- Department of Earth and Environmental Engineering, Department of Chemical Engineering, Lenfest Center for Sustainable Energy, The Earth Institute, Columbia University, New York, NY, United States
Ever-increasing anthropogenic CO2 emissions have required us to develop carbon capture, utilization, and storage (CCUS) technologies, and in order to address climate change, these options should be at scale. In addition to engineered systems of CO2 capture from power plants and chemical processes, there are emerging approaches that include the Earth (i.e., air, Earth, and ocean) within its system boundary. Since oceans constitute the largest natural sink of CO2, technologies that can enhance carbon storage in the ocean are highly desired. Here, we discuss alkalinity enhancement and biologically inspired CO2 hydration reactions that can shift the equilibrium of ocean water to pump more carbon into this natural sink. Further, we highlight recent work that can harvest and convert CO2 captured by the ocean into chemicals, fuels, and materials using renewable energy such as off-shore wind. Through these emerging and innovative technologies, organic and inorganic carbon from ocean-based solutions can replace fossil-derived carbon and create a new carbon economy. It is critical to develop these ocean-based CCUS technologies without unintended environmental or ecological consequences, which will create a new engineered carbon cycle that is in harmony with the Earth’s system.
Introduction
Ever-rising global anthropogenic carbon emissions accounted for 40 GtCO2 y−1 in 2020 (Friedlingstein et al., 2020) and thus it is critical to effectively manage carbon through natural and engineered systems to address climate change (Caldeira et al., 2018; Watson et al., 2020; National Academies, 2019; National Academies, 2022; Kelemen et al., 2020). During the past few decades, significant effort has been devoted to capturing CO2 from industrial point sources such as coal-fired and natural gas-fired power plants. While the world moves towards the rapid decarbonization of industrial sectors, negative emission technologies (NETs) provide an important pathway to capture historically emitted CO2. For example, technologies that can directly capture CO2 from the atmosphere, called direct air capture (DAC), are currently in development (Sanz-Perez et al., 2016). DAC is challenging because of the very low concentration of CO2 in air (i.e., ∼420 ppm in air (Tans and Keeling, 2021) compared to 3–15% in flue gas of power plants (Songolzadeh et al., 2014)) and demands unique requirements such as a low pressure drop and high oxidative thermal stability. There have been significant advancements in the design and scale-up of air contactors, and efforts are currently focused on the development of more efficient and selective DAC materials and regeneration methods. (Sanz-Perez et al., 2016). CO2 captured from the atmosphere with DAC can be considered “non-fossil carbon” and can be used to produce chemicals, materials, and fuels using renewable energy. Thus, DAC holds promise to not only provide a pathway towards a negative emission technology, but also to create a new carbon economy that is not based on fossil resources.
Historically emitted CO2 can be found in three places in the environment–the air (ca. 420 ppm gaseous CO2), the Earth (e.g., mineral carbonates and CO2 in soil), and in the ocean. A surprisingly untapped source of carbon can be found in the Earth’s oceans. The ocean is the largest natural sink of CO2 and is thought have absorbed at least 2.5 PgC yr−1 of anthropogenic carbon from 1994–2007 (Watson et al., 2020). In 2019, the Global Carbon Budget found that oceans absorbed 2.6 GtC y−1 (Friedlingstein et al., 2020). Within oceans, most human-made CO2 is found at shallow depths (<1,000 m) (Caldeira et al., 2018), and with the continued increase in CO2 concentration in the atmosphere, the pH of the shallow ocean is significantly lower than at deep levels leading to ocean acidification (DeVries et al., 2017; Caldeira et al., 2018). Importantly, the concentration of inorganic carbon in oceans (0.099 kgCO2 m−3) is 125 times higher than the concentration of CO2 in air (Khatiwala et al., 2013; Sanz-Perez et al., 2016; Patterson et al., 2019), which suggests that direct ocean capture (DOC) of CO2 in ocean or seawater that does not interfere with marine aquatic life (Eisaman et al., 2018; Fuss et al., 2018) is another important pathway for carbon capture technologies (Sharifian et al., 2021; Terlouw et al., 2021). Carbon captured by the ocean is distributed and converted to different forms including dissolved inorganic carbon and marine biomass, as illustrated in Figure 1.
Carbon dissolved in ocean or seawater primarily consists of carbonic acid (H2CO3), bicarbonate (HCO3−), and carbonate (CO32–). Carbon dioxide dissolution into ocean water is controlled by the partial pressure of carbon dioxide in the atmosphere and the pH and alkalinity of water. Once dissolved in ocean water, carbon dioxide is in equilibrium with bicarbonate (pKa = 5.9) (Mojica Prieto and Millero, 2002) and carbonate (pKa = 9.1) (Mojica Prieto and Millero, 2002) following Eqs. 1–3
Thus, at a typical oceanic surface pH of 8.2, most dissolved carbon is speciated as HCO3−, not dissolved carbon dioxide. This is more clearly seen in Figure 2, which plots the speciation of CO2 in seawater at different pH values. While HCO3− is the predominant carbon species at ocean water pH, CO2 can be evolved if the pH is lowered. The total alkalinity of ocean water can be measured to determine the extent of carbonate dissociation. Total alkalinity (AT) is defined as the proton deficiency in a solution relative to a baseline. As the alkalinity of ocean water increases, more dissociation of carbonic acid and bicarbonate occur and more carbonate is produced. Thus, the addition of alkalinity into the ocean would increase the total CO2 uptake by the ocean.
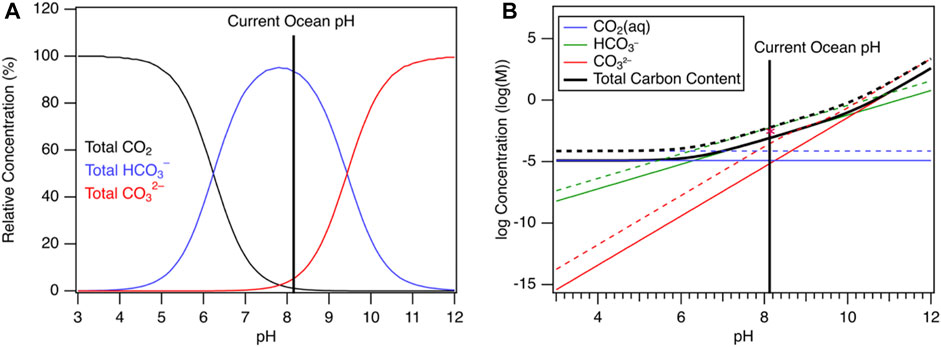
FIGURE 2. (A) Bjerrum plot showing the speciation of dissolved inorganic carbon in a background of seawater (Riebesell et al., 2010) at fixed total carbon concentration at 25°C (B) CO2 speciation vs pH for pure (solid lines) and seawater (Riebesell et al., 2010) (dotted lines) at fixed partial pressure in an open CO2 system at 25°C. Calculations were performed with ChemEQL (Müller, 2015). The asterisk denotes the total carbon content of water as calculated in Ref. (Riebesell et al., 2010).
