty acids and a decrease in that of saturated fatty acids (Atikij et al., 2019). Not only lipid but also carotenoid content increased under salinity stress, especially MgCl2, and NaCl (Kobayashi et al., 1997; Rad et al., 2011; Pourkarimi et al., 2020; Lu and Lu, 2022).
Ulleungdo is located east of the Korean Peninsula and is a volcanic island formed by flows of lava derived from volcanic eruptions. Various microbial communities can be discovered in streams originating from the island’s groundwater. However, bacteria and fungi are actively being analyzed, and barely know about microalgal communities (Chang et al., 2002). Studies regarding microalgae originating from Ulleungdo Island are relatively few, thus a more systematic approach is required. Furthermore, the discovery of unique species arising independently on an island far from the Korean Peninsula is possible. Thus, the biochemical characteristics of isolated microalgae strains and the possibility of using them as bioenergy feedstock warrant investigation.
Herein, we isolated the freshwater microalga Graesiella emersonii KNUA204 from Ulleungdo Island, South Korea, and characterized its biomass productivity, biochemical composition, and potential application as bioenergy feedstock under salt stress of MgCl2 and NaCl.
2 Materials and methods
2.1 Isolation and identification of Graesiella emersonii KNUA204
Freshwater samples were collected from Ulleungdo Island, South Korea, in April 2021. Subsequently, the collected samples were inoculated into BG-11 media to obtain an axenic strain based on previously described methods (Jo et al., 2020). The isolated microalga was observed under a light microscope (Zeiss Axio Imager A2; Carl Zeiss, Göttingen, Germany). For molecular identification, genomic DNA was isolated and amplified using polymerase chain reaction (PCR), with each primer set located within the internal transcribed spacer (ITS) region, as previously reported (Abou-Shanab et al., 2011). Each PCR amplicon was ligated into a pGEM T-Easy Vector (Promega, Madison, WI, United States) and transformed into Escherichia coli DH5α. The resulting plasmid DNA was sequenced, and the closely matched sequences were identified using the national center for biotechnology information (NCBI) BLAST tool. A phylogenetic tree was constructed using the maximum likelihood (ML) method with 500 bootstrap replicates (Soltis and Soltis, 2003).
2.2 Growth measurements
2.2.1 Growth conditions
The experiment was performed at 25°C under a light/dark cycle of 16/8 h and 135 μmol m−2 s −1 light intensity using an orbital shaker at 160 rpm (Vision Scientific Korea, Bucheon, Korea). Cell cultures were grown until the optical density at 680 nm (OD680) reached 1.0. Each culture was centrifuged, and the collected pellet was inoculated into the media. Furthermore, salts at four different concentrations (75 mM MgCl2, 150 mM MgCl2, 200 mM NaCl, and 400 mM NaCl) were added to each culture medium. The specific salt concentrations were determined through preliminary research, which indicated that G. emersonii KNUA204 exhibited better growth characteristics under salt stress compared with those of other candidate strains (Supplementary Figure S1).
2.2.2 Measurements of growth rate and biomass productivity
The growth rate of microalgae was monitored via OD680 measurements using a spectrophotometer (Optimizer 2120 UV spectrophotometer, Mecasys, Daejeon, Korea), and their biomass productivity was determined via dry cell weight (DCW) measurement using the gravimetric method, as previously reported (Jo et al., 2020). The OD680 and DCW measurements were conducted every 2 days for 2 weeks. The maximum specific growth rate (μ) was calculated using the following equation (Levasseur et al., 1993):
where N2 and N1 denote the values of OD680 at t2 and t1 days, respectively.
2.3 Measurement of physicochemical properties and pigment analysis
2.3.1 Chlorophyll and total carotenoid contents
The cells were harvested after 14 days, and 2 mL cells were collected and centrifuged at 16,022 ×g for 5 min. The collected cells were suspended in 2 mL ethanol to measure the chlorophyll a (Chl-a), chlorophyll b (Chl-b), and total carotenoid contents. The samples were sonicated using a probe-type ultrasonicator (Branson Ultrasonics Sonifier 450; Danbury, CT, United States) at a resonance frequency of 40 Hz and then incubated at 4°C for 24 h in the dark. Following incubation, the samples were centrifuged at 16,022 ×g for 20 min, and the supernatant was assessed using a UV-VIS spectrophotometer at 470, 649, and 664 nm. Pigment concentrations were calculated using the following equations:
2.3.2 Pigment analysis using high-performance liquid chromatography
High-performance liquid chromatography (HPLC) analysis was performed as described by Yang et al., 2020. The extracted pigments were dissolved in HPLC-grade acetone and filtered through a 0.2-µm membrane filter (Minisart syringe filter, Sartorius Stedim Biotech, Göttingen, Germany). Then, the pigments were analyzed using Agilent 1,200 series gradient HPLC system (Agilent Technologies, Palo Alto, CA, United States) equipped with a C30 carotenoid column (250 mm × 4.6 mm, 5 μm, YMC, Kyoto, Japan). A mixture of 92% methanol and 10 mM ammonium acetate was used as solvent A and tert-butyl methyl ether as solvent B at a constant flow rate of 1 mL min−1 for 1 h. Carotenoid standards (β-carotene, lutein, and zeaxanthin) were purchased from Sigma-Aldrich (St. Louis, MO, United States). The profiles of the standards and extracted pigments were determined via OD measurements at 450 nm.
