- 1Thermo Fisher Scientific, Alachua, FL, United States
- 2New Mexico Consortium, Los Alamos, NM, United States
There is growing evidence that eukaryotic microalgae can become a more sustainable and profitable alternative than terrestrial crops to produce feed, fuels, and valuable coproducts. The major factor driving progress in algal biomass production is the potential of microalgae to produce substantially greater biomass per unit land area than terrestrial crops. To be financially feasible, however, current algal biomass yields must be increased. Given the fact that algal biomass production is in its infancy there exist multiple opportunities to improve biomass yields. For example, recent bioprospecting efforts have led to the identification of new microalgal strains having biomass yields that compete economically with plant biomass. Substantial increases in biomass yields have also been achieved using advanced genetic engineering approaches. Targeted improvements in photosynthetic efficiency have led to three-fold increases in algal biomass yields. One genetic tool that has seen limited application for algal biomass enhancement is advanced breeding genetics. The greater availability of algal genomes and recent advancements in breeding algae will further accelerate yield improvements. Genetic engineering strategies to increase biomass production will also be assisted by transcriptomic and metabolomic studies that help identify metabolic constraints that limit biomass production. In this review we assess some of the recent advances in algal strain selection, directed evolution, genetic engineering and molecular-assisted breeding that offer the potential for increased algal biomass production.
Highlights
• Based on thermodynamic considerations five-fold increases in algal biomass production are theoretically feasible
• Enhanced and stable algal biomass production strains have recently been identified that achieve biomass yields approaching those necessary for economical bioproduct production
• Molecular assisted breeding of algae has been shown to enhance biomass yields more than three fold
• Engineering the photosynthetic apparatus of algae has lead to three-fold increases in biomass production
• Future strategies for enhancing biomass production in algae are considered
Introduction
Among the major constraints limiting the commercialization of eukaryotic microalgae for biomass production are the high costs of cultivation, harvesting and processing. Relative to crop plants algal biomass production requires substantial capital and operating investments including lined ponds, pond aeration and mixing systems, and energy intensive algal harvesting and processing systems (Unkefer et al., 2017; Benedetti et al., 2018; Sun et al., 2018; Rajvanshi and Sayre, 2020). The high operating costs for algal biomass can largely be attributed to the fact that algal biomass concentration is only 0.1%–0.5% of the cultivation media substantial volumes of water must be managed to grow and harvest algae requiring specialized infrastructure and high energy costs that could exceed the energy content of the algal biomass. Offsetting these extensive cost factors, however, is the high growth rate of algae. High algal growth rates are associated with their potential to grow throughout the year, the absence of heterotrophic tissues not engaged in biomass production, and the presence of high efficiency photosynthetic systems including active CO2 concentrating systems that reduce photorespiratory carbon losses (Sayre, 2010; Wang et al., 2011; Subramanian et al., 2013). As a result, algae have the potential to produce as much as 5-fold more biomass per unit land area than terrestrial crop plants (Weyer et al., 2010; Subramanian et al., 2013). Consequently, there has been incredible interest to develop microalgae for low-cost production of feeds, fuels, high value bioproducts and to sequester atmospheric carbon dioxide to mitigate climate change (Olivares et al., 2016; Lammers et al., 2017; Unkefer et al., 2017; Marrone et al., 2018; Sayre, 2010). However, there remain multiple constraints that limit the commercialization of eukaryotic microalgae. Life cycle and techno-economic analyses of algal cultivation systems have indicated that only open-pond production systems can economically produce algal biomass that is cost competitive with crops (Chisti, 2013; Davis et al., 2014; Barry et al., 2016). But to be economically feasible, outdoor open-pond cultivation systems must produce stable biomass yields approaching 40 gdw/m2/day, corresponding to an overall solar to biomass conversion efficiency of ∼3% (Bolton and Hall, 1991; Kruse et al., 2005a; Kruse et al., 2005b; Zhu et al., 2008; Lammers et al., 2017). Given that the maximum theoretical efficiency for solar energy conversion into the chemical energy of algal biomass by photosynthesis is approximately 11% there is the potential to achieve 2- to 3-fold increases in biomass yields (Bolton and Hall, 1991; Kruse et al., 2005a; Kruse et al., 2005b; Zhu et al., 2008; Weyer et al., 2010). Thus, substantial improvements in biomass accumulation are theoretically possible.
When considering strategies to increase algae biomass yields it is informative to consider genetic and agronomic practices that have been successfully used to increase biomass production in terrestrial crops. Over the last 75 years great strides have been achieved in the development of higher yielding and more robust crops. These achievements have largely been the result of advanced agronomic practices to better manage resource inputs and the application of modern agricultural breeding techniques to improve crop genetics. Crop breeding strategies were initially based on the application of genetic linkage analyses between traits of interest and more tractable or scorable traits. In the last 40 years molecular assisted breeding and genomic selection techniques have further accelerated the development of improved crops. The application of modern breeding systems including, the use of inbred lines, hybrid sterility mechanisms, and transgenic modifications, has led to over a 7-fold increase in maize yields between 1940 and 2015 (168 bu/acre). Most of these improvements in crop yield have been achieved through the development of herbicide resistance genes, and the introduction of pest, pathogen and stress tolerance traits. Surprisingly, few projects focusing enhanced carbon capture for biomass yield improvements have reached the stage of commercial deployment.
In contrast to terrestrial crops, the greatest enhancements in microalgal biomass yields have been realized through the identification of more robust algal species and through directed selection and transgenic approaches rather than through traditional breeding approaches (Kumar et al., 2013; Kumar et al., 2016; Crozet et al., 2018; Fayyaz et al., 2020; Dementyeva et al., 2021; Mosey et al., 2021). In the following sections we address why that is the case and review recent advances that have led to further improvements in eukaryotic microalgal biomass production.