As shown in Figure 1, carbon in the ocean can also be used by aquatic life like marine biomass, corals, and shellfish. Because of climate change, the marine biome is rapidly transforming and the health of aquatic life is threatened. Thus, direct ocean capture (DOC) and utilizing marine biomass for energy and material production have emerged as unique engineered solutions for climate change mitigation because they provide a sustainable carbon balance in the ocean that is important for the Earth’s stability. Here, we discuss how oceans store, capture, and convert CO2 and how ocean-based technologies can be developed to harvest non-fossil carbon from the ocean to create a new carbon economy.
Storing carbon in the ocean
As discussed, the ocean is the largest natural sink for carbon. Below, we suggest several avenues that accelerate and increase CO2 uptake by the ocean including added alkalinity and enzymatic catalysis.
Enhanced carbon sequestration in the ocean via added alkalinity
The ocean can capture and store CO2 as carbonates on geologic time scales. Unfortunately, the anthropogenic CO2 emission rate is too fast for the ocean to keep up with, and thus, there is an imbalance of carbon leading to ocean acidification. In order to restore the ocean’s pH balance while storing more carbon in the ocean, several methods have been considered. These technologies rely on the formation enthalpies of adding aqueous metal ions like Ca2+ and Mg2+ to bicarbonate and carbonate-rich ocean water (Renforth and Henderson, 2017). The ocean already contains 0.010 mol kg−1 Ca2+ and 0.052 mol kg−1 Mg2+, but its capacity for additional alkalinity is vast (Riebesell et al., 2010). An additional source of alkaline metals can be found in earth-abundant minerals and rocks such as limestone (CaCO3), ultramafic rocks (e.g., olivine ((Mg,Fe)2SiO4), and serpentine (Mg3Si2O5(OH)4)). These can be mined, processed, and dissolved to obtain Ca2+ and Mg2+, (Renforth et al., 2013; La Plante et al., 2021), and their addition to the ocean water results in the capture and subsequent transformation of atmospheric CO2 to bicarbonate (dominant carbon species at pH 8.2) and carbonate ions.
An early example of this technology used CaO and Ca(OH)2 which easily dissolve and increase the bicarbonate concentration in ocean water (Kheshgi, 1995). Most CaO and Ca(OH)2 are currently produced from Earth abundant limestone (CaCO3) (Kenny and Oates, 2007; Kantzas et al., 2022), but they can also be derived from industrial alkaline wastes such as mine tailings, waste-to-energy ashes, iron and steel slag, and waste concrete (Renforth, 2019; Bui Viet et al., 2020; Gadikota, 2021; Hong et al., 2021; Rim et al., 2021). Since limestone already contains one carbon per calcium, a maximum of only 1 mol of additional CO2 can be captured by producing bicarbonate solutions from CaCO3. On the other hand, if calcium and magnesium-bearing silicate minerals such as olivine, (Gadikota et al., 2014), serpentine, (Park et al., 2008), wollastonite, (Zhao et al., 2013), pyroxenes, and plagioclase feldspar are used, the CO2 capture and storage capacity of this reaction can theoretically be doubled, since those alkaline metals are extracted without any attached carbon. In reality, the CO2 capture capacity of these silicate-bearing minerals would be slightly less than two because the calcium or magnesium to CO2 ratio would depend on the source of alkalinity and pH of the oceanwater. If magnesium and calcium ions are added into the ocean, the overall equilibrium shown in Figure 2 will shift and additional CO2 will be captured from the air into the ocean. Example reactions of calcium-bearing minerals are given below (Eqs. 4–8).
From carbonate minerals:
From silicate minerals without carbonate phases:
Magnesium-bearing minerals would undergo similar reactions capturing and storing CO2. One challenge is that silicate minerals are generally less reactive than carbonate minerals in ocean water, and thus, the process is often kinetically limited. Other recent work has shown that industrial wastes such as fly ash, steel slag, and cement dust can also be used for ocean alkalinity enhancement (Gadikota et al., 2020).
In all of these reactions, the rate-limiting process is thought to be mineral dissolution. Ex-situ mineral dissolution can significantly accelerate Ca2+ and Mg2+ leaching from different sources (on a time scale of hours or less) (Gerdemann et al., 2007; Gadikota et al., 2014). Alkaline calcium- and magnesium-rich solutions can be added to the ocean at desired locations, though this ex-situ technology would require the construction of engineered reactor systems and infrastructure. Mineral dissolution processes often use chemicals including acids and ligands to accelerate the calcium and magnesium leaching process. A few emerging technologies have been proposed, including the production of acid (e.g., HCl) from the ocean water via electrolysis using renewable energy (House et al., 2007). The strong acid produced can rapidly dissolve minerals to be added to the ocean (Rau, 2008; Rau et al., 2013). The use of this “renewable” acid in ocean-based solutions will also be discussed in the later section on the electrochemical recovery of CO2 from the ocean.
In-situ mineral dissolution utilizes the ocean surface to perform mineral dissolution, and it is often limited in terms of mineral dissolution rates since mixing and reaction temperatures are bound by the environmental conditions, and chemical additives cannot be used. Thus, it is important to select the appropriate approach for different regions and varied mineral compositions (i.e., in-situ versus ex-situ). The CarbFix project in Iceland has shown that alkaline metals can be effectively liberated in water saturated with CO2 and ultimately form solid carbonates (Snæbjörnsdóttir et al., 2020). A similar approach can be used to tune the ocean alkalinity. CarbFix2 is investigating this approach using captured CO2 from water treatment plants for mineralization using seawater (Snæbjörnsdóttir et al., 2020; Carbfix, 2022). A complementary approach, sCS2 (single step carbon sequestration and storage), which involves electrolytic precipitation of carbonates from CO2 and seawater may also provide interesting ocean based solutions (La Plante et al., 2021). Another noteworthy project is from the CarbonSAFE program in the United States, which aims to inject CO2 into ocean basalt at Cascaida Basin (Goldberg et al., 2018).
One important challenge for alkaline addition technologies is the energy efficient delivery of large quantities of alkaline-bearing materials (e.g., CaO, Ca(OH)2, Ca-silicates, etc.) to acidified ocean water. We believe that new and innovative offshore technologies and existing transportation systems can address some of the fundamental issues in ocean-based CO2 sequestration. For instance, shipping lines (Hassellöv et al., 2013) are major contributors to ocean acidification, and thus, it has been proposed that one method to combat localized acidification is to carry minerals and carbonates on tankers to different parts of the ocean (Butenschön et al., 2021; Caserini et al., 2021). For this use it would be important to control the rate of alkaline addition to the ocean water to ensure that local change in pH and alkalinity will not harmfully impact local ecosystems. Local changes for in situ carbonation approaches would likely also affect the carbonate chemistry of seawater, which could have important and/or unknown implications for calcifiers.