2.4 Biochemical composition of the microalgal biomass
The harvested microalgal biomass was freeze-dried using a freeze dryer (PVTFD20R, Ilshin Lab, Suwon, Korea), pulverized using a mortar and pestle, and sieved through a test sieve (ASTM No. 230 mesh, opening = 63 μm, Chunggye, Gunpo, Korea). The total carbohydrate content was determined using the phenol–sulfuric acid method, as described previously (Laurens et al., 2012). The total lipid content was determined using the sulfo-phospho-vanillin colorimetric method (Mishra et al., 2014). The protein content was calculated using the conversion factor (×6.25) of nitrogen, and the nitrogen content was derived from the ultimate analysis (Mariotti et al., 2008).
2.5 Proximate and ultimate analysis
Proximate analysis was performed via the thermogravimetric method using a thermal analyzer (DTG-60A, Shimadzu, Kyoto, Japan). After ∼10 mg biomass was utilized, the moisture content was determined by measuring the weight loss before the temperature reached 100°C under nitrogen (N2) supply, volatile matter corresponded to a weight loss between 100°C and 900°C, and the remaining biomass indicated the amount of fixed carbon and ash (Bi and He, 2013). Alpha-alumina (α-Al2O3) powder was used as a reference. Nitrogen (N2) gas was continuously supplied at 25 mL min−1 to protect the microalgal biomass from oxidation.
For the ultimate analysis, the carbon, hydrogen, oxygen, nitrogen, and sulfur contents were determined using Flash 2000 elemental analyzer (Thermo Fisher Scientific, Milan, Italy). The calorific value (CV) was calculated using the following equation (Given et al., 1986):
2.6 Fatty acid analysis and biodiesel properties based on fatty acid methyl ester profiles
Lipid extraction was conducted using a previously described method with slight modifications Breuer et al. (2013). The extracted lipids were transesterified to fatty acid methyl ester (FAME) by adding methanol with 5% (v/v) sulfuric acid and then incubated for 3 h at 70°C. All the samples were filtered before analysis, and the resulting FAME was analyzed via gas chromatography–mass spectrometry (GC–MS) (Agilent 7890A GC equipped with 5975C MSD; Agilent Technologies, Santa Clara, CA, United States) using a DB-FFAP column (30 m × 250 μm × 25 μm). GC–MS was conducted based on our previous study (Jo and Do, 007, 2020).
The biodiesel properties of biomass were determined by assessing the saponification value (SV), iodine value (IV), cetane number (CN), degree of unsaturation (DU), long-chain saturated factor (LCSF), cold filter plugging point (CFPP), oxidation stability (OS), kinematic viscosity (υ), and density (ρ). The following equations were used to estimate the biodiesel quality of biomass using fatty acid composition, as reported previously (Ramos et al., 2009; Islam et al., 2013; Yang et al., 2016):
In these equations, N, MW, D, and X represent the percentages of each fatty acid content, molecular weight, number of double bonds, and linoleic and linolenic acid contents in each FAME value, respectively. MUFA and PUFA represent the contents of monounsaturated and polyunsaturated fatty acids, respectively.
2.7 Statistical analysis
All experiments were performed in triplicate. Data are presented as the average of three values, and error bars represent standard deviation.
3 Results and discussion
3.1 Isolation and identification of Graesiella emersonii KNUA204
In the preliminary research, several indigenous microalgae were isolated from Ulleungdo Island, South Korea, and cultivated with different concentrations of MgCl2 and NaCl to enhance biomass productivity. KNUA204 was selected as the candidate microalga strain because compared with other strains, it exhibited the highest tolerance and sufficient growth level under both mild (75 mM MgCl2 and 200 mM NaCl) and high (150 mM MgCl2 and 400 mM NaCl) salt concentrations (Supplementary Figure S1). KNUA204 was non-motile and ellipsoidal in shape with globose cells, and the size of a single cell was ∼5 μm (Supplementary Figure S1A). Based on the ITS sequencing data, the ML analysis indicated that the isolate was phylogenetically close to G. emersonii (Supplementary Figure S1B).
3.2 Measurement of growth rate and biomass productivity
Previous studies have reported that the growth rate of microalgae decreases with increase in salt concentrations; however, some microalgae species exhibit increased growth rate in mild salt stress conditions (Talebi et al., 2013; Pandit et al., 2017; Elloumi et al., 2020). The growth rate of G. emersonii KNU204 was not significantly reduced by mild salt concentrations. A mild concentration of MgCl2 (75 mM) resulted in the highest growth rate (μ = 0.13 d−1) and DCW (3 g L−1) after 14 days of cultivation. The growth rate and DCW of microalga cultivated in media supplemented with 200 mM NaCl were μ = 0.12 d−1 and 2.6 g L−1, respectively, which were similar to those in the control (BG-11). However, doubling the concentrations of both the salts in the media negatively affected microalgae growth (Figure 1).
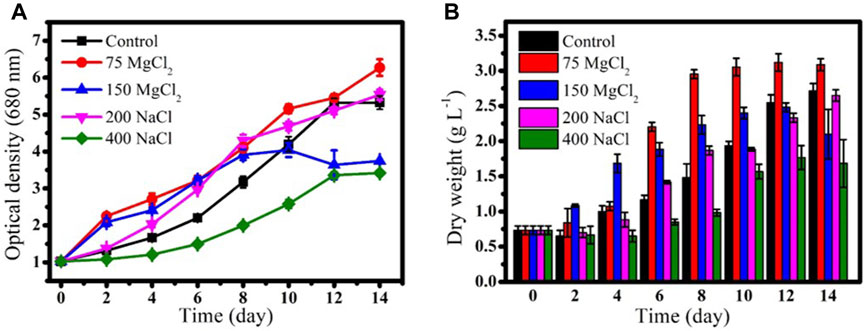
FIGURE 1. (A) Growth curves and (B) dry cell weight of Graesiella emersonii KNUA204 cultured with different concentrations of MgCl2 (75 mM and 150 mM) and NaCl (200 mM and 400 mM).