Strain selection for enhanced biomass yield
The emerging consensus is that the most economically feasible means to produce algal biomass is to cultivate algae in continuously mixed, open pond culture systems (Chisti, 2013; Davis et al., 2014; Barry et al., 2016; Lammers et al., 2017; Marrone et al., 2018). These pond systems typically range in depth from 15 to 30 cm. While greater algal biomass yields per unit area are possible in closed photobioreactor (PBR) systems these systems have several inherent disadvantages when operated at large scale including, greater capital and operating expenses than open pond systems, challenges associated with the formation of biofilms that reduce light penetration and the management of pathogens or herbivores (Chisti, 2013; Davis et al., 2014; Barry et al., 2016; Lammers et al., 2017; Marrone et al., 2018; Rajvanshi and Sayre, 2020). Overall PBR-based algal biomass production systems are estimated to be 2- to 2.5-fold more expensive to operate than open pond production systems (Chisti, 2013; Davis et al., 2014; Barry et al., 2016). Due to their open nature algal species that are cultivated in ponds must have fast growth rates to out compete weedy invasive species and be resistant to biotic and abiotic stressors including large temperature and light intensity shifts, limiting daylength periods at high latitudes, precipitation, competing weedy algal species, pathogens, and herbivores (Barry et al., 2016; Neofotis et al., 2016; Olivares et al., 2016; Marrone et al., 2018; Rajvanshi and Sayre, 2020). As a result of these challenges substantial efforts have gone into screening or bioprospecting for algal strains that not only have high growth rates but that can also tolerate abiotic or biological stressors that lead to pond crashes. Given that there are over 164,000 known species of algae bioprospecting for high biomass producing algal strains particularly those best suited for the local environments where they are cultivated continues to provide opportunities for enhancing algal biomass yields (Marrone et al., 2018; Guiry and Guiry, 2021).
Since the mid-1970s, the United States Department of Energy has supported multiple algae bioprospecting efforts to identify high biomass yielding and stable strains suitable for commercial biomass production (Weissman and Goebel, 1987; Barry et al., 2016; Olivares et al., 2016). To date, algal strains having the highest and most stable biomass production rates are dominated by species from two phyla, the Chlorophyta and Ochrophyta. These include species from the genera, Chlorella, Picochlorum, Scenedesmus, Cyclotela, Tetraselmus and Nanochloropsis (Table 1). Many of these species have demonstrated long-term biomass production (>100 days) yields approaching 40 gdw/m2/day 22, (Huesemann et al., 2018; Dahlin et al., 2019; Gonzalez-Esquer et al., 2019; Aketo et al., 2020; Mucko et al., 2020; Cano et al., 2021; Krishnan et al., 2021). Averaged over a yearlong growing season algae having a growth rate of 40 gdw/m2/day could yield as much as 145 metric tons dw/ha/yr having an average energy density of 21 kJ/gdw. In comparison, corn yields in the United States Midwest average 11.5 metric tons dw/ha/yr having an average energy density of 16.7 kJ/gdw. Thus, the annual areal energy yield for algal biomass grown at a rate of 40 gdw/m2/day is 16-fold greater than for corn. Given that the corn growing season is only 3 months, however, the relative efficiency of algal biomass production is about 4-fold greater than for corn on an annualized basis.
Assessing relative biomass yields between strains and treatments can be challenging due to the lack of standardized practices for measuring biomass yields or net productivity. Algal biomass yields may be quantified based on optical density, cell numbers per unit volume, dry weight per unit volume and dry weight yield per unit land area. Furthermore, since algae are grown under a variety of environmental conditions it can be challenging to assess relative yields between different studies. Given that solar energy inputs are based on the photon flux density per unit area it is useful to assess biomass yields based on areal productivity per unit time (gdw/m2/day) so that energy conversion efficiencies can be directly assessed.
Another important consideration in choosing algal strains for biomass production is the biochemical composition (lipid, carbohydrate and protein makeup) of the algae. Many algae species can also facultatively adjust their relative lipid and carbohydrate levels depending on culture conditions (Boussiba et al., 1987; Negi et al., 2016; Sarwer et al., 2022). In a metanalysis of the biomass composition of the Chlorophyta and Ochrophyta it was determined that the Chlorophytes had on average 33% protein, 16% lipid, 14% carbohydrate and 12% ash content per unit dry weight. In contrast, the Ochrophytes had 33% protein, 21% lipid, 14% carbohydrate, and 19% ash content by dry weight. Therefore, the ratio of carbohydrate to lipid (0.9) was greater for the Chlorophytes than for the Ochrophytes (0.5) (Finkel et al., 2016). Thus, the Ochrophytes were on average more energy dense than the Chlorophytes.
Recently, one genus of algae, Picochlorum, has emerged as one of the highest and most stable biomass producers (Dahlin et al., 2019; Gonzalez-Esquer et al., 2019; Mucko et al., 2020; Cano et al., 2021; Krishnan et al., 2021). Picochlorum are marine algae having a wide global distribution that tolerate high salinity and temperatures (40 C) well allowing for cultivation in a broad range of environments (Zhu et al., 2008; Dahlin et al., 2019; Krishnan et al., 2021). Furthermore, recent studies have indicated that some Picochlorum species have greater photosynthetic efficiency than many algal species (Cano et al., 2021). This enhanced efficiency is associated with ability to self-adjust their light harvesting antenna size to optimize light use efficiency in dynamically changing light environments (Perrine et al., 2012; Negi et al., 2020; Cano et al., 2021). In addition, P. celeri has been shown to be able to both adjust its light harvesting antenna size as well as accumulate high levels of photoprotective carotenoids involved in non-photochemical quenching of excess radiation (Cano et al., 2021). Recently, molecular toolboxes for the genetic manipulation of P. celeri have been developed including genetic transformation (transformation systems and the development of selectable marker genes) and genome editing tools (Cas9) allowing for additional engineering opportunities to improve biomass yields.