Another potential issue with mineral addition to ocean water would be increased local concentrations of unwanted dissolved species. This is critical for alkaline industrial wastes containing nickel (serpentine mine tailings), iron and cerium (iron and steel slag), and a wide range of heavy metals like zinc, iron, and lead (waste-to-energy plant ashes). These metal ions could have unwanted and potentially toxic effects to life found in ocean water (Hartmann et al., 2013; Montserrat et al., 2017; Fuss et al., 2018). For example, low concentrations of nickel (∼0.3 µM) are known to be cytotoxic to Mytilus edulis (blue mussel) (Montserrat et al., 2017). Thus, any impurities detrimental to the environment and the eco-system should be removed from these waste materials before they are added to ocean water. An ex-situ approach that first produces relatively pure calcium- and magnesium-bearing materials derived from silicates or alkaline industrial wastes would greatly improve the environmental friendliness of the proposed alkaline addition technology. Furthermore, the ex-situ approach will minimize the mass of alkaline materials that must be delivered to different ocean sites by separating out impurities and non-alkaline materials from the feedstock.
Biologically inspired technologies using carbonic anhydrase
Carbonic anhydrases are a metalloenzyme family that biologically catalyze CO2 hydration and dehydration. They are found in all mammals, plants, algae, fungi, and bacteria (Elleuche and Poggeler, 2009). Carbonic anhydrases play a key role in the ocean’s carbon balance by accelerating rate limiting steps of CO2 ocean uptake. They also are involved in CO2 homeostasis, biosynthetic reactions, lipogenesis, ureagenesis, and calcification, among other processes relevant to life in the ocean (Supuran, 2016). In nature, carbonic anhydrases are found in five different classes, ɑ, β, ɣ, δ, and ζ. The active site of carbonic anhydrases contains a tetrahedral metal (II) ion prosthetic group coordinated with three histidine side chains and a water molecule. This provides access to a metal hydroxide active species that can bind CO2 and produce a metal bicarbonate complex. In most carbonic anhydrases, Zn2+ is the catalytically active metal ion, though Fe2+ and Cd2+ are thought to be central to some carbonic anhydrases. Co2+ has also been observed to substitute Zn2+ with little activity loss. Carbonic anhydrases are thought to mediate the hydration of CO2 through the mechanism proposed in Figure 3, shown with a Zn2+ metal center. Here, a tetrahedral Zn2+ aquo complex is deprotonated, forming a highly nucleophilic zinc hydroxide active site. Diffusion of CO2 into the active site and nucleophilic attack on CO2 generates the Zn2+ bicarbonate. Further displacement of the bicarbonate with water allows the completion of the enzymatic catalytic cycle.
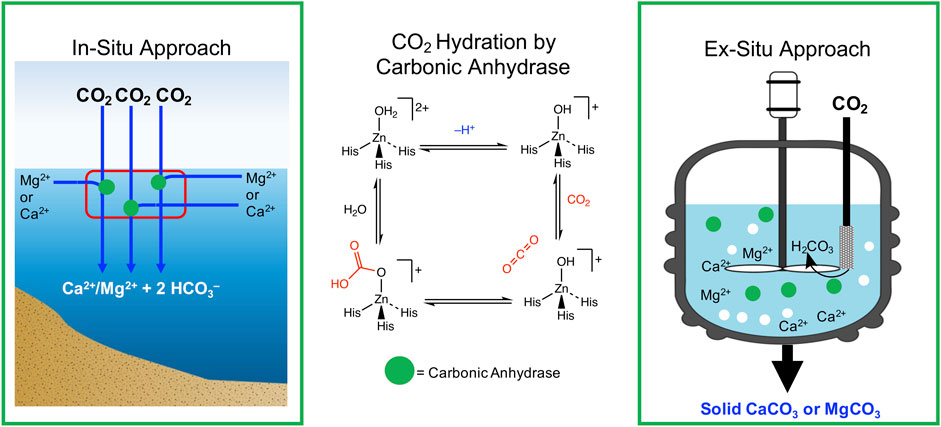
FIGURE 3. Illustration of how carbonic anhydrase can be used to increase alkalinity in both an in-situ and ex-situ approach. The middle panel of the figure describes the proposed mechanism for CO2 hydration (His = histidine).
For CDR/NET technologies, carbonic anhydrase is useful because it helps improve the rate of CO2 uptake by catalyzing the rate of CO2 hydration which is often the rate limiting step in these reactions. The reaction can also operate in the reverse direction. Thus, several groups have shown that carbonic anhydrase can catalyze the dissolution of calcites in seawater to enhance the rate of alkalinity addition into the ocean (Liu et al., 2005; Li et al., 2009; Xie and Wu, 2013; Subhas et al., 2017). In these systems, CO2 is bubbled into a solution containing the carbonic anhydrase enzyme and calcite particles. The carbonic anhydrase rapidly equilibrates dissolved CO2 with that in the gas phase (i.e., atmosphere), resulting in a reactive system that is limited by gas exchange kinetics. The rapid dissolution of CO2 by carbonic anhydrase provides additional protons for the calcite dissolution. Thus, calcite precipitation may be minimized as carbonic anhydrase catalyzes CO2 hydration reaction. One of the challenges associated with natural carbonic anhydrase is its thermal and long-term stability, and thus there are efforts to encapsulate and immobilize carbonic anhydrase and to incorporate it into innovative reactor designs such as a monolithic reactor and a fluidized bed reactor (Patel et al., 2013; Patel et al., 2014a; Patel et al., 2014b; Boucif et al., 2020; Fabbricino et al., 2021; Verma et al., 2021).
Another emerging area of research is biologically inspired catalysis mimicking carbonic anhydrase. Small molecule chemistry has been explored to synthesize homogenous metal catalysts with significantly improved thermal stability compared to natural carbonic anhydrase, which can thermally denature under the conditions required for CO2 adsorption and desorption (Koziol et al., 2012). The coordination center of Zn2+ in the carbonic anhydrase active site has been modeled by several groups using various amine and tris(pyrazolylborate) ligand systems (Kimura et al., 1990; Looney et al., 1993; Schrodt et al., 1997; Khan et al., 2012; Koziol et al., 2012; Floyd et al., 2013; Liu et al., 2013; Verma et al., 2021). In principle, these systems are thought to react with CO2 using the same mechanism as shown in Figure 3. Other types of mono, dinuclear, and trinuculear complexes, including molecules with copper or nickel centers, have also been used to capture CO2 (Verma et al., 2021), (Bernauer et al., 2002; Kolks et al., 2002; Appel et al., 2005; Verdejo et al., 2008; Mateus et al., 2011). One of the best studied catalysts in this area is the 1,4,7,10-tetraazacyclododecane Zn(II) (zinc cyclen), which, because of its low molecular weight, only shows a 5-fold lower activity than natural carbonic anhydrase (Koziol et al., 2012). Zinc cyclen is found to remain active at industrially relevant temperatures as high as 75°C (Koziol et al., 2012).
