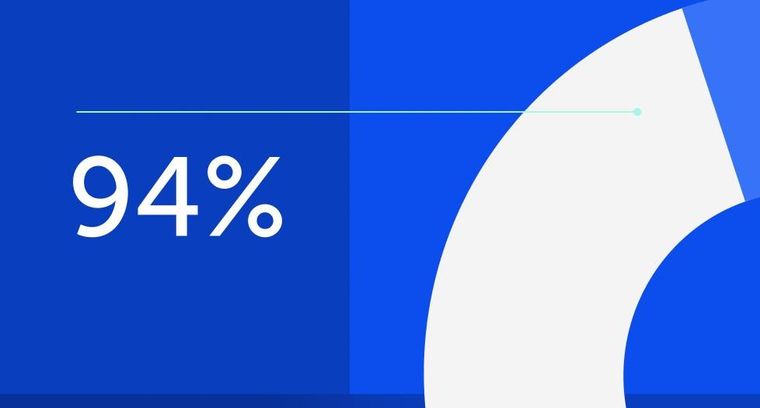
94% of researchers rate our articles as excellent or good
Learn more about the work of our research integrity team to safeguard the quality of each article we publish.
Find out more
ORIGINAL RESEARCH article
Front. Energy Res., 24 August 2022
Sec. Advanced Clean Fuel Technologies
Volume 10 - 2022 | https://doi.org/10.3389/fenrg.2022.984144
This article is part of the Research TopicThe Challenge and Opportunity of CCUS in the Development of Unconventional ResourceView all 15 articles
One of the important technologies for combating global climate change is CCUS (Carbon Capture, Utilization, and Storage), which aims to address the issue of “greenhouse effect” generated by a significant amount of greenhouse gas emissions. Supercritical CO2, a new type of anhydrous fracturing fluid with broad application prospects in low-pressure tight sandstone gas reservoirs, has several advantages over traditional water-based fracturing fluids, including quick flowback, minimal damage to the reservoir, and the ability to realize in-situ storage of greenhouse gases. The tight sandstone cores from the Jinqiu Gas field in the Sichuan Basin were used in an experimental investigation on the interaction mechanism between supercritical CO2 and tight sandstone to examine the viability of supercritical CO2 fracturing with tight sandstone, and analysis of the samples’ post-reaction samples’ mineral composition, microstructure, mass change, and total salinity change. The interaction of tight sandstone with supercritical CO2 results in an increase in quartz content, a decrease in clay mineral content, the formation of new minerals, and partial mineral dissolution on the surface of the sample. Since the degree of mineral dissolution of sandstone samples increases with time, CO2 can be stored in tight sandstone as carbonate minerals. This study evaluates how supercritical CO2 interacts with tight sandstone and can offer a solid theoretical foundation and experimental evidence in favor of CO2 in-situ storage in tight sandstone gas reservoir.
The “greenhouse effect,” which is brought on by a significant quantity of greenhouse gas emissions, has sparked widespread worry as social economy continues to advance and global industrialisation picks up speed. The average CO2 concentration in the atmosphere has risen to its greatest level in almost a million years since the globe started the industrialization era, and temperatures are increasing (Grainger and Smith, 2021). Both the ecosystem of the world and the advancement of human society are in grave danger. Future climate change will cause a number of chain reactions that will get worse as time goes on, including an increase in extreme weather occurrences, a rise in sea level, and the extinction of marine and terrestrial ecosystems (Zou et al., 2021). If CO2 emissions continue to climb, the world’s temperature will rise by 2°C by the end of the century, the sea level will rise by almost 1 m, most coral reefs will vanish, 13% of terrestrial ecosystems will be lost, and many plants and animals may be in danger (Umar et al., 2022). Although the industrial age has brought about great advancement and ease, it has also led to major environmental issues and unsustainable expansion.
The Paris Climate Agreement, which proposed achieving the goal of “net zero emissions” of CO2 around 2050, or carbon neutrality, was adopted at the 21st United Nations Climate Change Conference in order to address global climate change (Zhao et al., 2022), realize the advancement of human civilization, and ensure the sustainable development of the earth’s ecosystem (Tapia et al., 2018). As a result, countries have adopted policies that reflect the significance of lowering greenhouse gases, particularly CO2 emissions.
The geological storage method of CO2 is currently the best efficient way to deal with CO2, a greenhouse gas. It involves gathering the CO2 gas produced by fixed point sources (industrial point sources or power plants) and storing it in a reasonably closed geological structure (Hasan et al., 2015). All deep salt water bearing strata, oil and gas reservoirs, barren coal seams, and deep ocean have the perfect storage location. Their individual CO2 storage mechanisms, however, differ as a result of the various storage sites.