Thermodynamic modeling of the energy efficiency of converting solar photons into chemical energy of biomass in algae indicates that the type of major energy product stored has an impact on the overall solar to chemical energy conversion efficiency. Storage of reduced carbon as starch has been shown to be more energy efficient than lipid storage (Subramanian et al., 2013; Finkel et al., 2016; Sun et al., 2018; Aketo et al., 2020; Changko et al., 2020; Negi et al., 2020). The greater energy storage efficiency of starch versus lipids is largely due to the loss of previously reduced carbon during lipid synthesis that occurs with the decarboxylation of pyruvate to produce acetyl CoA for lipid synthesis. This loss of previously fixed carbon does not occur during starch accumulation. It follows to reason that screening techniques for the selection of high biomass production strains efforts should focus on high starch content strains rather than high lipid strains.
Recently, a green algal species Pseudoneochloris sp. Strain NKY372003 was shown to accumulate very high starch levels relative to other algal strains (68% of total biomass) (Aketo et al., 2020). In addition, Pseudoneochloris has the ability to increase its cell volume by over 100-fold. In comparative growth studies, the volumetric biomass yield (8.1 gdw/L) of Pseudoneochloris was shown to be 47% greater than for Tetraselmis (5.5 gdw/L) one of the higher known areal biomass producers (37 gdw/m2/day) (Table 1). These results suggest that alterations in the allocation of fixed carbon between starch and lipid storage could substantially impact algal biomass yields. Given the fact that starch has a substantially higher mass density (1.54 g/cm3) than lipids (0.91 g/cm3), simple sedimentation or isopycnic centrifugation selection systems could be used to efficiently bioprospect for high starch/high biomass strains. Due to the greater interest in algal lipids for biofuel production, however, less research effort has focused on bioprospecting for high starch containing strains than for high lipid strains.
As previously mentioned, development of management systems for the control of pathogens in open pond production systems is only in its infancy (Richmond et al., 1990; Davis and Laurens, 2010; Olivares et al., 2016; Lammers et al., 2017). Pathogen/herbivore infections can cause the very rapid in (as little as 2 days) and complete collapse of algae pond production systems. A variety of strategies have been used to control pathogens in algal cultivation systems. Chemical pathogen management practices for pathogen and herbivores have included the use of ammonia as a nitrogen source, phosphite as a source of phosphorous in strains engineered to oxidize phosphite, salts, and antibiotics (Lammers et al., 2017; Changko et al., 2020). Physical management practices include taking advantage of the differential sheer sensitivity of algae and versus pathogens to mechanical forces such as pumping or sonication (Lammers et al., 2017; Marrone et al., 2018). Competing weedy algae and pathogens can also be controlled using pulsed rather than continuous applications of macro-nutrients. The greatest success in managing pond crashes, however, has been achieved through the selection of algal strains that are naturally resistant to pathogens (Krishnan et al., 2021; Pang et al., 2022). In large part the molecular basis for disease resistance is not known. Interestingly, some species of Picochlorum have been shown to grow heterotrophically on bacteria presumably by phagocytosis opening the possibility for breeding or engineering this trait into algal production strains to control bacterial pathogens (Pang et al., 2022). Overall, pathogen control in algae remains one of the major opportunities for increasing in algal biomass yield.
Breeding algae for increased biomass production
Improvements in terrestrial crop production yields over the last 80 years can largely be attributed to improved agronomic practices and the application of advanced genetic breeding approaches for crop improvement. More recently, molecular assisted breeding and genomic selection tools have been applied to crop breeding and have accelerated the development of advanced crops with desirable properties. Molecular assisted breeding has been made possible through the development of low-cost and efficient genome sequencing tools. In contrast to crop plants, however, there has been little application of molecular-assisted breeding tools for the genetic improvement of algae except for the model species Chlamydomonas. Chlamydomonas is particularly amenable to breeding for enhanced yields since its dominant (mating type) generation is haploid, the dominant growth generation for Chlamydomonas is haploid. Ecotypes of different haploid mating types have been identified and used for breeding purposes. Recently, novel recombinant Chlamydomonas strains having three-fold increases in biomass yield relative to their parental strains have been generated through genetic crosses and strong selection regimes (Lucker et al., 2022). The increases in yield in the progeny were correlated with widespread alterations in gene frequencies between the two parental strains.
Unlike Chlamydomonas, however, the dominant generation for many algae is diploid. Thus, the challenge for mating many algae strains is how to induce gametogenesis and conjugation. Induction of meiosis, gamete formation and subsequent mating in diploid algae is currently an inexact science. The most common approach to induce meiosis in algae is nutrient stress in the dark (Huang and Beck, 2003; Přibyl P Light is a, 2013). In addition, exposure of gametes to a short pulse of blue light activates the phototropin receptor and can induce mating in some algal strains (Pfeifer et al., 2010). One approach to assess the effectiveness of various environmental treatments on gametogenesis and mating is tracking changes in DNA ploidy levels per cell in real time. This can be accomplished by quantifying relative changes in cellular DNA content using DNA binding fluorescent dyes coupled with fluorescent activated cell counting (Dept, 2017). As shown in Figure 1, Chlorella sorokiniana Cs-1228 cells starved of nitrogen in the dark for 6 h had half the DNA content of cells grown in nitrogen replete media indicative of the production of gametes under nitrogen stress (Dept, 2017). Gamete induction was observed in as little as 2 h after transfer of cells to media without nitrogen in the dark (Figure 1). At 22 h after nitrogen deprivation in the dark there was a small shift to higher DNA content/cell suggesting some level of mating and production of diploid cells. But the additional treatment of cells with a brief pulse of blue light (1–2 µmol photons/m2/s for 5 min) resulted in a near complete shift to diploid cells indicative of mating. The conversion of diploid to haploid cells competent for mating during dark nutrient stress treatments was further supported by the appearance of flagella required for mating at cells 6 h after treatment in the dark without nitrogen (Figure 1). Mating was also demonstrated by cytoplasmic exchange in blue-light treated cells (Figure 1) (Dept, 2017).