Harvesting inorganic and organic carbon captured and stored in the ocean
In order to achieve deep decarbonization and address climate change, we need to go beyond zero emission technologies and develop a multifaceted set of NETs (Negative Emission Technologies). Further, we need to create a new carbon economy that is not based on fossil carbon, and it would be closely integrated with a new hydrogen economy. The locations and scale of different types of carbon are shown in Figure 1. With the rapid deployment of renewable energy including off-shore wind and its significantly reduced cost, we now have a great potential to harvest inorganic ocean carbon and convert it to high value chemicals, materials, and fuels. The direct recovery of carbon from ocean water can be achieved via different chemical and biological pathways. There are a number of interesting studies that focus on cultivating and harvesting marine biomass. Marine biomass is attractive for energy and chemical production because it utilizes the large ocean surface and does not require land use.
To convert and utilize inorganic carbon that is stored in the ocean, an equilibrium shifting approach, where acid is directly added to carbonate-rich water to shift its equilibrium back to CO2 (see Eqs. 1–3, Figure 2), is often used. An electrochemical acidification cell employs an ion-exchange membrane with an applied voltage to trade sodium ions for protons (Willauer et al., 2011). As a result, acid (e.g., HCl) and base (e.g., NaOH) can be produced using renewable electricity, and they can be used to either capture or release CO2 from the ocean by shifting equilibrium. In these systems, hydrogen gas is also evolved as a byproduct, and thus, the carbon and hydrogen economies can be effectively integrated.
Tandem capture and conversion of ocean carbon to chemicals, fuels, and materials
A number of innovative electrochemical systems have been employed to capture and convert CO2 stored in the ocean. As bicarbonates and carbonates become CO2 via electrochemical equilibrium shifting, they are converted to low molecular weight carbon products (C1 or C2). For example, Digdaya et al. recently reported an innovative approach to capture and convert dissolved carbon in the ocean to CO with total Faradaic efficiencies up to 95% (Digdaya et al., 2020). Their device operates using a bipolar membrane electrodialysis cell. There, ion transport through the membranes generates a proton gradient (as discussed above), acidifying the ocean water and liberating CO2. Dissolved CO2 is then reduced using Ag or Cu electrodes. The silver catalyst system was found to be highly selective for CO production. Other examples of these types of electrochemical systems use bipolar membranes to facilitate the electrolytic conversion of CO2 derived from ocean carbonates and bicarbonates into low carbon products, including CO (Li et al., 2019; Lees et al., 2020) and formate (HCO2−) (Li et al., 2020). With copper electrodes, a wide array of reduction products are found, including larger carbon containing products like ethanol, propanol, and acetate (Digdaya et al., 2020). These organic compounds as fuels or chemical feedstocks can play a role in a new carbon economy.
Conversion of carbon in marine biomass to chemicals, fuels, and materials
During the last several years, there has been a growing movement to use algae, microalgae, and seaweed biomass to produce biofuels (Sayre, 2010; Zhao and Su, 2014). Among the advantages of biomass are its high production efficiency, available cultivation area that does not compete with current land use, and ability to capture and convert CO2 and carbonates dissolved in aqueous environments. Seaweeds, which have myriad uses and are cultivated on large scales, can sequester seven times the carbon dioxide of terrestrial lignocellulosic biomass (not normalized) (Zhang et al., 2020). These types of marine and salt water biomass have active bicarbonate pumps that can concentrate and dehydrate bicarbonates with carbonic anhydrases. The CO2 captured in this biological process is then converted to sugars via photosynthesis. The need for efficient cultivation and harvesting of marine biomass in the open ocean has led to innovations in automated robotic systems (Zereik et al., 2018). Off-shore biomass conversion systems are also being considered (Nassar et al., 2020).
Marine biomass can be converted into high-energy liquid fuels or chemicals that can be directly used in the current carbon economy. There are a number of biomass conversion technologies including anaerobic digestion, gasification, and pyrolysis. Compared to terrestrial biomass, the water and salt contents of marine biomass are significantly higher, and thus, their conversion pathways are limited. Thus, innovative technologies that can directly work with wet and salty biomass, such as seaweed, are desired.
Recently, we have demonstrated a unique approach that converts seaweed to bio-hydrogen (Zhang et al., 2020). Our alkaline thermal treatment (ATT) of wet, brown seaweed with NaOH and a Ni/ZrO2 catalyst produced bio-hydrogen, while suppressing the formation of COx, at moderate temperatures (<500°C) under ambient pressure. In this process, we use solar energy stored in marine biomass to produce bio-derived H2, while the carbon and nutrients (e.g., N, P, etc.) will return to the ocean as dissolved species. This innovative reaction scheme produces bio-derived hydrogen, while marine biomass is converted to solid carbonates, as illustrated in Figure 4. By using Ca(OH)2 derived from alkaline industrial wastes such as iron and steel slags and fly ash rather than freshly mined limestone (CaCO3), the ATT reaction can be performed with reduced overall energy requirement, while treating solid wastes and minimizing their landfill requirement. A net equation is shown in Eq. 9. The integration of two chemical reaction loops, alkaline thermal treatment of marine biomass and carbon mineralization, allows a new pathway towards a negative emission bioenergy production, since carbon from marine biomass can be sequestered into the ocean as bicarbonates. Other technologies that maximize carbon atom efficiencies and provide long-term carbon storage should be investigated. These new pathways might be useful not only to produce existing chemicals and materials, but to produce new chemicals and materials through highly integrated innovative technologies.
An important emerging area that can be directly tied with the ATT technology is the recovery of strategically important critical elements that are found dilute concentration in the ocean or in marine biomass. While the absolute concentration of many rare Earth elements (REEs) found in seawater are often quite low (31.8–168 pmol L−1 found in the western Pacific Ocean) (Deng et al., 2017), those REE concentrations found in biomass, like seaweeds can be significantly higher. Those REE contents are highest for La and Ce, but can be found from 1–100 ng g−1 in a variety of seaweeds. Moreover, the concentration of REEs in ash derived from seaweeds is significantly higher, such that the concentrations of La and Ce can rise above 100 ng g−1 (Fu et al., 2002; Piarulli et al., 2021). In the ATT reaction outlined above, in addition to net CO2 storage in the form of carbonates, we also produce biochar residues from marine biomass with concentrated REEs and other critical elements at levels similar to unconventional resource streams (e.g., waste-to-energy ashes or steel/iron slag). An interesting future direction would be to integrate the ATT production of H2 and storage of CO2 with downstream leaching and separation steps to recover rare Earth elements like Ce or La from these materials.