Researchers proposed three pathways for CO2 disposal in deep saline water layer in the CO2 storage in deep saline water layer study (Wang et al., 2021). First, in a process known as dissolution landfill technology, CO2 is directly dissolved in formation water. This approach is expected to raise the acidity of the formation water, which will increase the solubility of minerals in the parent rock because the formation water with CO2 dissolved is already mildly acidic (Zhou et al., 2019); Second, after CO2 injection, it directly or indirectly reacts with minerals in the formation to produce secondary carbonate minerals, which are precipitated in the form of new minerals (Zhou et al., 2018). Third, CO2 is stored under the cover of low permeability rock in the form of gas or supercritical fluid, that is, CO2 is loaded into “sealed tank,” which is typically referred to as liquid landfill technology (Yue et al., 2022). Mineral landfill technique is the name given to this process. This process has a good chance of success since it can solidify CO2 into a component of solid minerals over an extended period of time.
Theoretical study and practical experience demonstrate that oil and gas reservoirs can store CO2 for a long period because of their effective capping. The chance of CO2 stored there leaking out is the smallest compared to other geological entities such deep salt water layers, basalt, and non-minable coal seams. Additionally, it is easier to store CO2 and has higher economic benefits now that production wells and injection wells have been constructed in the oil and gas reservoirs. A long-term technique of storing CO2 is in oil and gas reservoirs. One of the primary methods for storing CO2 is to inject supercritical CO2 into oil and gas reservoirs using oil field injection wells or production wells (Bachu, 2016). Therefore, increased oil recovery is paired with CO2 reservoir storage as the primary method. Oil and gas reservoir storage uses CO2 to force crude oil to flow to production wells, similar to how water drive enhance oil recovery. The main mechanism of CO2 oil displacement involves interphase mass transfer, crude oil volume expansion, viscosity reduction, lowering of oil-gas interfacial tension, and oil-gas mixing, all of which occur during the contact between CO2 and crude oil (Mouahid et al., 2022).
Supercritical CO2 has been employed as a working fluid in various theoretical and experimental studies in petroleum engineering (Nikolai et al., 2019; Peng et al., 2019), and it can be used to displace oil. Supercritical CO2 hydraulic fracturing is regarded as one of the most environmentally benign fracturing technologies since it has several benefits in reservoirs (Zhao et al., 2017; Hazarika and Boruah, 2022), particularly for water-sensitive formations (Liu et al., 2014), low pressure reservoirs, and water-stressed regions (Cao et al., 2017). Although it is well known that supercritical CO2 has many advantages over water-based fracturing fluid, the majority of the work is still done on shale (Jiang et al., 2016; Jia et al., 2018; Memon et al., 2022). For example, the matrix minerals dissolve (Ao et al., 2017), the dissolved minerals precipitate (Chen et al., 2022), the rock breakdown pressure is lowered (Gathitu et al., 2009; Wang et al., 2018; Peng et al., 2020), the rock matrix swells (Day et al., 2008), and so on. Uncertainty exists regarding the exact mechanism of action between supercritical CO2 and tight sandstone. Tight sandstone and supercritical CO2 will interact physicochemically during supercritical CO2 fracturing (Peng et al., 2016). The flow condition of subsurface oil and gas will be impacted by changes in tight sandstone’s pore characteristics, porosity, permeability, and other physical qualities (Lin et al., 2008; Lin et al., 2022). The evaluation of the mineral composition, microstructure, mass change, and total salinity change of supercritical CO2 fracturing in low pressure tight sandstone reservoirs is therefore critical; nevertheless, there have only been a few publications in the literature up to this point.
This study examines the feasibility of fracturing supercritical CO2 with tight, low-pressure sandstone. We investigate how tight sandstone and supercritical CO2 interact. Different tight sandstone samples were treated with supercritical CO2 using the tight sandstone cores from the Jinqiu Gas field in the Sichuan Basin, and the results were evaluated for changes in mineral composition, microstructure, mass change, and total salinity under various time, pressure, and temperature conditions. To further enhance the interaction mechanism between supercritical CO2 and low pressure tight sandstone, quantify the impact of supercritical CO2 on the mineral composition, microstructure, mass change, and total salinity of tight sandstone. This discovery evaluates the mechanism of supercritical CO2’s interaction with tight sandstone, and it can offer a theoretical foundation and experimental support for CO2 in-situ storage in tight sandstone gas reservoirs.
The channel sand bodies in the Shaximiao Formation in the Sichuan Basin are found to be very developed longitudinally, widely dispersed on the plane, with good reservoir physical properties, and to have great exploration potential, according to recent comprehensive geological research on the Shaximiao Formation System. The three-dimensional carving stereogram of river sand body is shown in Figure 1 (Zhang and Yang, 2022).