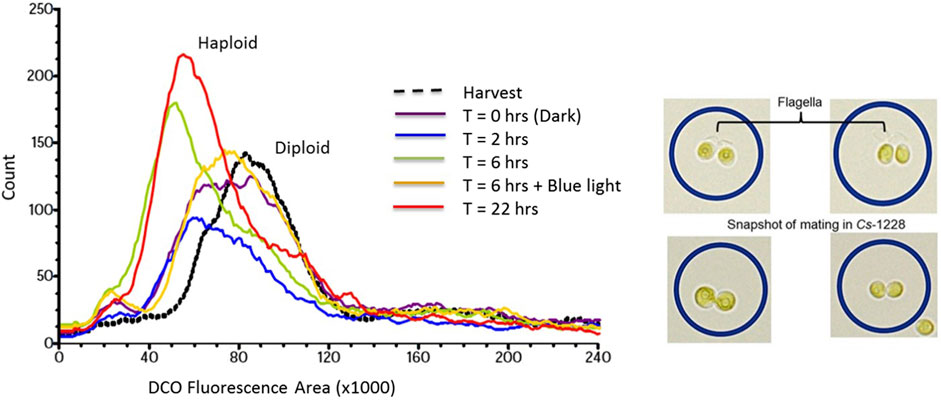
FIGURE 1. Changes in apparent DNA ploidy per cell following induction of meiosis, gamete formation and mating in Chlorella sorokiniana Cs-1228. Panel on the left shows changes in fluorescence levels (FL2-A) of a DNA binding dye per cell following different periods (0, 2, 6 and 22 h) of exposure in the dark to growth media lacking nitrogen followed by blue light treatment where indicated. The panel on the right shows the presence of flagella in cells grown for 6 h without nitrogen in the dark and the conjugation of cells and cytoplasmic exchange 6 h after minus nitrogen treatment in the dark plus blue light (Dept, 2017).
The development of procedures to induce gametogenesis and mating in other polyploid algae opens the door for the use of advanced genome wide association studies, molecular breeding tools and selection for enhanced performance characteristics and biomass yield (Přibyl P Light is a, 2013; Lucker et al., 2022). Overall, it is expected that the potential to increase biomass yields is great using breeding approaches given the vast genetic diversity that exists in natural algal ecotypes and the possibility to engineer algae (Baker et al., 2007; Ng et al., 2017; Crozet et al., 2018; Naduthodi et al., 2020; Ng et al., 2020; Shokravi et al., 2021).
Directed and adaptive evolution of algae for increased biomass yield
Biomass production can also be optimized for particular environments using directed or adaptive evolution approaches. When coupled with the generation of mutant populations for selection, additional improvements in yield resulting from new genotypes may also be achieved. Significantly, additional understanding of the metabolic factors that regulate growth can be achieved through comparative genomic, transcriptomic, proteomic and metabolomic studies of improved strains selected through directed evolution. For example, Haematococcus pluvialis strains selected for improved growth under high (15%) CO2 conditions were shown to have enhanced photosynthesis and glycolysis associated with the increased expression of genes involved in photosynthetic electron transport including PetH (ferredoxin–NADPC reductase) and ATPF0A (F-type H+-transporting ATPase subunit), and genes involved in respiration including, the PetJ (cytochrome c6) gene involved in NADPH generation. Additionally, genes of the C3 and C4 photosynthetic pathways in plants were overexpressed in improved strains including PddK, pyruvate, orthophosphate dikinase and FBA, fructose-bisphosphate aldolase. These genes facilitated carbon uptake under high CO2 growth conditions (Li et al., 2017). Among the greatest improvements in biomass yield was achieved using directed evolution approaches under high CO2 growth conditions.. As much as a 3-fold increase in algal biomass production (3.7 gdw/L) was achieved after 31 rounds of selection under high (10–20%) CO2 (Li et al., 2015). This increase in biomass yield was associated with the accumulation of greater amounts of starch per cell. It is important to note however, that the molecular adjustments resulting in increased biomass yields may not be the same for slow growing versus fast growing parental strains. If the biomass yield of the parental strain is low to begin with, modest metabolic changes resulting in large relative increases in biomass yield may not be applicable to very fast-growing strains. The metabolic bottlenecks limiting growth for each strain must be evaluated.
Engineering algae for increased biomass yield
Metabolic engineering strategies to increase biomass yields can be separated into at least four different categories including increasing carbon source strength, increasing carbon sink strength, alterations in the expression of master regulatory genes that control global carbon partitioning and flux rates, and strategies that control the cell cycle and cell division rates. First, we describe genetic manipulations that increase carbon source strength or rates of photosynthesis (Table 2).