As shown above, H2 can also be produced through electrochemical means from seawater (see Tandem Capture and Conversion Section). Electrochemical approaches also hold promise as NETs that can produce H2 (Rau and Baird, 2018; Rau et al., 2018). The question for which process will ultimately provide a higher impact on CO2 concentrations is still undecided, and clearly both technologies are worth pursuing. The ATT strategy shown here, we believe, holds enormous promise. Solar concentrators could be used to heat the reactions, which rely on fairly modest temperatures. Moreover, we see the potential for not only negative-emission H2 production, but also the concentration of elements of strategic importance to future clean energy innovations.
Final remarks
Oceanic carbon capture, conversion, and storage offer an enticing alternative route to address urgent climate change at scale. The present level of engineered CO2 uptake by the ocean, outside of adding alkalinity and using an electrochemical acidification process, is underexplored. Thus, significant effort should be devoted to addressing critical challenges and opportunities in this area, while creating a better environment for marine life and restoring the Earth’s carbon balance. With the rapid increase in affordable renewable energy, we now have synergistic opportunities to capture, convert, and store organic and inorganic carbon in the ocean and replace fossil-derived carbon. Innovative electrochemical systems allow tandem reactions that convert CO2 derived from bicarbonate and carbonates to value-added products (e.g., CO and formate). In another approach, alkaline thermal treatment can produce bio-hydrogen and store carbon in solid carbonates.
Including the environment into the system boundary of engineered solutions often has undesired consequences, particularly when deployed at large industrial scales. There could be local spikes in ocean pH, a significant rise in metal concentrations leading to harmful algal plumes and increased toxicity. Thus, it is critical to consider all the short-term and long-term environmental and ecological impacts of each engineered solution before it is implemented at a large scale. Further, the deployment of these ocean-based carbon management solutions requires innovative technological development of systems. These include the efficient transportation of materials to the site and reactors that may have to operate in a distributed mode with renewable energy. The automation and process intensification would be very important for such technology development. The use of marine industries, such as container shipping lines, to access large areas of the ocean to deploy CCUS technologies (e.g., added alkalinity) are potential synergistic options. These ocean-based CCUS solutions are great examples of how we can work with the ocean to develop a new carbon economy, while creating a better planet for all.
Data availability statement
The original contributions presented in the study are included in the article/supplementary material, further inquiries can be directed to the corresponding author.
Author contributions
HV and AHP conceived of and wrote the manuscript.
Funding
This work was supported by Korea Institute of Energy Technology Evaluation and Planning (KETEP) grant funded by the Korea government (MOTIE) (No. 20188550000580) and NSF CBET 1927336 (AccelNet-SCO2RE).
Conflict of interest
The authors declare that the research was conducted in the absence of any commercial or financial relationships that could be construed as a potential conflict of interest.
Publisher’s note
All claims expressed in this article are solely those of the authors and do not necessarily represent those of their affiliated organizations, or those of the publisher, the editors and the reviewers. Any product that may be evaluated in this article, or claim that may be made by its manufacturer, is not guaranteed or endorsed by the publisher.
References
Appel, A. M., Newell, R., DuBois, D. L., and Rakowski DuBois, M. (2005). Concentration of carbon dioxide by electrochemically modulated complexation with a binuclear copper complex. Inorg. Chem. 44 (9), 3046–3056. doi:10.1021/ic050023k
Bernauer, K., Cabort, A., Guicher, N., Stoeckli-Evans, H., and Süss-Fink, G. (2002). Carbonate binding to copper(II) in solution: Mixed-ligand complex formation and its application to the isolation and separation of the three isomers of [Cu(bpp)(H2O)] [ClO4]2 [bpp = 2, 6-bis(pyrrolidin-2-yl)pyridine]. J. Chem. Soc. Dalton Trans. (9), 2069–2073. doi:10.1039/b111501b
Boucif, N., Roizard, D., and Favre, E. (2020). “The carbonic anhydrase promoted carbon dioxide capture,” in Membranes for environmental applications. Environmental chemistry for a sustainable world Editors Z. Zhang, W. Zhang, and E. Lichtfouse (Springer, Cham), vol 42. doi:10.1007/978-3-030-33978-4_1
Bui Viet, D., Chan, W. P., Phua, Z. H., Ebrahimi, A., Abbas, A., and Lisak, G. (2020). The use of fly ashes from waste-to-energy processes as mineral CO2 sequesters and supplementary cementitious materials. J. Hazard. Mat. 398, 122906. doi:10.1016/j.jhazmat.2020.122906
Butenschön, M., Lovato, T., Masina, S., Caserini, S., and Grosso, M. (2021). Alkalinization scenarios in the mediterranean sea for efficient removal of atmospheric CO2 and the mitigation of ocean acidification. Front. Clim. 3, 614537. doi:10.3389/fclim.2021.614537
Caldeira, K., Akai, M., Brewer, P., Chen, B., Haugan, P., Iwama, T., et al. (2018). Ch. 6. Ocean storage. Intergovermental Panel on Climate Change.
Carbfix (2022). Project CO2 Seastone. Available from: https://www.carbfix.com/co2-seastone (Accessed September 11, 2022).