FIGURE 1. Three dimensional carving stereogram of channel sand body of Shaximiao Formation in Central Sichuan.
The Shaximiao formation’s natural gas has the advantages of shallow burial, low cost, short cycle, and quick effect. At the moment, it is one of the important practical fields of benefit development under low oil prices, and has drawn a lot of attention. Major natural gas exploration breakthroughs have been made in Qiulin, Jinhua, Yanting, and other locations in the central and western Sichuan region. As a result, Shaximiao formation has emerged as the Sichuan Basin’s newest focus for oil and gas exploration (Zhang et al., 2022).
The reservoir temperature is 1.9–2.5°C/100 m, the reservoir depth is 1,500–2800 m, the cumulative thickness of the reservoir is 20–70 m, the reservoir porosity is mainly 8%–15%, the permeability is mainly 0.01–1.00 mD, and the pressure coefficient of the seven# sand body is only 0.47, belonging to the normal temperature and low pressure tight sandstone gas reservoir (Zheng et al., 2021).
Select a sandstone core that is underground that has a complete exterior and no visible fractures. Drill, cut, and polish the two ends with an end grinder to make sure they are parallel and smooth. The core measures 15 mm in length and 25 mm in diameter, As shown in Figure 2. The end face of the core sample must be vertical to the circumference and the maximum deviation must not be greater than 0.05 mm. The height and diameter errors of the core sample must not be greater than 0.3 mm. The core must be processed in accordance with the standards of the International Society of Rock Mechanics (ISRM) to make the sample error within the following range. The sandstone sample must be wrapped and covered with fresh-keeping film after preparation in order to shield it from air reaction during following studies.
As shown in Figure 3, the visualization apparatus for the interaction of supercritical CO2 with rock consists of a CO2 gas cylinder, a CO2 storage tank, a cold bath, a CO2 booster pump, a visual reactor, a heating jacket, and control systems. Visual reactor is a crucial part of the experimental system. As seen in Figure 3, the sapphire glass window in the visual reactor allows for real-time observation of the interaction process between supercritical CO2 and rock. The visual reactor has a volume of 500 ml, the CO2 storage tank has a volume of 5 L, the temperature range is −5°C–150°C, and the pressure bearing capability of the entire unit is 50 MPa. The flow chart of the experimental device is shown in Figure 4. By using the control system and changing the settings of the heating jacket and CO2 booster pump, the liquid CO2 is converted to supercritical CO2, as shown in Figure 5, which can be used for the interaction process between supercritical CO2 and rock at different temperatures, pressures and times.
The scanning electron microscopy apparatus is represented in Figure 6, and it is primarily made up of an electron microscope, an electron gun filament, a vacuum system, and an automated system for acquiring and processing samples and images. With a maximum magnification of ×150000 and a resolution higher than 10 nm, it can swiftly produce images with rich surface features that can be used to measure samples that are smaller than a micron or even smaller than a nanometer in size. It is used to research how supercritical CO2 interacts with rock and to track trends in reservoir physical qualities like permeability and porosity.
Figure 7 shows an X-ray diffractometer. A high stability X-ray source, a sample and sample position orientation adjustment mechanism system, a ray detector, and a system for processing and analyzing diffraction patterns make up its core parts. Equipment specifications: A common size light tube is used as the Cu target, and a theta/theta vertical goniometer is used. The theta angle range is 2–160, and the angle accuracy is 0.0001. The X-ray needed for measurement is produced by the high stability X-ray source. The X-ray tube’s anode target material can alter the wavelength of the radiation. The intensity of the X-ray source can be changed by adjusting the anode voltage. This allows for routine phase analysis and semi-quantitative analysis of polycrystalline powder, block, and liquid samples, as well as the determination and correction of unit cell parameters, the X-ray diffraction indexation of unidentified polycrystalline samples, and the determination of grain size and crystallinity.
The flow compatibilizer, high-pressure infusion pump, sampler, chromatographic column, detector, and data processing system are the key components of the ion chromatograph, as shown in Figure 8. The chromatographic pump has a maximum operating pressure of 50 MPa, a flow rate of 0.001–20 ml/min, a minimum graduation value of 0.001 ml/min, a concentration range of 0–100% for the ion chromatograph’s eluent, an effective injection volume of 0.5–11 ml, and a measurement range of 0–15000 us/cm without section switching. The plasma concentrations of K+, Na+, Ca2+, Mg2+, Cl−, F−, and SO42− in aqueous solution are used in a liquid chromatography method for separation and detection that uses the ionic properties of the tested substances. This method is a powerful reference for the variation characteristics and laws of ion concentration in liquid.