3.3 Physicochemical properties and carotenoid analysis
In G. emersonii KNU204, >45% Chl-a and Chl-b contents decreased following exposure to MgCl2 and NaCl (Figures 2A, B). The magnitude of decrease in the total carotenoid content was higher in the NaCl-treated cells than in the MgCl2-treated cells (Figure 2C). The highest carotenoid/chlorophyll ratio was achieved with 150 mM MgCl2, suggesting that G. emersonii KNU204 reduced the antenna size to protect themselves against salt stress–induced photo-oxidative damage (Figure 2D) (Pancha et al., 2015). As high carotenoid/chlorophyll ratio is considered an appropriate stress response of microalgae, the addition of 150 mM MgCl2 to the microalgal cultures was possibly the strongest stress factor among those tested here (Saha et al., 2013). Salt stress modifies the concentrations of photosynthetic pigments in numerous microalgae (Romanenko et al., 2017; Elloumi et al., 2020; Fal et al., 2022). The contents of none of the major carotenoids extracted from G. emersonii KNU204 increased under MgCl2 exposure (Figures 2E–G). Previous studies have reported that salt stress leads to increase in β-carotene and xanthophyll contents in microalgae (Chokshi et al., 2017; Sun et al., 2018). Herein, no significant differences in the contents of β-carotene and lutein were observed between the control and salt-treated cells (Figures 2E, F). However, zeaxanthin content increased by more than twice when treated with 400 mM NaCl compared with that in the control (Figure 2G). The overall pigment content in G. emersonii KNU204 decreased in salt-supplemented media, signifying that MgCl2 and NaCl did not efficiently enhance carotenoid and chlorophyll contents. However, treatment with high NaCl concentrations (400 mM) could effectively produce zeaxanthin-rich microalgae.
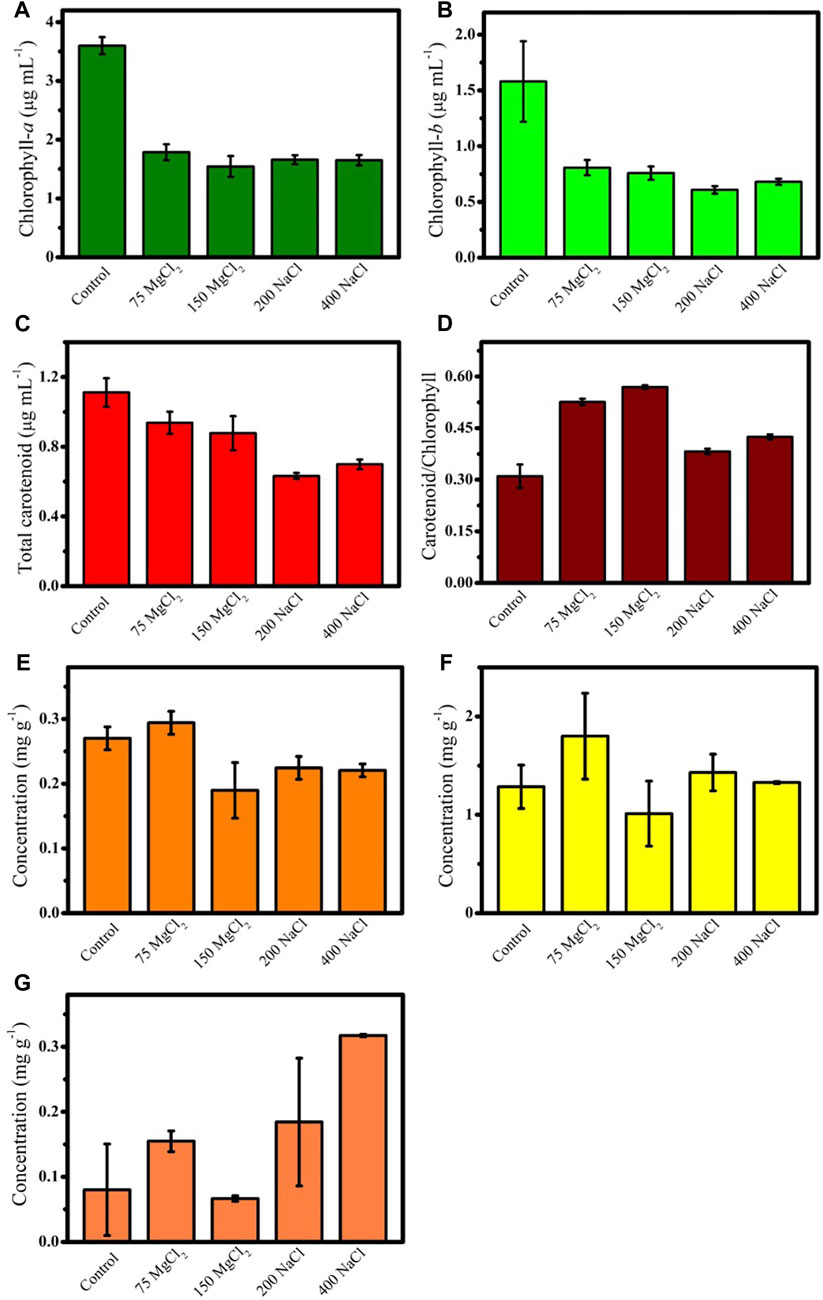
FIGURE 2. Variation of pigment contents in Graesiella emersonii KNUA204 cultivated with different concentrations of MgCl2 (75 mM and 150 mM) and NaCl (200 mM and 400 mM). Contents of (A) chlorophyll a, (B) chlorophyll b, (C) total carotenoid, (D) carotenoid/chlorophyll ratio, (E) β-carotene, (F) lutein, and (G) zeaxanthin.