Based on relative thermodynamic efficiency, the earliest steps in photosynthesis involved in light capture and utilization, i.e., the conversion of chlorophyll excited states into charge separated states driving photosynthetic electron transfer processes, are collectively the least efficient processes in biomass production (Subramanian et al., 2013; Okada et al., 2020; Vecchi et al., 2020). This is largely attributed to the fact that photosynthetic electron transfer rates saturate at 20–25% of full sunlight intensity in all algae and plants (Perrine et al., 2012). This is due to the fact that at full sunlight intensities the rate of photon capture by the light harvesting complexes is 5- to 10-fold faster than the rate-limiting steps in photosynthetic electron transport, i.e., the oxidation of plastoquinol by the cytochrome b6f complex (Baker et al., 2007; Perrine et al., 2012). In addition, as the proton gradient increases across the thylakoid membrane following illumination rates of plastoquinol oxidation are further diminished (Baker et al., 2007; Kiirats et al., 2009).
When addressing electron transport or metabolic steps having rate-limiting kinetics one strategy to alleviate bottlenecks is to overexpress the enzyme(s) or enzyme complex that are rate limiting. For example, to compensate for the slow rate of RuBP carboxylase/oxygenase (RuBisCO) catalysis plants and algae substantially overexpress the enzyme RuBisCO). In algae the cytochrome b6f complex, catalyzes the rate-limiting step in electron transport. The cytochrome b6f complex is present in equal stoichiometry with the photosystem I and II reaction center complexes (Kiirats et al., 2009). Thus, it is conceivable that elevating the relative number of cytochrome b6f complexes coupled with accelerated dissipation of the proton gradient by the ATPase complex, could compensate for the slow rate of plastoquinol oxidation. To date, however, there have been no reports of successfully overexpressing the cytochrome b6f complex to address this bottleneck in electron transport. This inability to substantially alter the stoichiometry of cytochrome b6f complexes may in part be due to the unique requirements for assembling stable cytochrome b6f complexes.
An alternative strategy for addressing inefficiencies in coupling chlorophyll excited state transitions to electron transport rates has been to reduce the optical cross-section or size of the light harvesting antenna to a level where the rate of photon capture corresponds with the rate of electron transfer. Reductions in light harvesting antenna size have been achieved by multiple means in mutant and engineered Chlamydomonas. One of the more successful strategies for reducing light harvesting antenna sizes has been reduction in chlorophyll b (Chl b) accumulation. Chl b is present only in the peripheral, nuclear-encoded, light harvesting pigment/protein complexes associated with reaction center complexes (photosystem II and I) and is not present in the chloroplast-encoded reaction center proximal antenna proteins or the core reaction center complex proteins. The core reaction center proteins only contain only Chl a (Melis, 2009; Perrine et al., 2012). Reduction in Chl b levels destabilizes the light harvesting protein subunits resulting in the turnover of Chl b-binding proteins effectively reducing the peripheral light harvesting antenna size (Melis, 2009; Perrine et al., 2012; Negi et al., 2020). The earliest studies on reduction of Chl b levels in algae were those carried out by Melis and colleagues (Melis, 2009), Their studies on antennae size modulation focused on the selection for mutants that did not accumulate Chl b. When grown under photoheterotrophic conditions the Chl b-less mutants showed some improvements in yield. However, when grown strictly photosynthetically chlorophyll a oxidase mutants, unable to produce any Chl b, had substantially reduced growth rates compared to their wild-type parents (Perrine et al., 2012).
But all things in moderation. By fine tuning Chl b levels by partially but not completely suppressing chlorophyl a oxygenase (CAO) activity it was determined that moderate reductions in light harvesting antenna size resulted in the greatest increases in photosynthetic efficiency. The highest biomass producing strains were shown to have lost one LHCII trimer complex relative to wild-type algae, equivalent to a Chl a/b ratio of 5 (Perrine et al., 2012). Transgenic algae having Chl a/b ratios lower than 5 (larger antenna sizes) including wild-type algae (Chl a/b = 2.0–2.5) or algae having Chl a/b ratios greater than 5 (smaller antenna) had reduced photosynthetic rates and reduced abilities to tolerate high light stress due to a reduced capacity for non-photochemical quenching of excess captured energy. Significantly, biomass yields for algae having Chl a/b ratios of 5 was 40% greater than those of wild-type algae when grown in photobioreactors simulating outdoor pond cultivation conditions (Perrine et al., 2012). Similar observations were made for plants engineered to have reduced light harvesting antenna sizes. Optimal biomass production in Camelina was achieved in plants having a Chl a/b ratio of 5 (Friedland et al., 2019).
Reductions in light harvesting antenna complexes have also been achieved by overexpression of the NAB1 protein which regulates the expression of multiple light harvesting complex protein (Beckmann et al., 2009). The NAB1 protein is a translational repressor that binds to the 5′ end of transcripts encoding members of the light harvesting complex. Overexpression of the activated (reduced disulfides) NAB1 protein resulted in a 17% reduction in antenna size in transgenic Chlamydomonas corresponding to a Chl a/b ratio of 2.2. Biomass yields were 30% greater in NAB1 transgenics relative to the parental wild-type strain when grown in photobioreactors supplemented with elevated CO2. Additional approaches have been used to engineer light harvesting antenna size. Mussgnug et al (Mussgnug et al., 2007) described a more generalized approach to reduce light harvesting antenna size by expressing an RNAi construct that targeted a common DNA sequence present in the 5′ untranslated region of multiple LHCII family members. They observed a 30% reduction in Chl content/cell leading to a Chl a/b ratio of 4. Under mixotrophic growth conditions the transgenics had a 45% faster growth rate than wild type, however, no biomass studies were reported.
To date, the greatest biomass increases resulting from genetic engineering approaches have been achieved through the light regulation of light harvesting antenna size (Negi et al., 2020). Dynamic regulation of light harvesting antenna size was achieved by co-expression of a light-regulated NAB1 translational repressor along with coexpression of a modified CAO gene having a 5’ NAB1 binding light response element expressed in a CAO knock strain. In contrast, under high light conditions light harvesting antenna sizes were reduced due to increased NAB1 expression. In contrast, under low-light growth conditions antenna sizes were increased due reduced NAB1 expression in transgenic strains. It was also observed that sensitivity to photoinhibition was reduced relative to wild type cells in the transgenic strains relative to wild type.