It is required to examine and identify changes in the microscopic properties, mineral composition, and ion concentration of the solution of sandstone samples before and after the experiment in order to research the mechanism of interaction between supercritical CO2 and sandstone. The steps of the experiment are as follows:
1) In order to accurately obtain the change of core weight, sandstone samples should be cleaned with distilled water to remove surface impurities, and then dried and weighed.
2) Assemble the visualization tool for the interaction between supercritical CO2 and rock and wait until it is ready for usage. It is used to carry out the interaction process between supercritical CO2 and tight sandstone.
3) Place the formation water-prepared container into the visualization device for the interaction between supercritical CO2 and rock, then insert it into the sandstone core. Next, start the booster pump to inject CO2 for pressurization. Then, set the pressurization temperature and time according to different experiments. Finally, determine the sampling times according to the time. Take a core and 20 ml of liquid each time, and inspect and analyze the core with scanning electron microscopy.
4) After the reaction, allow the reactor to cool to ambient temperature before opening it to remove the sample. The sandstone sample should then be cleaned with distilled water, dried, and weighed in an oven.
5) Following the aforementioned treatment, the core was examined and evaluated using a scanning electron microscope and an X-ray diffractometer; an ion chromatograph was used to ascertain the composition of the reaction solution. The interaction mechanism between supercritical CO2 and tight sandstone is analyzed.
When supercritical CO2 comes into contact with sandstone at the proper temperature and pressure, it first diffuses into the rock aquifer’s pores, interacts with formation water to form a weak acidic fluid, and then reacts with the rocks to produce a series of complicated chemical reactions that lead to the decomposition of brittle minerals like carbonate and feldspar in the sandstone and the precipitation of new minerals, altering the microstructure, weight, porosity, and permeability of sandstone.
The fluid changes into a weak acidic fluid as a result of CO2 first coming into touch with the water in the pores, followed by it dissolving in the water at the water-air interface and reacting with it to generate carbonic acid (Bierg and Banwart, 2000).The reaction formula is:
Carbonic acid quickly breaks down into bicarbonate ions:
The released hydrogen ions will result in the complexation of dissolved cations with bicarbonate ions and the disintegration of carbonate minerals and silicates in tight sandstone rocks, including:
Finally, the dissolved bicarbonate combines with cations to produce additional carbonate minerals such as calcite, magnesite, siderite, and dawsonite:
Therefore, soluble minerals in the rock are dissolved after CO2 and tight sandstone come into contact, and new minerals are also generated, modifying the sandstone.
According to the formation conditions and the characteristic time stage after simulated fracturing treatment. Tight sandstone and supercritical CO2 reaction for 48, 168, and 360 h, respectively, at 60°C under a test pressure of 10 MPa, the temperature is the formation temperature, the pressure is the difference between the minimum principal stress of the formation and the formation pore pressure, and the action time is selected as several characteristic time points after fracturing treatment. The samples of sandstone are weighed after the reaction. Table 1 shows the test results. The weight of the rocks decreases after the test. After supercritical CO2 dissolves in water, the hydrogen ions decomposed by weak acidic fluid dissolve potassium feldspar, plagioclase and some clay minerals, resulting in rock weight changes. The weight of the tight sandstone samples reduces by 1.91% after 48 h, 2.88% after 168 h, and 4.85% after 360 h. More weight loss over time suggests that the supercritical CO2 and rock primarily have a certain dissolving response during the experiment, and that the degree of dissolution gradually increases as time goes on.
Tight sandstone and supercritical CO2 reacted at 60°C for 48 h, 168 h, and 360 h, respectively, under an experimental pressure of 10 MPa. After the reaction, sandstone samples were examined under a scanning electron microscope to reveal that after 48 h, the feldspar was only mildly corroded while the quartz and clay minerals remained comparatively stable, generating a few corrosion pits, as seen in Figure 9. As depicted in Figure 10, after 168 h of reaction, the degree of feldspar dissolution increased, quartz and clay minerals also started to undergo weak dissolution, and some deep holes developed on the visible surface; After 360 h of reaction, the feldspar’s degree of dissolution increased further, and quartz and clay minerals started to dissolve somewhat as well, resulting in a broken dissolution phenomena, as illustrated in Figure 11.
When CO2 is in a supercritical state, it reacts with tight sandstone. It initially diffuses into the tight sandstone aquifer pores at formation temperature and pressure, reacts with formation water to form weak acidic fluid, and then interacts with rocks to produce a series of intricate chemical processes.
The mineral compositions of sandstone before and after supercritical CO2 and tight sandstone were qualitatively and quantitatively examined by XRD in order to further characterize the CO2 water rock interaction mechanism.
In Figure 12 and Table 2, the results of the analysis and interpretation of the relative content test of mineral components of tight sandstone samples prior to the action of supercritical CO2 are displayed. The examination of sandstone composition shows that Jinqiu tight sandstone has the highest concentrations of quartz and plagioclase, at 54.2% and 34.5%, respectively.