3.4 Biochemical composition of biomass
Salt stress–induced variations in carbohydrate, protein, and lipid contents in microalgae are species-dependent (Fal et al., 2022; Haris et al., 2022). Furthermore, the appropriate concentration of salt required to alter the amount of bioactive compounds and biochemical products varies from species to species. Herein, the carbohydrate and protein contents of G. emersonii KNU204 increased by 7% and 3%, respectively, under mild salt concentrations, and there was no significant variation in lipid content. Conversely, the carbohydrate and lipid contents considerably decreased under high salt concentrations by >14% and 21%, respectively (Table 1). Various studies have been conducted to increase the lipid or carotenoid content of microalgae, such as of Dunaliella sp., Tetraselmis suecica, Halochlorella rubescens, Scenedesmus sp., Chlamydomonas reinhardtii, and Chlorella vulgaris under salt stress (Pancha et al., 2015; BenMoussa-Dahmen et al., 2016; Sun et al., 2018; Gour et al., 2020; Jo et al., 2020; Fal et al., 2022). However, herein, lipid concentration decreased with increase in salt concentrations. Only the mild MgCl2 concentration (75 mM) barely maintained the same lipid content as that in the control. Compared with the control, the protein content in the culture exposed to the high NaCl concentration (400 mM) increased by 8.44% (Table 1). At this concentration, protein content was also shown to increase in Tetradesmus chuii, Isochrysis galbana, and Chlymydomonas reinhardtii (Haris et al., 2022). As in other microalgae, the accumulation of protein in G. emersonii KNU204 could be due to a protective mechanism against osmotic stress (Anand et al., 2019).
3.5 Proximate and ultimate analysis
In the proximate analysis, the mild MgCl2 concentration (75 mM) afforded the highest VM (93.85%) and the lowest MC (2.38%) means that most of the calorific value would be released (Sukarta_2018). However, the high NaCl concentration (400 mM) increased MC (4.12%) and FC + ash contents (6.90%) and decreased VM (88.98%) compared with those in the control. In the ultimate analysis, the carbon content (48.68%) and CV (22.55 MJ kg−1) were the highest in the 75 mM MgCl2–treated cells (mild concentration) and the lowest in the 150 mM MgCl2–treated cell (high concentration) (Table 2). VM is defined as a part of discharged solid fuel with a content of up to 80% of biomass, usually 63 %–80% for crop residue and 72 %–78% for wood (Hong et al., 2016). High VM content (>80%) in microalgal biomass is advantageous for bioenergy and biofuel production (Awaluddin et al., 2016). The VM of microalgal biomass in all salt stress conditions was higher than the range of VM in wood-based biomass feedstocks. CV was also calculated to determine the potential of this microalgal biomass as biofuel feedstock. All treatments afforded higher CV in the microalga compared with that in terrestrial crops, except the high MgCl2 concentration (150 mM) (17.0–20.0 MJ kg−1); thus, G. emersonii KNUA204 biomass can be used as a potential sustainable source of bioenergy (Ross et al., 2008).
3.6 FAME analysis and assessment of biodiesel properties
The GC–MS results revealed that G. emersonii KNUAU204 contained 26.40% SFA, 22.38% MUFA, and 40.25% PUFA. Based on the biodiesel properties indicated in the FAME profiles, the higher the percentages of SFA and MUFA, higher the CN (Yang et al., 2016), and a high CN of biodiesel can help improve engine performance (Pinzi et al., 2009). Both MgCl2 treatments increased SFA and MUFA contents and decreased PUFA content. Conversely, both NaCl treatments decreased SFA and MUFA contents and increased PUFA content (Supplementary Table S1). Therefore, MgCl2 treatment afforded higher CN than NaCl treatment. Although several values, such as CFPP, OS, and kinetic viscosity, were acceptable in terms of the required biodiesel standards, the IV and CN of all biomass exceeded the standard ranges (EN 14214, ASTM D6751). The cells treated with the high MgCl2 concentration (150 mM) exhibited optimal IV (≤120, EN14214) and the highest CN (Supplementary Table S2). The lowest CN was observed in cells treated with the high NaCl concentration (400 mM), which was attributed to the highest PUFA contents (Ong et al., 2013). Thus, the supply of proper amounts of MgCl2 to cultures can enhance the quality of biodiesel obtained from microalgal biomass.
4 Conclusion
The characteristics of G. emersonii KNUA204, a freshwater indigenous microalga, were investigated under various salt stress conditions. Although the chlorophyll and carotenoid contents decreased under all treatments, the highest specific growth rate and biomass productivity were obtained with a mild MgCl2 concentration (75 mM). The VM and CV of the microalga were higher than those of wood and other crops. Increased IV was further investigated using FAME profiles. Overall, it was confirmed that adequate salt stress conditions could enhance the biomass productivity of G. emersonii KNUA204, thereby rendering it a valuable bioenergy feedstock.
Data availability statement
The original contributions presented in the study are included in the article/Supplementary Material, further inquiries can be directed to the corresponding author.