Caserini, S., Pagano, D., Campo, F., Abbà, A., De Marco, S., Righi, D., et al. (2021). Potential of maritime transport for ocean liming and atmospheric CO2 removal. Front. Clim. 3. doi:10.3389/fclim.2021.575900
Deng, Y., Ren, J., Guo, Q., Cao, J., Wang, H., and Liu, C. (2017). Rare Earth element geochemistry characteristics of seawater and porewater from deep sea in Western Pacific. Sci. Rep. 7 (1), 16539. doi:10.1038/s41598-017-16379-1
DeVries, T., Holzer, M., and Primeau, F. (2017). Recent increase in oceanic carbon uptake driven by weaker upper-ocean overturning. Nature 542 (7640), 215–218. doi:10.1038/nature21068
Digdaya, I. A., Sullivan, I., Lin, M., Han, L., Cheng, W. H., Atwater, H. A., et al. (2020). A direct coupled electrochemical system for capture and conversion of CO2 from oceanwater. Nat. Commun. 11 (1), 4412. doi:10.1038/s41467-020-18232-y
Eisaman, M. D., Rivest, J. L. B., Karnitz, S. D., de Lannoy, C-F., Jose, A., DeVaul, R. W., et al. (2018). Indirect ocean capture of atmospheric CO2: Part II. Understanding the cost of negative emissions. Int. J. Greenh. Gas Control 70, 254–261. doi:10.1016/j.ijggc.2018.02.020
Elleuche, S., and Poggeler, S. (2009). Evolution of carbonic anhydrases in fungi. Curr. Genet. 55 (2), 211–222. doi:10.1007/s00294-009-0238-x
Fabbricino, S., Del Prete, S., Russo, M. E., Capasso, C., Marzocchella, A., and Salatino, P. (2021). In vivo immobilized carbonic anhydrase and its effect on the enhancement of CO2 absorption rate. J. Biotechnol. 336, 41–49. doi:10.1016/j.jbiotec.2021.06.016
Floyd, W. C., Baker, S. E., Valdez, C. A., Stolaroff, J. K., Bearinger, J. P., Satcher, J. H., et al. (2013). Evaluation of a carbonic anhydrase mimic for industrial carbon capture. Environ. Sci. Technol. 47 (17), 10049–10055. doi:10.1021/es401336f
Friedlingstein, P., O'Sullivan, M., Jones, M. W., Andrew, R. M., Hauck, J., Olsen, A., et al. (2020). Global carbon Budget 2020. Earth Syst. Sci. Data 12 (4), 3269. doi:10.5194/essd-12-3269-2020
Fu, F. F., Akagi, T., Yabuki, S., Iwaki, M., and Ogura, N. (2002). Distribution of rare Earth elements in seaweed: Implication of two different sources of rare Earth elements and silicon in seaweed. J. Phycol. 36 (1), 62–70. doi:10.1046/j.1529-8817.2000.99022.x
Fuss, S., Lamb, W. F., Callaghan, M. W., Hilaire, J., Creutzig, F., Amann, T., et al. (2018). Negative emissions—Part 2: Costs, potentials and side effects. Environ. Res. Lett. 13 (6), 063002. doi:10.1088/1748-9326/aabf9f
Gadikota, G., Matter, J., Kelemen, P., Brady, P. V., and Park, A-H. A. (2020). Elucidating the differences in the carbon mineralization behaviors of calcium and magnesium bearing alumino-silicates and magnesium silicates for CO2 storage. Fuel, 117900. doi:10.1016/j.fuel.2020.117900
Gadikota, G., Matter, J., Kelemen, P., and Park, A-H. A. (2014). Chemical and morphological changes during olivine carbonation for CO2 storage in the presence of NaCl and NaHCO3. Phys. Chem. Chem. Phys. 16 (10), 4679–4693. doi:10.1039/c3cp54903h
Gadikota, G. (2021). Carbon mineralization pathways for carbon capture, storage and utilization. Commun. Chem. 4 (1), 23. doi:10.1038/s42004-021-00461-x
Gerdemann, S. J., O'Connor, W. K., Dahlin, D. C., Penner, L. R., and Rush, H. (2007). Ex situ aqueous mineral carbonation. Environ. Sci. Technol. 41 (7), 2587–2593. doi:10.1021/es0619253
Goldberg, D., Aston, L., Bonneville, A., Demirkanli, I., Evans, C., Fisher, A., et al. (2018). Geological storage of CO2 in sub-seafloor basalt: The CarbonSAFE pre-feasibility study offshore Washington state and British columbia. Energy Procedia 146, 158–165. doi:10.1016/j.egypro.2018.07.020
Hartmann, J., West, A. J., Renforth, P., Köhler, P., De La Rocha, C. L., Wolf-Gladrow, D. A., et al. (2013). Enhanced chemical weathering as a geoengineering strategy to reduce atmospheric carbon dioxide, supply nutrients, and mitigate ocean acidification. Rev. Geophys. 51 (2), 113–149. doi:10.1002/rog.20004
Hassellöv, I. M., Turner, D. R., Lauer, A., and Corbett, J. J. (2013). Shipping contributes to ocean acidification. Geophys. Res. Lett. 40 (11), 2731–2736. doi:10.1002/grl.50521
Hong, S., Park, A-H. A., and Park, Y. (2021). Evaluation of elemental leaching behavior and morphological changes of steel slag in both acidic and basic conditions for carbon sequestration potential. Korean J. Chem. Eng. 38, 2279–2285. doi:10.1007/s11814-021-0874-5
House, K. Z., House, C. H., Schrag, D. P., and Aziz, M. J. (2007). Electrochemical acceleration of chemical weathering as an energetically feasible approach to mitigating anthropogenic climate change. Environ. Sci. Technol. 41 (24), 8464–8470. doi:10.1021/es0701816
Kantzas, E. P., Val Martin, M., Lomas, M. R., Eufrasio, R. M., Renforth, P., Lewis, A. L., et al. (2022). Substantial carbon drawdown potential from enhanced rock weathering in the United Kingdom. Nat. Geosci. 15 (5), 382–389. doi:10.1038/s41561-022-00925-2
Kelemen, P. B., McQueen, N., Wilcox, J., Renforth, P., Dipple, G., and Vankeuren, A. P. (2020). Engineered carbon mineralization in ultramafic rocks for CO2 removal from air: Review and new insights. Chem. Geol., 550.
Kenny, M., and Oates, T. (2007). Lime and limestone. Ullmann's Encyclopedia of Industrial Chemistry2007.