The tight sandstone reacts with supercritical CO2 after 48 h, 168 h, and 360 h at 60°C under an experimental pressure of 10 MPa. The sandstone samples are analyzed by X-ray diffraction after the reaction. In Figure 13, the experimental results are displayed.
FIGURE 13. Mineral composition of tight sandstone changes after various supercritical CO2 reaction times.
Following the experiment, the sandstone’s material composition was compared and examined. It can be seen that after 48 h of reaction with supercritical CO2, the quartz content of Jinqiu tight sandstone increased by 1.5%, the potassium feldspar content decreased by 0.2%, the plagioclase content decreased by 1.2%, and the clay minerals content decreased by 0.4%. 0.3% of newly formed calcite was discovered at the same time. After 168 h of reaction, quartz’s content increased by 3.4%, potassium feldspar’s content dropped by 0.7%, plagioclase’s content dropped by 2.8%, the content of clay minerals dropped by 0.6%, and the content of newly formed calcite increased to 0.7%. After 360 h of reaction, there was a 5.3% increase in quartz content, a 1.1% decrease in potassium feldspar content, a 4.3% drop in plagioclase content, a 1.0% decrease in clay mineral content, and a 1.0% increase in newly created calcite content.
Generally speaking, when supercritical CO2 interacts with Jinqiu tight sandstone, quartz content rises, potassium feldspar and plagioclase content fall, and new calcite minerals form. Thus, it is clear how the ionic reaction Ca2+ + CO32- ⇋ CaCO3 (calcite) in the solution and the deposition of the resulting calcite on the sample surface occur during the experiment as a result of the interaction between CO2 and the sandstone samples. It is possible to implement the underground storage of CO2 using material traps, as evidenced by the development of calcite, which shows that CO2 can be stored in the solution as insoluble carbonate and precipitated as calcite and other CO2 capture minerals.
Feldspar, quartz, and other soluble minerals in the reservoir sandstone can dissolve in the acidic fluid created when CO2 dissolves in water, creating new carbonate minerals. The formation temperature and pressure of the reservoir are simulated in accordance with the detection results of the formation water quality of the reservoir in order to further study the dissolution of feldspar, quartz, clay minerals, and other soluble minerals, combined with the change of ion concentration in the reaction solution. The production of CO2 fluid and the interaction of water and sandstone are further discussed.
After completely soaking the dense sandstone samples, Table 3 displays the formation water data of the sha1-2 sub member of the Shaximiao Formation in the Jinqiu gas field. CaCl2 type formation water is used. According to the salt formed by the final combination of Na+, Cl− and other ions, the name of this kind of salt is water type. It mainly includes NaHCO3 type, Na2SO4 type, MgCl2 type and CaCl2 type. CaCl2 type formation water represents the water formed under the deep closed structural environment, with good sealing property, which is conducive to the accumulation and preservation of oil and gas, and is a sign of good oil and gas.
The tight sandstone reacts with supercritical CO2 over 48 h, 168 h, and 360 h at 60°C under a test pressure of 10 MPa. Following the reaction, the water quality of the solution containing the samples of sandstone is examined and evaluated. Figure 14 displays the examination results.
FIGURE 14. Change of mineral composition of after different action time of sandstone and supercritical CO2.
In cations, the mass concentrations of K+, Na+, Ca2+ increased with reaction time. K+ mainly came from the dissolution of potassium feldspar, and the K+ concentration increased from 5,133 mg/L to 5,339 mg/L, an increase of 206 mg/L. Na+ and Ca2+ are mainly from the dissolution of plagioclase, indicating that the dissolution degree of potassium feldspar and plagioclase is gradually increasing. The concentration of Na+ increases from 6,227 mg/L to 6,401 mg/L, an increase of 174 mg/L, and the concentration of Ca2+ increases from 18,000 mg/L to 18,200 mg/L, an increase of 200 mg/L. The mass concentration of Mg2+ and Ba2+ changed slightly with time. The concentration of Mg2+ increased from 233 mg/L to 272 mg/L, increased by 39 mg/L, the concentration of Ba2+ increased from 1815 mg/L to 1821 mg/L, increased by 6 mg/L, and Al3+ also increased from nothing, but the content was low. Al3+ concentration increased from 0 mg/L to 12 mg/L, an increase of 12 mg/L.