Author contributions
J-MD was involved in the data analysis, and manuscript writing; HSS was involved in laboratory work, data curation, and editing of the manuscript; H-TY was involved in laboratory work and editing of the manuscript; H-SY was involved in the conceptualization and editing of the manuscript.
Funding
This research was supported by Basic Science Research Program through the National Research Foundation of Korea (NRF) funded by the Ministry of Education (Grant Number 2021R1I1A205551712-1-2). This research was also funded by the Ministry of Oceans and Fisheries, Republic of Korea (grant no. 20220027).
Conflict of interest
The authors declare that the research was conducted in the absence of any commercial or financial relationships that could be construed as a potential conflict of interest.
Publisher’s note
All claims expressed in this article are solely those of the authors and do not necessarily represent those of their affiliated organizations, or those of the publisher, the editors and the reviewers. Any product that may be evaluated in this article, or claim that may be made by its manufacturer, is not guaranteed or endorsed by the publisher.
Supplementary material
The Supplementary Material for this article can be found online at: https://www.frontiersin.org/articles/10.3389/fenrg.2023.1056835/full#supplementary-material
References
Abbasi, K. R., Shahbaz, M., Zhang, J., Irfan, M., and Alvarado, R. (2022). Analyze the environmental sustainability factors of China: The role of fossil fuel energy and renewable energy. Renew. Energy 187, 390–402. doi:10.1016/j.renene.2022.01.066
Abbasi, M., Pishvaee, M. S., and Mohseni, S. (2021). Third-generation biofuel supply chain: A comprehensive review and future research directions. J. Clean. Prod. 323, 129100. doi:10.1016/j.jclepro.2021.129100
Abou-Shanab, R. A. I., Hwang, J. H., Cho, Y., Min, B., and Jeon, B. H. (2011). Characterization of microalgal species isolated from fresh water bodies as a potential source for biodiesel production. Appl. Energy 88, 3300–3306. doi:10.1016/j.apenergy.2011.01.060
Anand, V., Kashyap, M., Samadhiya, K., Ghosh, A., and Kiran, B. (2019). Salinity driven stress to enhance lipid production in Scenedesmus vacuolatus: A biodiesel trigger? Biomass Bioenergy 127, 105252. doi:10.1016/j.biombioe.2019.05.021
Ananthi, V., Raja, R., Carvalho, I. S., Brindhadevi, K., Pugazhendhi, A., and Arun, A. (2021). A realistic scenario on microalgae based biodiesel production: Third generation biofuel. Fuel 284, 118965. doi:10.1016/j.fuel.2020.118965
Atikij, T., Syaputri, Y., Iwahashi, H., Praneenararat, T., Sirisattha, S., Kageyama, H., et al. (2019). Enhanced lipid production and molecular dynamics under salinity stress in green microalga chlamydomonas reinhardtii (137C). Mar. Drugs 17, 484–515. doi:10.3390/md17080484
Awaluddin, S. A., Izhar, S., Hiroyuki, Y., Danquah, M. K., and Harun, R. (2016). Sub-critical water technology for enhance extraction of bioactive compound from microalgae. J. Eng. Sci. Technol. 11, 63–72.
BenMoussa-Dahmen, I., Chtourou, H., Rezgui, F., Sayadi, S., and Dhouib, A. (2016). Salinity stress increases lipid, secondary metabolites and enzyme activity in Amphora subtropica and Dunaliella sp. for biodiesel production. Bioresour. Technol. 218, 816–825. doi:10.1016/j.biortech.2016.07.022
Bi, Z., and He, B. B. (2013). Characterization of microalgae for the purpose of biofuel production. Trans. ASABE 56, 1529–1539. doi:10.13031/trans.56.10090
Breuer, G., Evers, W. A. C., de Vree, J. H., Kleinegris, D. M. M., Martens, D. E., Wijffels, R. H., et al. (2013). Analysis of fatty acid content and composition in microalgae. J. Vis. Exp. 80, e50628. doi:10.3791/50628
Castillo, T., Ramos, D., García-Beltrán, T., Brito-Bazan, M., and Galindo, E. (2021). Mixotrophic cultivation of microalgae: An alternative to produce high-value metabolites. Biochem. Eng. J. 176, 108183. doi:10.1016/j.bej.2021.108183
Chang, K.-I., Kim, Y.-B., Suk, M.-S., and Byun, S.-K. (2002). Hydrography around dokdo. Ocean. Polar Res. 24, 369–389. doi:10.4217/opr.2002.24.4.369
Chen, B., Wan, C., Mehmood, M. A., Chang, J. S., Bai, F., and Zhao, X. (2017). Manipulating environmental stresses and stress tolerance of microalgae for enhanced production of lipids and value-added products–A review. Bioresour. Technol. 244, 1198–1206. doi:10.1016/j.biortech.2017.05.170
Chokshi, K., Pancha, I., Ghosh, A., and Mishra, S. (2017). Salinity induced oxidative stress alters the physiological responses and improves the biofuel potential of green microalgae Acutodesmus dimorphus. Bioresour. Technol. 244, 1376–1383. doi:10.1016/j.biortech.2017.05.003