Khan, S., Roy, S., Bhar, K., Kumar, R. K., Maji, T. K., and Ghosh, B. K. (2012). Syntheses, structures and properties of μ3-carbonato bridged trinuclear zinc(II) complexes containing a tailored tetradentate amine. Polyhedron 32 (1), 54–59. doi:10.1016/j.poly.2011.07.001
Khatiwala, S., Tanhua, T., Mikaloff Fletcher, S., Gerber, M., Doney, S. C., Graven, H. D., et al. (2013). Global ocean storage of anthropogenic carbon. Biogeosciences 10 (4), 2169–2191. doi:10.5194/bg-10-2169-2013
Kheshgi, H. S. (1995). Sequestering atmospheric carbon dioxide by increasing ocean alkalinity. Energy 20 (9), 915–922. doi:10.1016/0360-5442(95)00035-f
Kimura, E., Shiota, T., Koike, T., Shiro, M., and Kodama, M. (1990). A zinc(II) complex of 1, 5, 9-triazacyclododecane ([12]aneN3) as a model for carbonic anhydrase. J. Am. Chem. Soc. 112 (15), 5805–5811. doi:10.1021/ja00171a020
Kolks, G., Lippard, S. J., and Waszczak, J. V. (2002). A tricopper(II) complex containing a triply bridging carbonate group. J. Am. Chem. Soc. 102 (14), 4832–4833. doi:10.1021/ja00534a045
Koziol, L., Valdez, C. A., Baker, S. E., Lau, E. Y., Floyd, W. C., Wong, S. E., et al. (2012). Toward a small molecule, biomimetic carbonic anhydrase model: Theoretical and experimental investigations of a panel of zinc(II) aza-macrocyclic catalysts. Inorg. Chem. 51 (12), 6803–6812. doi:10.1021/ic300526b
La Plante, E. C., Simonetti, D. A., Wang, J., Al-Turki, A., Chen, X., Jassby, D., et al. (2021). Saline water-based mineralization pathway for gigatonne-scale CO2 management. ACS Sustain. Chem. Eng. 9 (3), 1073–1089. doi:10.1021/acssuschemeng.0c08561
Lees, E. W., Goldman, M., Fink, A. G., Dvorak, D. J., Salvatore, D. A., Zhang, Z., et al. (2020). Electrodes designed for converting bicarbonate into CO. ACS Energy Lett. 5 (7), 2165–2173. doi:10.1021/acsenergylett.0c00898
Li, T., Lees, E. W., Goldman, M., Salvatore, D. A., Weekes, D. M., and Berlinguette, C. P. (2019). Electrolytic conversion of bicarbonate into CO in a flow cell. Joule 3 (6), 1487–1497. doi:10.1016/j.joule.2019.05.021
Li, T., Lees, E. W., Zhang, Z., and Berlinguette, C. P. (2020). Conversion of bicarbonate to formate in an electrochemical flow reactor. ACS Energy Lett. 5 (8), 2624–2630. doi:10.1021/acsenergylett.0c01291
Li, W., Zhou, P. P., Jia, L. P., Yu, L. J., Li, X. L., and Zhu, M. (2009). Limestone dissolution induced by fungal mycelia, acidic materials, and carbonic anhydrase from fungi. Mycopathologia 167 (1), 37–46. doi:10.1007/s11046-008-9143-y
Liu, X., Du, P., and Cao, R. (2013). Trinuclear zinc complexes for biologically relevant μ3-oxoanion binding and carbon dioxide fixation. Nat. Commun. 4, 2375. doi:10.1038/ncomms3375
Liu, Z., Yuan, D., and Dreybrodt, W. (2005). Comparative study of dissolution rate-determining mechanisms of limestone and dolomite. Environ. Geol. 49 (2), 274–279. doi:10.1007/s00254-005-0086-z
Looney, A., Han, R., McNeill, K., and Parkin, G. (1993). Tris(pyrazolyl)hydroboratozinc hydroxide complexes as functional models for carbonic anhydrase: On the nature of the bicarbonate intermediate. J. Am. Chem. Soc. 115 (11), 4690–4697. doi:10.1021/ja00064a033
Mateus, P., Delgado, R., Lloret, F., Cano, J., Brandao, P., and Felix, V. (2011). A trinuclear copper(II) cryptate and its μ3-CO3 cascade complex: Thermodynamics, structural and magnetic properties. Chem. Eur. J. 17 (40), 11193–11203. doi:10.1002/chem.201101372
Mojica Prieto, F. J., and Millero, F. J. (2002). The values of pK1 + pK2 for the dissociation of carbonic acid in seawater. Geochim. Cosmochim. Acta 66 (14), 2529–2540. doi:10.1016/s0016-7037(02)00855-4
Montserrat, F., Renforth, P., Hartmann, J., Leermakers, M., Knops, P., and Meysman, F. J. (2017). Olivine dissolution in seawater: Implications for CO2 sequestration through enhanced weathering in coastal environments. Environ. Sci. Technol. 51 (7), 3960–3972. doi:10.1021/acs.est.6b05942
Müller, B. (2015). ChemEQL - a software for the calculation of chemical equilibria. Kastanienbau, Switzerland: Swiss Federal Institute of Aquatic Science and Technology.
Nassar, W. M., Anaya-Lara, O., Ahmed, K. H., Campos-Gaona, D., and Elgenedy, M. (2020). Assessment of multi-use offshore platforms: Structure classification and design challenges. Sustainability 12 (5), 1860. doi:10.3390/su12051860
National Academies, (2019). Negative emissions technologies and reliable sequestration: A research agenda. Washington, DC: National Academies of Sciences Engineering, and Medicine
Park, A-H. A., Jadhav, R., and Fan, L-S. (2008). CO2 mineral sequestration: Chemically enhanced aqueous carbonation of serpentine. Can. J. Chem. Eng. 81 (3-4), 885–890. doi:10.1002/cjce.5450810373
Patel, T. N., Park, A. H., and Banta, S. (2013). Periplasmic expression of carbonic anhydrase in Escherichia coli: A new biocatalyst for CO2 hydration. Biotechnol. Bioeng. 110 (7), 1865–1873. doi:10.1002/bit.24863
Patel, T. N., Park, A. H., and Banta, S. (2014). Surface display of small peptides on Escherichia coli for enhanced calcite precipitation rates. Biopolymers 102 (2), 191–196. doi:10.1002/bip.22466
Patel, T. N., Swanson, E. J., Park, A-H. A., and Banta, S. (2014). An automated method for measuring the operational stability of biocatalysts with carbonic anhydrase activity. Biochem. Eng. J. 82, 48–52. doi:10.1016/j.bej.2013.11.003
Patterson, B. D., Mo, F., Borgschulte, A., Hillestad, M., Joos, F., Kristiansen, T., et al. (2019). Renewable CO2 recycling and synthetic fuel production in a marine environment. Proc. Natl. Acad. Sci. U. S. A. 116 (25), 12212–12219. doi:10.1073/pnas.1902335116
Piarulli, S., Hansen, B. H., Ciesielski, T., Zocher, A. L., Malzahn, A., Olsvik, P. A., et al. (2021). Sources, distribution and effects of rare Earth elements in the marine environment: Current knowledge and research gaps. Environ. Pollut. 291, 118230. doi:10.1016/j.envpol.2021.118230
Rau, G. H., and Baird, J. R. (2018). Negative-CO2-emissions ocean thermal energy conversion. Renew. Sustain. Energy Rev. 95, 265–272. doi:10.1016/j.rser.2018.07.027
Rau, G. H., Carroll, S. A., Bourcier, W. L., Singleton, M. J., Smith, M. M., and Aines, R. D. (2013). Direct electrolytic dissolution of silicate minerals for air CO2 mitigation and carbon-negative H2 production. Proc. Natl. Acad. Sci. U. S. A. 110 (25), 10095–10100. doi:10.1073/pnas.1222358110
Rau, G. H. (2008). Electrochemical splitting of calcium carbonate to increase solution alkalinity: Implications for mitigation of carbon dioxide and ocean acidity. Environ. Sci. Technol. 42 (23), 8935–8940. doi:10.1021/es800366q
Rau, G. H., Willauer, H. D., and Ren, Z. J. (2018). The global potential for converting renewable electricity to negative-CO2-emissions hydrogen. Nat. Clim. Chang. 8 (7), 621–625. doi:10.1038/s41558-018-0203-0
Renforth, P., and Henderson, G. (2017). Assessing ocean alkalinity for carbon sequestration. Rev. Geophys. 55 (3), 636–674. doi:10.1002/2016rg000533
Renforth, P., Jenkins, B. G., and Kruger, T. (2013). Engineering challenges of ocean liming. Energy 60, 442–452. doi:10.1016/j.energy.2013.08.006
Renforth, P. (2019). The negative emission potential of alkaline materials. Nat. Commun. 10 (1), 1401. doi:10.1038/s41467-019-09475-5
National Academies (2022). A research strategy for ocean-based carbon dioxide removal and sequestration. National Academies of Arts and Sciences
U. Riebesell, V. J. Fabry, L. Hansson, and J-P. Gattuso (Editors) (2010). Part 1: Sewater carbonate chemistry (Luxembourg: Publications Office of the European Union).