In anions, a small amount of clay minerals dissolved, resulting in a slow increase in anionic Cl− concentration, which increased from 51,323 mg/L to 51,569 mg/L, an increase of 246 mg/L. The mass concentration of SO42- changed little, and the concentration of SO42- increased from 5 mg/L to 12 mg/L, an increase of 7 mg/L. The concentrations of HCO3− and CO32- in the solution first increased rapidly, and then the growth rate gradually decreased. HCO3− concentration increased from 123 mg/L to 733 mg/L, an increase of 610 mg/L. The concentration of CO32− increased from 0 mg/L to 69 mg/L, an increase of 69 mg/L. The two reactions H2CO3 = H+ + HCO3− and HCO3− = H+ + CO32− can be used to illustrate the changing process. The CO32− is consumed by the reaction equation (HCO3− = H+ + CO32−), which causes the divalent cations dispersed in the solution and CO32− to form insoluble carbonate. As the minerals dissolve, the divalent cations dissolve and produce carbonate precipitation, which slows the pace at which HCO3− and CO32− concentrations develop in the solution.
1) Supercritical CO2 is a new type of anhydrous fracturing fluid that can achieve in-situ storage of greenhouse gases and has a wide range of potential applications in low-pressure tight sandstone gas reservoirs. It differs from conventional water-based fracturing fluid in that it can achieve in-situ storage of greenhouse gases.
2) In this study, tight sandstone reacts with supercritical CO2 after 360 h at 60°C under pressure of 10 MPa, there was a 5.3% increase in quartz content, a 1.1% decrease in potassium feldspar content, a 4.3% drop in plagioclase content, a 1.0% decrease in clay mineral content, and a 1.0% increase in newly created calcite content. Time increases the degree to which sandstone samples dissolve, and CO2 can be tightly trapped in sandstone as carbonate minerals.
3) After the tight sandstone reacts with supercritical CO2. In cations, the mass concentrations of K+, Na+, Ca2+ increased with reaction time, indicating that the dissolution degree of potassium feldspar and plagioclase is gradually increasing. The mass concentration of Mg2+ and Ba2+ changed slightly with time. In anions, a small amount of clay minerals dissolved, resulting in a slow increase in anionic Cl− concentration. The mass concentration of SO42- changed little. The concentrations of HCO3− and CO32- in the solution first increased rapidly, and then the growth rate gradually decreased.The interaction mechanism between supercritical CO2 and sandstone is very important for understanding the feasibility, long-term and safety of CO2 underground storage and supercritical CO2 fracturing.
The original contributions presented in the study are included in the article/Supplementary Material, further inquiries can be directed to the corresponding author.
HP is responsible for the overall structure and revision. JY, JLP, JHP, and QL help evaluate and improve the paper. JS and JL help in setting up the device test, writing, and the experiment.
The authors declare that this study received funding from Southwest Oil and Gas Field Company, PetroChina. The funder was not involved in the study design, collection, analysis, interpretation of data, the writing of this article or the decision to submit it for publication.
Authors HP, JY, JLP and JS were employed by Engineering Technology Research Institute of Southwest Oil & Gas Field Company, PetroChina and Key Laboratory of Oil & Gas Well Stimulation Technology of Southwest Oil & Gas Field Company JHP and QL were employed by Southwest Oil & Gas Field Company, PetroChina. JL was employed by No. 8 Oil Extraction Plant of Changqing Oilfield Company, PetroChina.
All claims expressed in this article are solely those of the authors and do not necessarily represent those of their affiliated organizations, or those of the publisher, the editors and the reviewers. Any product that may be evaluated in this article, or claim that may be made by its manufacturer, is not guaranteed or endorsed by the publisher.
Ao, X., Lu, Y., Tang, J., Chen, Y., and Li, H. (2017). Investigation on the physics structure and chemical properties of the shale treated by supercritical CO2. J. CO2 Util. 20, 274–281. doi:10.1016/j.jcou.2017.05.028
Bachu, S. (2016). Identification of oil reservoirs suitable for CO2-EOR and CO2 storage (CCUS) using reserves databases, with application to Alberta, Canada. Int. J. Greenh. Gas Control 44, 152–165. doi:10.1016/j.ijggc.2015.11.013