Another novel approach to alter light harvesting efficiency has been the expression of light absorbing proteins in the stroma of chloroplasts. The expression of the green fluorescent protein in the chloroplasts of the diatom Phaeodactylum tricornutum resulted in a 50% increase in biomass yield (0.23 gdw/L/day) under simulated pond growth conditions supplemented with 1% CO2 (Fu et al., 2017). This increase in biomass yield was only observed when the transgenics were grown under light intensities (400 µmoles photons/m2/sec) that were greater than those that light-saturate photosynthetic electron transport. Interestingly, non-photochemical quenching in GFP expressing strains was reduced under high-light growth conditions relative to wild type. These results suggest that GFP was shading the light harvesting apparatus resulting in reduced energy dissipation through NPQ allowing for enhanced photosynthetic electron transport at high light levels.
One of the more elegant strategies to enhance algal biomass production has been the genetic manipulation of master growth regulatory genes that globally regulate multiple genes involved in biomass accumulation. One such algal regulatory gene is the blue light photoreceptor, phototropin (Im et al., 2006; Pfeifer et al., 2010; Petroutsos et al., 2016). In Chlamydomonas phototropin regulates the expression of multiple gene systems including genes involved in the sexual life cycle, eye spot size, and genes involved chlorophyll, carotenoid, and chlorophyll binding protein synthesis (Im et al., 2006; Petroutsos et al., 2016). Negi et. al. (Negi et al., 2017) demonstrated that phototropin knock out mutants had higher Chl a/b ratios than wild type cells ranging from 2.9 when grown under low light to 3.4 when grown under high light conditions associated with a reduction in light harvesting antenna size. In addition, cell division rates and biomass accumulation rates were substantially increased in phototropin knock out mutants. RNAseq experiments indicated that the expression of multiple genes involved photosynthesis, carbon metabolism, and cell division rates were significantly altered in phototropin mutants. Phototropin mutants had a 2- to 5-fold increase in the expression levels of the Rieske Fe-S protein (cytochrome b6f complex), the Calvin-Benson-Bassham Cycle (CBBC) enzymes ribulose-1,5-bisphosphate carboxylase/oxygenase, sedoheptulose 1,7 bisphosphatase glyceraldehyde-3- phosphate dehydrogenase, carbonic anhydrase, and genes involved in starch synthesis including ADP glucose pyrophosphorylase, starch synthase, and genes involved in respiration and fatty acid biosynthesis In addition, the SNRK1 gene that shares homology with the major growth regulatory genes KIN10 and KIN11 in plants had ten-fold higher expression levels in phototopin mutants (Baena-González et al., 2007; Negi et al., 2017). Genes involved in cell cycle control including NIMA (never in mitosis), NEK2, NEK6 (NIMA related kinases), RCC1 (regulator of chromosome condensation, cyclin and cyclin-dependent kinases: Cyclin-dependent kinases, and MAT3 a homolog of retinoblastoma protein (MAT3/RB) were also upregulated 2-to 15-fold in photoptropin mutants relative to wild type. Overall, there was a two-fold increase in biomass yield in phototropin mutants relative to wild-type cells when grown under environmental conditions that mimicked outdoor light and temperature conditions (Negi et al., 2017). However, there is some evidence that phototropin mutants may be more susceptible to photoinhibition than wild type (Li et al., 2017) These results indicate that the coordinated regulation of source and sink strength genes as well as cell cycle control genes can enhance biomass accumulation in algae.
In contrast to efforts to improve biomass yields through alterations in light use efficiency, there have been limited efforts to improve algal biomass yields through manipulation of CBBC enzymes or by increasing carbon sink strength. There have, however, been substantial efforts to increase lipid accumulation in microalgae through genetic engineering and genome editing approaches often at a loss in biomass accumulation (Gonzalez-Esquer et al., 2019; Fayyaz et al., 2020; Ng et al., 2020; Shokravi et al., 2021). One approach to increase biomass accumulation through manipulation of the CBBC activity was achieved through enhancement of fructose 1,6-bisphosphate aldolase activity, an enzyme playing a major role in carbon flux control in the CBBC (Yang et al., 2017). Transgenic Chlorella cells overexpressing fructose 1,6-bisphosphate aldolase were shown to have 20% higher rates of photosynthesis but only a 6% increase in biomass yields relative to wild type. The small increase biomass yields may be due to the low light conditions under which the algae were cultivated. Additionally, it has been demonstrated that genetic complementation of Chlamydomonas mutants impaired in starch synthesis (ADP glucopyrophosphorylase mutants) resulted in reduced generation of potentially toxic reactive oxygen species indicating the critical role starch synthesis plays as an electron sink (Saroussi et al., 2019). Finally, starch hyperaccumulation mutants impaired in glycolysis were shown to have 72% higher cell numbers at stationary phase than wild-type cells (Koo et al., 2017). Collectively, these results demonstrate the critical role carbon metabolism and starch accumulation plays in overall algal biomass accumulation.
Finally, biomass yields are also determined by cell cycle kinetics and changes in cell volume (Li et al., 2016). The regulation of cell cycle activity and cell size in algae, however, is less well understood than central metabolism but offers significant opportunities for manipulation of biomass production (Li et al., 2016).
Conclusion
Microalgae are gaining increased attention as possible alternative food sources, platforms to produce high value co-products, biofuels, and for long-term carbon sequestration in geologic formations. Recent life cycle analyses of algal production systems have determined that algal biomass production rates are one of the major bottlenecks impeding the commercialization of algal biomass, bioproducts and biofuel products. Thus, the need for enhanced algal biomass production to make algae commercially competitive with other biomass production platforms.