Rim, G., Roy, N., Zhao, D., Kawashima, S., Stallworth, P., Greenbaum, S. G., et al. (2021). CO2 utilization in built environment via the PCO2 swing carbonation of alkaline solid wastes with different mineralogy. Faraday Discuss. 230 (0), 187–212. doi:10.1039/d1fd00022e
Sanz-Perez, E. S., Murdock, C. R., Didas, S. A., and Jones, C. W. (2016). Direct capture of CO2 from ambient air. Chem. Rev. 116 (19), 11840–11876. doi:10.1021/acs.chemrev.6b00173
Sayre, R. (2010). Microalgae: The potential for carbon capture. BioScience 60 (9), 722–727. doi:10.1525/bio.2010.60.9.9
Schrodt, A., Neubrand, A., and van Eldik, R. (1997). Fixation of CO2 by zinc(II) chelates in alcoholic medium. X-Ray structures of {[Zn(cyclen)]3(μ3-CO3)}(ClO4)4 and [Zn(cyclen)EtOH](ClO4)2. Inorg. Chem. 36 (20), 4579–4584. doi:10.1021/ic961368t
Sharifian, R., Wagterveld, R. M., Digdaya, I. A., Xiang, C., and Vermaas, D. A. (2021). Electrochemical carbon dioxide capture to close the carbon cycle. Energy Environ. Sci. 14 (2), 781–814. doi:10.1039/d0ee03382k
Snæbjörnsdóttir, S. Ó., Sigfússon, B., Marieni, C., Goldberg, D., Gislason, S. R., and Oelkers, E. H. (2020). Carbon dioxide storage through mineral carbonation. Nat. Rev. Earth Environ. 1 (2), 90–102. doi:10.1038/s43017-019-0011-8
Songolzadeh, M., Soleimani, M., Takht Ravanchi, M., and Songolzadeh, R. (2014). Carbon dioxide separation from flue gases: A technological review emphasizing reduction in greenhouse gas emissions. Sci. World J. 2014, 828131–828134. doi:10.1155/2014/828131
Subhas, A. V., Adkins, J. F., Rollins, N. E., Naviaux, J., Erez, J., and Berelson, W. M. (2017). Catalysis and chemical mechanisms of calcite dissolution in seawater. Proc. Natl. Acad. Sci. U. S. A. 114 (31), 8175–8180. doi:10.1073/pnas.1703604114
Supuran, C. T. (2016). Structure and function of carbonic anhydrases. Biochem. J. 473 (14), 2023–2032. doi:10.1042/bcj20160115
Tans, P., and Keeling, R. (2021). Trends in atmospheric carbon dioxide. San Diego, CA: Scripps Institution of Oceanography.
Terlouw, T., Bauer, C., Rosa, L., and Mazzotti, M. (2021). Life cycle assessment of carbon dioxide removal technologies: A critical review. Energy Environ. Sci. 14, 1701–1721. doi:10.1039/d0ee03757e
Verdejo, B., Blasco, S., González, J., García-España, E., Gaviña, P., Tatay, S., et al. (2008). CO2 fixation and activation by CuII complexes of 5, 5″-terpyridinophane macrocycles. Eur. J. Inorg. Chem. 2008 (1), 84–97. doi:10.1002/ejic.200700767
Verma, M., Bhaduri, G. A., Phani Kumar, V. S., and Deshpande, P. A. (2021). Biomimetic catalysis of CO2 hydration: A materials perspective. Ind. Eng. Chem. Res. 60 (13), 4777–4793. doi:10.1021/acs.iecr.0c06203
Watson, A. J., Schuster, U., Shutler, J. D., Holding, T., Ashton, I. G. C., Landschutzer, P., et al. (2020). Revised estimates of ocean-atmosphere CO2 flux are consistent with ocean carbon inventory. Nat. Commun. 11 (1), 4422. doi:10.1038/s41467-020-18203-3
Willauer, H. D., DiMascio, F., Hardy D, R., Lewis, M. K., and Williams, F. W. (2011). Extraction of carbon dioxide from seawater by an electrochemical acidification cell. Part II- laboratory scaling studies. Washington, DC: Naval Research Laboratory.
Xie, T., and Wu, Y. (2013). The role of microalgae and their carbonic anhydrase on the biological dissolution of limestone. Environ. Earth Sci. 71 (12), 5231–5239. doi:10.1007/s12665-013-2925-7
Zereik, E., Bibuli, M., Mišković, N., Ridao, P., and Pascoal, A. (2018). Challenges and future trends in marine robotics. Annu. Rev. Control 46, 350–368. doi:10.1016/j.arcontrol.2018.10.002
Zhang, K., Kim, W. J., and Park, A-H. A. (2020). Alkaline thermal treatment of seaweed for high-purity hydrogen production with carbon capture and storage potential. Nat. Commun. 11 (1), 3783. doi:10.1038/s41467-020-17627-1
Zhao, B., and Su, Y. (2014). Process effect of microalgal-carbon dioxide fixation and biomass production: A review. Renew. Sustain. Energy Rev. 31, 121–132. doi:10.1016/j.rser.2013.11.054
Keywords: climate change, direct ocean capture, ocean acidification, carbonate, alkaline thermal treatment
Citation: Vibbert HB and Park A-HA (2022) Harvesting, storing, and converting carbon from the ocean to create a new carbon economy: Challenges and opportunities. Front. Energy Res. 10:999307. doi: 10.3389/fenrg.2022.999307
Received: 20 July 2022; Accepted: 29 August 2022;
Published: 21 September 2022.
Edited by:
Tao Wang, Zhejiang University, ChinaReviewed by:
Qingyang Lin, Zhejiang University, ChinaCopyright © 2022 Vibbert and Park. This is an open-access article distributed under the terms of the Creative Commons Attribution License (CC BY). The use, distribution or reproduction in other forums is permitted, provided the original author(s) and the copyright owner(s) are credited and that the original publication in this journal is cited, in accordance with accepted academic practice. No use, distribution or reproduction is permitted which does not comply with these terms.
*Correspondence: Ah-Hyung Alissa Park, ap2622@columbia.edu