Berg, A., and Banwart, S. A. (2000). Carbon dioxide mediated dissolution of Ca-feldspar: Implications for silicate weathering. Chem. Geol. 163 (1-4), 25–42. doi:10.1016/s0009-2541(99)00132-1
Cao, Y., Zhang, J., Zhai, H., Fu, G., Tian, L., and Liu, S. (2017). CO2 gas fracturing: A novel reservoir stimulation technology in low permeability gassy coal seams. Fuel 203, 197–207. doi:10.1016/j.fuel.2017.04.053
Chen, K., Liu, X., Nie, B., Zhang, C., Song, D., Wang, L., et al. (2022). Mineral dissolution and pore alteration of coal induced by interactions with supercritical CO2. Energy 248, 123627. doi:10.1016/j.energy.2022.123627
Day, S., Fry, R., and Sakurovs, R. (2008). Swelling of Australian coals in supercritical CO2. Int. J. Coal Geol. 74 (1), 41–52. doi:10.1016/j.coal.2007.09.006
Gathitu, B. B., Chen, W. Y., and McClure, M. (2009). Effects of coal interaction with supercritical CO2: Physical structure. Ind. Eng. Chem. Res. 48 (10), 5024–5034. doi:10.1021/ie9000162
Grainger, A., and Smith, G. (2021). The role of low carbon and high carbon materials in carbon neutrality science and carbon economics. Curr. Opin. Environ. Sustain. 49, 164–189. doi:10.1016/j.cosust.2021.06.006
Hasan, M. F., First, E. L., Boukouvala, F., and Floudas, C. A. (2015). A multi-scale framework for CO2 capture, utilization, and sequestration: CCUS and CCU. Comput. Chem. Eng. 81, 2–21. doi:10.1016/j.compchemeng.2015.04.034
Hazarika, S., and Boruah, A. (2022). Supercritical CO2 (SCO2) as alternative to water for shale reservoir fracturing. Mater. Today Proc. 50, 1754–1757. doi:10.1016/j.matpr.2021.09.187
Jia, Y., Lu, Y., Elsworth, D., Fang, Y., and Tang, J. (2018). Surface characteristics and permeability enhancement of shale fractures due to water and supercritical carbon dioxide fracturing. J. Petroleum Sci. Eng. 165, 284–297. doi:10.1016/j.petrol.2018.02.018
Jiang, Y., Luo, Y., Lu, Y., Qin, C., and Liu, H. (2016). Effects of supercritical CO2 treatment time, pressure, and temperature on microstructure of shale. Energy 97, 173–181. doi:10.1016/j.energy.2015.12.124
Lin, H., Fujii, T., Takisawa, R., Takahashi, T., and Hashida, T. (2008). Experimental evaluation of interactions in supercritical CO2/water/rock minerals system under geologic CO2 sequestration conditions. J. Mat. Sci. 43 (7), 2307–2315. doi:10.1007/s10853-007-2029-4
Lin, R., Yu, Z., Zhao, J., Dai, C., Sun, Y., Ren, L., et al. (2022). Experimental evaluation of tight sandstones reservoir flow characteristics under CO2–brine–rock multiphase interactions: A case study in the chang 6 layer, ordos basin, China. Fuel 309, 122167. doi:10.1016/j.fuel.2021.122167
Liu, H., Wang, F., Zhang, J., Meng, S., and Duan, Y. (2014). Fracturing with carbon dioxide: Application status and development trend. Petroleum Explor. Dev. 41 (4), 513–519. doi:10.1016/s1876-3804(14)60060-4
Memon, S., Feng, R., Ali, M., Bhatti, M. A., Giwelli, A., Keshavarz, A., et al. (2022). Supercritical CO2-Shale interaction induced natural fracture closure: Implications for scCO2 hydraulic fracturing in shales. Fuel 313, 122682. doi:10.1016/j.fuel.2021.122682
Mouahid, A., Claeys-Bruno, M., Bombarda, I., Amat, S., Ciavarella, A., Myotte, E., et al. (2022). Supercritical CO2 extraction of oil from Moroccan unroasted Argan Kernels: Effects of process parameters to produce cosmetic oil. J. CO2 Util. 59, 101952. doi:10.1016/j.jcou.2022.101952
Nikolai, P., Rabiyat, B., Aslan, A., and Ilmutdin, A. (2019). Supercritical CO2: Properties and technological applications-A review. J. Therm. Sci. 28 (3), 394–430. doi:10.1007/s11630-019-1118-4
Peng, Y., Li, Y., and Zhao, J. (2016). A novel approach to simulate the stress and displacement fields induced by hydraulic fractures under arbitrarily distributed inner pressure. J. Nat. Gas Sci. Eng. 35, 1079–1087. doi:10.1016/j.jngse.2016.09.054
Peng, Y., Zhao, J., Sepehrnoori, K., Li, Y., and Li, Z. (2020). The influences of stress level, temperature, and water content on the fitted fractional orders of geomaterials. Mech. Time. Depend. Mat. 24 (2), 221–232. doi:10.1007/s11043-019-09417-0
Peng, Y., Zhao, J., Sepehrnoori, K., Li, Z., and Xu, F. (2019). Study of delayed creep fracture initiation and propagation based on semi-analytical fractional model. Appl. Math. Model. 72, 700–715. doi:10.1016/j.apm.2019.03.034