Theoretical and empirical evidence indicate that algae that accumulate starch as their major reduced energy reserve have faster growth rates and greater biomass accumulation potential than algae that predominantly store lipids as energy reserves. Since carbon skeletons for lipid production are in large part derived from starch it is apparent that the focus on identifying algal strains that are high lipid accumulators may not be the most effective bioprospecting strategy for identifying algal strains that have higher biomass yield. A potentially better strategy for identifying new commercials strains of algal for coproduct production may be to identify the highest starch accumulating species.
Substantial progress has, however, been made in identifying algal strains that have stable, long-term biomass production in outdoor environments. Genome sequencing and the development of molecular toolboxes including genetic transformation, enhanced and inducible gene expression cassettes, and genome editing tools will substantially expand the potential of algae as sustainable platforms for the production of high value biomolecules and for carbon sequestration. Progress has been made in improving light use efficiency in photosynthesis resulting in nearly a 200% increase in biomass yield in simulated outdoor growth studies. Surprisingly, there has been less research focus on increasing carbon flux through the CBBC or increasing carbon sink strength to improve biomass yields.
Perhaps, the greatest unrealized potential for improvements in algal biomass yields is through the application of molecular assisted breeding of algae. With the increased availability of annotated algal genomes, isolation of ecotypes from diverse environments, a growing understanding of methods to induce gametogenesis and mating and the availability of high throughput screening and selection systems for identifying fast growing progeny of sexual crosses the future for algae having enhanced biomass yields is bright.
Author contributions
RS drafted the review article. SS contributed the sections on Chlorella sorokiniana mating and Figure 1.
Conflict of interest
The authors declare that the research was conducted in the absence of any commercial or financial relationships that could be construed as a potential conflict of interest.
Publisher’s note
All claims expressed in this article are solely those of the authors and do not necessarily represent those of their affiliated organizations, or those of the publisher, the editors and the reviewers. Any product that may be evaluated in this article, or claim that may be made by its manufacturer, is not guaranteed or endorsed by the publisher.
Abbreviations
Bu, bushels; CBBC, Calvin-Benson-Bassham Cycle; Chl, chlorophyll; gdw, gram dry weight.
References
Aketo, T., Hashizume, R., Yabu, Y., Hoshikawa, Y., Nojima, D., Maeda, Y., et al. (2020). Characterization of a novel marine unicellular alga, Pseudoneochloris sp. strain NKY372003 as a high carbohydrate producer. J. Biosci. Bioeng. 129, 687–692. doi:10.1016/j.jbiosc.2019.12.010
Baena-González, E., Rolland, F., Thevelein, J. M., and Sheen, J. (2007). A central integrator of transcription networks in plant stress and energy signalling. Nature 448, 938–942. doi:10.1038/nature06069
Baker, N. R., Harbinson, J., and Kramer, D. M. (2007). Determining the limitations and regulation of photosynthetic energy transduction in leaves. Plant, Cell Environ. 30, 1107–1125. doi:10.1111/j.1365-3040.2007.01680.x
Barry, A., Wolfe, A., English, C., and Ruddick, C. Lambert D. 2016 national algal biofuels technology review USDOE office of energy efficiency and renewable energy (EERE), Bioenergy technologies office (EE-3B). Available at: https://www.energy.gov/sites/prod/files/2016/06/f33/national_algal_biofuels_technology_review.pdf.
Beckmann, J., Lehr, F., Finazzi, G., Hankamer, B., Posten, C., Wobbe, L., et al. (2009). Improvement of light to biomass conversion by de-regulation of light-harvesting protein translation in Chlamydomonas reinhardtii. J. Biotechnol. 142, 70–77. doi:10.1016/j.jbiotec.2009.02.015
Benedetti, M., Vecchi, V., Barera, S., and Dall’Osto, L. (2018). Biomass from microalgae: The potential of domestication towards sustainable biofactories. Microb. Cell Fact. 17, 173. doi:10.1186/s12934-018-1019-3
Bolton, J. R., and Hall, D. O. (1991). The maximum efficiency of photosynthesis. Photochem. Photobiol. 53, 545–548. doi:10.1111/j.1751-1097.1991.tb03668.x
Boussiba, S., Vonshak, A., Cohen, Z., and Richmond, A. (1987). Development of an outdoor system for production of lipid-rich halotolerant microalgae. Available at: https://www. osti. gov/biblio/66852 89.
Cano, M., Karns, D. A. J., Weissman, J. C., Heinnickel, M. L., and Posewitz, M. C. (2021). Pigment modulation in response to irradiance intensity in the fast-growing alga Picochlorum celeri. Algal Res. 58, 102370. doi:10.1016/j.algal.2021.102370
Changko, S., Rajakumar, P. D., Young, R. E. B., and Purton, S. (2020). The phosphite oxidoreductase gene, ptxD as a bio-contained chloroplast marker and crop-protection tool for algal biotechnology using Chlamydomonas. Appl. Microbiol. Biotechnol. 104, 675–686. doi:10.1007/s00253-019-10258-7
Chisti, Y. (2013). Constraints to commercialization of algal fuels. J. Biotechnol. 167, 201–214. doi:10.1016/j.jbiotec.2013.07.020
Crozet, P., Navarro, F. J., Willmund, F., Mehrshahi, P., Bakowski, K., Lauersen, K. J., et al. (2018). Birth of a photosynthetic chassis: A MoClo toolkit enabling synthetic biology in the microalga Chlamydomonas reinhardtii. ACS Synth. Biol. 7, 2074–2086. doi:10.1021/acssynbio.8b00251
Dahlin, L. R., Gerritsen, A. T., Henard, C. A., Van Wychen, S., Linger, J. G., Kunde, Y., et al. (2019). Development of a high-productivity, halophilic, thermotolerant microalga Picochlorum renovo. Commun. Biol. 2, 388. doi:10.1038/s42003-019-0620-2
Davis, R. E., Fishman, D. B., Frank, E. D., Johnson, M. C., Jones, S. B., Kinchin, C. M., et al. (2014). Integrated evaluation of cost, emissions, and resource potential for algal biofuels at the national scale. Environ. Sci. Technol. 48, 6035–6042. doi:10.1021/es4055719
Davis, R., and Laurens, L. M. L. (2010). Algal biomass production via open pond algae farm cultivation: 2019 state of technology and future research. Available at: https://www. nrel. gov/docs/fy20o sti/76569. pdf.