De Bhowmick, G., Sarmah, A. K., and Sen, R. (2019). Zero-waste algal biorefinery for bioenergy and biochar: A green leap towards achieving energy and environmental sustainability. Sci. Total Environ. 650, 2467–2482. doi:10.1016/j.scitotenv.2018.10.002
Deviram, G., Mathimani, T., Anto, S., Ahamed, T. S., Ananth, D. A., and Pugazhendhi, A. (2020). Applications of microalgal and cyanobacterial biomass on a way to safe, cleaner and a sustainable environment. J. Clean. Prod. 253, 119770. doi:10.1016/j.jclepro.2019.119770
Elloumi, W., Jebali, A., Maalej, A., Chamkha, M., and Sayadi, S. (2020). Effect of mild salinity stress on the growth, fatty acid and carotenoid compositions, and biological activities of the thermal freshwater microalgae Scenedesmus sp. Biomolecules 10, 1515–1517. doi:10.3390/biom10111515
Fal, S., Aasfar, A., Rabie, R., Smouni, A., and Arroussi, H. E. L. (2022). Salt induced oxidative stress alters physiological, biochemical and metabolomic responses of green microalga Chlamydomonas reinhardtii. Heliyon 8, e08811. doi:10.1016/j.heliyon.2022.e08811
Figler, A., B-Béres, V., Dobronoki, D., Márton, K., Nagy, S. A., and Bácsi, I. (2019). Salt tolerance and desalination abilities of nine common green microalgae isolates. WaterSwitzerl. 11, 2527–2617. doi:10.3390/w11122527
Given, P. H., Weldon, D., and Zoeller, J. H. (1986). Calculation of calorific values of coals from ultimate analyses: Theoretical basis and geochemical implications. Fuel 65, 849–854. doi:10.1016/0016-2361(86)90080-3
Gomaa, M. A., Al-Haj, L., and Abed, R. M. M. (2016). Metabolic engineering of Cyanobacteria and microalgae for enhanced production of biofuels and high-value products. J. Appl. Microbiol. 121, 919–931. doi:10.1111/jam.13232
Gour, R. S., Garlapati, V. K., and Kant, A. (2020). Effect of salinity stress on lipid accumulation in Scenedesmus sp. and Chlorella sp.: Feasibility of stepwise culturing. Curr. Microbiol. 77, 779–785. doi:10.1007/s00284-019-01860-z
Haris, N., Manan, H., Jusoh, M., Khatoon, H., Katayama, T., and Kasan, N. A. (2022). Effect of different salinity on the growth performance and proximate composition of isolated indigenous microalgae species. Aquac. Rep. 22, 100925. doi:10.1016/j.aqrep.2021.100925
Hejazi, M. A., and Wijffels, R. H. (2004). Milking of microalgae. Trends Biotechnol. 22, 189–194. doi:10.1016/j.tibtech.2004.02.009
Hong, J. W., Kim, O. H., Jo, S.-W., Kim, H., Jeong, M. R., Park, K. M., et al. (2016). Biochemical composition of a Korean domestic microalga Chlorella vulgaris KNUA027. Microbiol. Biotechnol. Lett. 44, 400–407. doi:10.4014/mbl.1512.12008
Ishika, T., Bahri, P. A., Laird, D. W., and Moheimani, N. R. (2018). The effect of gradual increase in salinity on the biomass productivity and biochemical composition of several marine, halotolerant, and halophilic microalgae. J. Appl. Phycol. 30, 1453–1464. doi:10.1007/s10811-017-1377-y
Islam, M. A., Magnusson, M., Brown, R. J., Ayoko, G. A., Nabi, M. N., and Heimann, K. (2013). Microalgal species selection for biodiesel production based on fuel properties derived from fatty acid profiles. Energies 6, 5676–5702. doi:10.3390/en6115676
Jambo, S. A., Abdulla, R., Mohd Azhar, S. H., Marbawi, H., Gansau, J. A., and Ravindra, P. (2016). A review on third generation bioethanol feedstock. Renew. Sustain. Energy Rev. 65, 756–769. doi:10.1016/j.rser.2016.07.064
Jo, S. W., Hong, J. W., Do, J. M., Na, H., Kim, J. J., Park, S. I., et al. (2020). Nitrogen deficiency-dependent abiotic stress enhances carotenoid production in indigenous green microalga Scenedesmus rubescens KNUA042, for use as a potential resource of high value products. Sustain 12, 5445. doi:10.3390/su12135445
Kim, Z. H., Kim, K., Park, H., Lee, C. S., Nam, S. W., Yim, K. J., et al. (2021). Enhanced fatty acid productivity by parachlorella sp., a freshwater microalga, via adaptive laboratory evolution under salt stress. Biotechnol. Bioprocess Eng. 26, 223–231. doi:10.1007/s12257-020-0001-1
Kobayashi, M., Kurimura, Y., and Tsuji, Y. (1997). Light-independent, astaxanthin production by the green microalga Haematococcus pluvialis under salt stress. Biotechnol. Lett. 19, 507–509. doi:10.1023/A:1018372900649
Laurens, L. M. L., Dempster, T. A., Jones, H. D. T., Wolfrum, E. J., Van Wychen, S., McAllister, J. S. P., et al. (2012). Algal biomass constituent analysis: Method uncertainties and investigation of the underlying measuring chemistries. Anal. Chem. 84, 1879–1887. doi:10.1021/ac202668c
Levasseur, M., Thompson, P. A., and Harrison, P. J. (1993). Physiological acclimation of marine phytoplankton to different nitrogen sources. J. Phycol. 29, 587–595.