Tapia, J. F. D., Lee, J. Y., Ooi, R. E., Foo, D. C., and Tan, R. R. (2018). A review of optimization and decision-making models for the planning of CO2 capture, utilization and storage (CCUS) systems. Sustain. Prod. Consum. 13, 1–15. doi:10.1016/j.spc.2017.10.001
Umar, M., Farid, S., and Naeem, M. A. (2022). Time-frequency connectedness among clean-energy stocks and fossil fuel markets: Comparison between financial, oil and pandemic crisis. Energy 240, 122702. doi:10.1016/j.energy.2021.122702
Wang, J., Elsworth, D., Wu, Y., Liu, J., Zhu, W., and Liu, Y. (2018). The influence of fracturing fluids on fracturing processes: A comparison between water, oil and SC-CO2. Rock Mech. Rock Eng. 51 (1), 299–313. doi:10.1007/s00603-017-1326-8
Wang, N., Akimoto, K., and Nemet, G. F. (2021). What went wrong? Learning from three decades of carbon capture, utilization and sequestration (CCUS) pilot and demonstration projects. Energy Policy 158, 112546. doi:10.1016/j.enpol.2021.112546
Yue, P., Zhang, R., Sheng, J. J., Yu, G., and Liu, F. (2022). Study on the influential factors of CO2 storage in low permeability reservoir. Energies 15 (1), 344. doi:10.3390/en15010344
Zhang, B. J., Pan, K., and Wu, C. J. (2022). Compound gas accumulation mechanism and model of jurassic Shaximiao formation multistage sandstone formations in Jinqiu gas field of the Sichuan Basin. Nat. Gas. Ind. 42 (1), 51–61. doi:10.3787/j.issn.1000-0976.2022.01.005
Zhang, D. W., and Yang, Y. (2022). Exploration potential and development direction of continental tight sandstone gas in the Sichuan Basin[J]. Nat. Gas. Ind. 42 (1), 1–11. doi:10.3787/j.issn.1000-0976.2022.01.001
Zhao, J., Peng, Y., Li, Y., and Tian, Z. (2017). Applicable conditions and analytical corrections of plane strain assumption in the simulation of hydraulic fracturing. Petroleum Explor. Dev. 44 (3), 454–461. doi:10.1016/s1876-3804(17)30052-6
Zhao, X., Ma, X., Chen, B., Shang, Y., and Song, M. (2022). Challenges toward carbon neutrality in China: Strategies and countermeasures. Resour. Conservation Recycl. 176, 105959. doi:10.1016/j.resconrec.2021.105959
Zheng, Y., Han, X., Zeng, J., Zhou, C., Zhou, L., and Chen, W. (2021). Practice of high-intensity volume fracturing in the Shaximiao Formation tight sandstone gas reservoirs of the Qiulin Block, central Sichuan Basin. Nat. Gas. Ind. B 8 (4), 367–375. doi:10.1016/j.ngib.2021.07.007
Zhou, D., Zhang, G., Prasad, M., and Wang, P. (2019). The effects of temperature on supercritical CO2 induced fracture: An experimental study. Fuel 247, 126–134. doi:10.1016/j.fuel.2019.02.099
Zhou, J., Xie, S., Jiang, Y., Xian, X., Liu, Q., Lu, Z., et al. (2018). Influence of supercritical CO2 exposure on CH4 and CO2 adsorption behaviors of shale: Implications for CO2 sequestration. Energy fuels. 32 (5), 6073–6089. doi:10.1021/acs.energyfuels.8b00551
Keywords: supercritical CO2, tight sandstone, interaction mechanism, CCUS, fracturing technology, experimental evaluation
Citation: Peng H, Yang J, Peng J, Pu J, Liu Q, Su J and Liu J (2022) Experimental investigation of the mechanism of supercritical CO2 interaction with tight sandstone. Front. Energy Res. 10:984144. doi: 10.3389/fenrg.2022.984144
Received: 01 July 2022; Accepted: 25 July 2022;
Published: 24 August 2022.
Edited by:
Yibo Li, Southwest Petroleum University, ChinaReviewed by:
Jiaqi Wang, Harbin Engineering University, ChinaCopyright © 2022 Peng, Yang, Peng, Pu, Liu, Su and Liu. This is an open-access article distributed under the terms of the Creative Commons Attribution License (CC BY). The use, distribution or reproduction in other forums is permitted, provided the original author(s) and the copyright owner(s) are credited and that the original publication in this journal is cited, in accordance with accepted academic practice. No use, distribution or reproduction is permitted which does not comply with these terms.
*Correspondence: Huan Peng, MjgyNjAwMTIwQHFxLmNvbQ==
Disclaimer: All claims expressed in this article are solely those of the authors and do not necessarily represent those of their affiliated organizations, or those of the publisher, the editors and the reviewers. Any product that may be evaluated in this article or claim that may be made by its manufacturer is not guaranteed or endorsed by the publisher.
Research integrity at Frontiers
Learn more about the work of our research integrity team to safeguard the quality of each article we publish.