Li, X., Damartzis, T., Stadler, Z., Moret, S., Meier, B., Friedl, M., et al. (2020). Decarbonization in complex energy systems: A study on the feasibility of carbon neutrality for Switzerland in 2050. Front. Energy Res. 8, 1–17. doi:10.3389/fenrg.2020.549615
Liyanaarachchi, V. C., Premaratne, M., Ariyadasa, T. U., Nimarshana, P. H. V., and Malik, A. (2021). Two-stage cultivation of microalgae for production of high-value compounds and biofuels: A review. Algal Res. 57, 102353. doi:10.1016/j.algal.2021.102353
Londoño-Pulgarin, D., Cardona-Montoya, G., Restrepo, J. C., and Muñoz-Leiva, F. (2021). Fossil or bioenergy? Global fuel market trends. Renew. Sustain. Energy Rev. 143, 110905. doi:10.1016/j.rser.2021.110905
Lu, Q., and Lu, Y. (2022). Microalga- and yeast-based astaxanthin production via nutrient recovery from wastewater for aquaculture practice: An emerging technology for sustainable development. J. Chem. Technol. Biotechnol. 97, 3035–3048. doi:10.1002/jctb.7164
Mariotti, F., Tomé, D., and Mirand, P. P. (2008). Converting nitrogen into protein - beyond 6.25 and Jones’ factors. Crit. Rev. Food Sci. Nutr. 48, 177–184. doi:10.1080/10408390701279749
Michalak, I., and Chojnacka, K. (2015). Algae as production systems of bioactive compounds. Eng. Life Sci. 15, 160–176. doi:10.1002/elsc.201400191
Mishra, S. K., Suh, W. I., Farooq, W., Moon, M., Shrivastav, A., Park, M. S., et al. (2014). Rapid quantification of microalgal lipids in aqueous medium by a simple colorimetric method. Bioresour. Technol. 155, 330–333. doi:10.1016/j.biortech.2013.12.077
Mohseni, A., Kube, M., Fan, L., and Roddick, F. A. (2021). Treatment of wastewater reverse osmosis concentrate using alginate-immobilised microalgae: Integrated impact of solution conditions on algal bead performance. Chemosphere 276, 130028. doi:10.1016/j.chemosphere.2021.130028
Musa, M., Ayoko, G. A., Ward, A., Rösch, C., Brown, R. J., and Rainey, T. J. (2019). Factors affecting microalgae production for biofuels and the potentials of chemometric methods in assessing and optimizing productivity. Cells 8. doi:10.3390/cells8080851
Ong, H. C., Silitonga, A. S., Masjuki, H. H., Mahlia, T. M. I., Chong, W. T., and Boosroh, M. H. (2013). Production and comparative fuel properties of biodiesel from non-edible oils: Jatropha curcas, Sterculia foetida and Ceiba pentandra. Energy Convers. Manag. 73, 245–255. doi:10.1016/j.enconman.2013.04.011
Pancha, I., Chokshi, K., Maurya, R., Trivedi, K., Patidar, S. K., Ghosh, A., et al. (2015). Salinity induced oxidative stress enhanced biofuel production potential of microalgae Scenedesmus sp. CCNM 1077. Bioresour. Technol. 189, 341–348. doi:10.1016/j.biortech.2015.04.017
Pandit, P. R., Fulekar, M. H., and Karuna, M. S. L. (2017). Effect of salinity stress on growth, lipid productivity, fatty acid composition, and biodiesel properties in Acutodesmus obliquus and Chlorella vulgaris. Environ. Sci. Pollut. Res. 24, 13437–13451. doi:10.1007/s11356-017-8875-y
Pinzi, S., Garcia, I. L., Lopez-Gimenez, F. J., DeCastro, M. D. L., Dorado, G., and Dorado, M. P. (2009). The ideal vegetable oil-based biodiesel composition: A review of social, economical and technical implications. Energy Fuels 23, 2325–2341. doi:10.1021/ef801098a
Pourkarimi, S., Hallajisani, A., Nouralishahi, A., Alizadehdakhel, A., and Golzary, A. (2020). Factors affecting production of beta-carotene from Dunaliella salina microalgae. Biocatal. Agric. Biotechnol. 29, 101771. doi:10.1016/j.bcab.2020.101771
Rad, F. A., Aksoz, N., and Hejazi, M. A. (2011). Effect of salinity on cell growth and β-carotene production in Dunaliella sp. isolates from Urmia Lake in northwest f Iran. Afr. J. Biotechnol. 10, 2282–2289. doi:10.5897/AJB10.1934
Ramos, M. J., Fernández, C. M., Casas, A., Rodríguez, L., and Pérez, Á. (2009). Influence of fatty acid composition of raw materials on biodiesel properties. Bioresour. Technol. 100, 261–268. doi:10.1016/j.biortech.2008.06.039
Ren, Y., Sun, H., Deng, J., Huang, J., and Chen, F. (2021). Carotenoid production from microalgae: Biosynthesis, salinity responses and novel biotechnologies. Mar. Drugs 19, 713. doi:10.3390/md19120713
Romanenko, E. A., Romanenko, P. A., Babenko, L. M., and Kosakovskaya, I. V. (2017). Salt stress effects on growth and photosynthetic pigments’ content in algoculture of Acutodesmus dimorphus (Chlorophyta). Int. J. Algae 19, 271–282. doi:10.1615/InterJAlgae.v19.i3.70
Ross, A. B., Jones, J. M., Kubacki, M. L., and Bridgeman, T. (2008). Classification of macroalgae as fuel and its thermochemical behaviour. Bioresour. Technol. 99, 6494–6504. doi:10.1016/j.biortech.2007.11.036
Saad, M. G., Dosoky, N. S., Zoromba, M. S., and Shafik, H. M. (2019). Algal biofuels: Current status and key challenges. Energies 12, 1920. doi:10.3390/en12101920
Saha, S. K., McHugh, E., Hayes, J., Moane, S., Walsh, D., and Murray, P. (2013). Effect of various stress-regulatory factors on biomass and lipid production in microalga Haematococcus pluvialis. Bioresour. Technol. 128, 118–124. doi:10.1016/j.biortech.2012.10.049
Sarkodie, S. A., Strezov, V., Weldekidan, H., Asamoah, E. F., Owusu, P. A., and Doyi, I. N. Y. (2019). Environmental sustainability assessment using dynamic autoregressive-distributed lag simulations—nexus between greenhouse gas emissions, biomass energy, food and economic growth. Sci. Total Environ. 668, 318–332. doi:10.1016/j.scitotenv.2019.02.432
Shahid, A., Malik, S., Zhu, H., Xu, J., Nawaz, M. Z., Nawaz, S., et al. (2020). Cultivating microalgae in wastewater for biomass production, pollutant removal, and atmospheric carbon mitigation; a review. Sci. Total Environ. 704, 135303. doi:10.1016/j.scitotenv.2019.135303
Shanmugam, S., Hari, A., Kumar, D., Rajendran, K., Mathimani, T., Atabani, A. E., et al. (2021). Recent developments and strategies in genome engineering and integrated fermentation approaches for biobutanol production from microalgae. Fuel 285, 119052. doi:10.1016/j.fuel.2020.119052
Shetty, P., Gitau, M. M., and Maróti, G. (2019). Salinity stress responses and adaptation mechanisms in eukaryotic green microalgae. Cells 8, 1657–1716. doi:10.3390/cells8121657
Sivakaminathan, S., Wolf, J., Yarnold, J., Roles, J., Ross, I. L., Stephens, E., et al. (2020). Light guide systems enhance microalgae production efficiency in outdoor high rate ponds. Algal Res. 47, 101846. doi:10.1016/j.algal.2020.101846
Soltis, P. S., and Soltis, D. E. (2003). Applying the bootstrap in phylogeny reconstruction. Stat. Sci. 18, 256–267. doi:10.1214/ss/1063994980
Su, Y., Song, K., Zhang, P., Su, Y., Cheng, J., and Chen, X. (2017). Progress of microalgae biofuel’s commercialization. Renew. Sustain. Energy Rev. 74, 402–411. doi:10.1016/j.rser.2016.12.078
Sun, H., Zhao, W., Mao, X., Li, Y., Wu, T., and Chen, F. (2018). High-value biomass from microalgae production platforms: Strategies and progress based on carbon metabolism and energy conversion. Biotechnol. Biofuels 11, 227–323. doi:10.1186/s13068-018-1225-6
Talebi, A. F., Tabatabaei, M., Mohtashami, S. K., Tohidfar, M., and Moradi, F. (2013). Comparative salt stress study on intracellular ion concentration in marine and salt-adapted freshwater strains of microalgae. Not. Sci. Biol. 5, 309–315. doi:10.15835/nsb539114
von Alvensleben, N., Magnusson, M., and Heimann, K. (2016). Salinity tolerance of four freshwater microalgal species and the effects of salinity and nutrient limitation on biochemical profiles. J. Appl. Phycol. 28, 861–876. doi:10.1007/s10811-015-0666-6
Wu, J., Gu, X., Yang, D., Xu, S., Wang, S., Chen, X., et al. (2021). Bioactive substances and potentiality of marine microalgae. Food Sci. Nutr. 9, 5279–5292. doi:10.1002/fsn3.2471
Yang, H. W., Song, J. Y., Cho, S. M., Kwon, H. C., Pan, C. H., and Park, Y. Il (2020). Genomic survey of salt acclimation-related genes in the halophilic cyanobacterium euhalothece sp. Z-m001. Sci. Rep. 10, 676–711. doi:10.1038/s41598-020-57546-1
Yang, I. S., Salama, E. S., Kim, J. O., Govindwar, S. P., Kurade, M. B., Lee, M., et al. (2016). Cultivation and harvesting of microalgae in photobioreactor for biodiesel production and simultaneous nutrient removal. Energy Convers. Manag. 117, 54–62. doi:10.1016/j.enconman.2016.03.017
Yin, Z., Zhu, L., Li, S., Hu, T., Chu, R., Mo, F., et al. (2020). A comprehensive review on cultivation and harvesting of microalgae for biodiesel production: Environmental pollution control and future directions. Bioresour. Technol. 301, 122804. doi:10.1016/j.biortech.2020.122804
Keywords: indigenous microalgae, salt stress, bioenergy, biomass productivity, pigment
Citation: Do J-M, Yeo H-T, Suh HS and Yoon H-S (2023) Effect of salt stress on the biomass productivity and potential bioenergy feedstock of Graesiella emersonii KNUA204 isolated from Ulleungdo Island, South Korea. Front. Energy Res. 11:1056835. doi: 10.3389/fenrg.2023.1056835
Received: 29 September 2022; Accepted: 06 February 2023;
Published: 16 February 2023.
Edited by:
Mostafa Ghasemi, Sohar University, OmanReviewed by:
Arivalagan Pugazhendhi, Daegu University, Republic of KoreaMostafa Mohamed El-Sheekh, Tanta University, Egypt
Copyright © 2023 Do, Yeo, Suh and Yoon. This is an open-access article distributed under the terms of the Creative Commons Attribution License (CC BY). The use, distribution or reproduction in other forums is permitted, provided the original author(s) and the copyright owner(s) are credited and that the original publication in this journal is cited, in accordance with accepted academic practice. No use, distribution or reproduction is permitted which does not comply with these terms.
*Correspondence: Ho-Sung Yoon, aHN5QGtudS5hYy5rcg==