Dementyeva, P., Freudenberg, R. A., Baier, T., Rojek, K., Wobbe, L., Weisshaar, B., et al. (2021). A novel, robust and mating-competent Chlamydomonas reinhardtii strain with an enhanced transgene expression capacity for algal biotechnology. Biotechnol. Rep. (Amst). 31, e00644. doi:10.1016/j.btre.2021.e00644
Dept, U. S. (2017). Energy. Project peer review report. Available at: https://www.energy.gov/eere/bioenergy/downloads/2017-project-peer-review-report.
Fayyaz, M., Chew, K. W., Show, P. L., Ling, T. C., Ng, I. S., and Chang, J. S. (2020). Genetic engineering of microalgae for enhanced biorefinery capabilities. Biotechnol. Adv. 43, 107554. doi:10.1016/j.biotechadv.2020.107554
Finkel, Z. V., Follows, M. J., Liefer, J. D., Brown, C. M., Benner, I., and Irwin, A. J. (2016). Phylogenetic diversity in the macromolecular composition of microalgae. PLoS One 11, e0155977. doi:10.1371/journal.pone.0155977
Friedland, N., Negi, S., Wu, G., Ma, L., Flynn, S., Cahoon, E., et al. (2019). Fine-tuning the photosynthetic light harvesting apparatus for improved photosynthetic efficiency and biomass yield. Sci. Rep. 9, 13028–13112. doi:10.1038/s41598-019-49545-8
Fu, W., Chaiboonchoe, A., Khraiwesh, B., Sultana, M., Jaiswal, A., Jijakli, K., et al. (2017). Intracellular spectral recompositioning of light enhances algal photosynthetic efficiency. Sci. Adv. 3, e1603096. doi:10.1126/sciadv.1603096
Gonzalez-Esquer, C. R., Wright, K. T., Sudasinghe, N., Carr, C. K., Sanders, C. K., Turmo, A., et al. (2019). Demonstration of the potential of Picochlorum soloecismus as a microalgal platform for the production of renewable fuels. Algal Res. 43, 101658. doi:10.1016/j.algal.2019.101658
Guiry, M. D., and Guiry, G. M. (2021). AlgaeBase. World-wide electronic publication. Galway: National University of Ireland. Available at: https://www.algaebase.org.
Huang, K., and Beck, C. F. (2003). Phototropin is the blue-light receptor that controls multiple steps in the sexual life cycle of the green alga Chlamydomonas reinhardtii. Proc. Natl. Acad. Sci. U. S. A. 100, 6269–6274. doi:10.1073/pnas.0931459100
Huesemann, M., Chavis, A., Edmundson, S., Rye, D., Hobbs, S., Sun, N., et al. (2018). Climate-simulated raceway pond culturing: Quantifying the maximum achievable annual biomass productivity of Chlorella sorokiniana in the contiguous USA. J. Appl. Phycol. 30, 287–298. doi:10.1007/s10811-017-1256-6
Im, C. S., Eberhard, S., Huang, K., Beck, C. F., and Grossman, A. R. (2006). Phototropin involvement in the expression of genes encoding chlorophyll and carotenoid biosynthesis enzymes and LHC apoproteins in Chlamydomonas reinhardtii. Plant J. 48, 1–16. doi:10.1111/j.1365-313x.2006.02852.x
Kiirats, O., Cruz, J. A., Edwards, G. E., and Kramer, D. M. (2009). Feedback limitation of photosynthesis at high CO2 acts by modulating the activity of the chloroplast ATP synthase. Funct. Plant Biol. 36, 893–901. doi:10.1071/fp09129
Koo, K. M., Jung, S., Lee, B. S., Kim, J. B., Jo, Y. D., Choi, H. I. l., et al. (2017). The mechanism of starch over-accumulation in Chlamydomonas reinhardtii high-starch mutants identified by comparative transcriptome analysis. Front. Microbiol. 8, 858–869. doi:10.3389/fmicb.2017.00858
Krishnan, A., Likhogrud, M., Cano, M., Edmundson, S., Melanson, J. B., Huesemann, M., et al. (2021). Picochlorum celeri as a model system for robust outdoor algal growth in seawater. Sci. Rep. 11, 11649. doi:10.1038/s41598-021-91106-5
Kruse, O., Rupprecht, J., Bader, K. P., Thomas-Hall, S., Schenk, P. M., Finazzi, G., et al. (2005). Improved photobiological H2 production in engineered green algal cells. J. Biol. Chem. 280, 34170–34177. doi:10.1074/jbc.m503840200
Kruse, O., Rupprecht, J., Mussgnug, J. H., Dismukes, G. C., and Hankamer, B. (2005). Photosynthesis: A blueprint for solar energy capture and biohydrogen production technologies. Photochem. Photobiol. Sci. 4, 957–970. doi:10.1039/b506923h
Kumar, A., Falcao, V. R., and Sayre, R. T. (2013). Evaluating nuclear transgene expression systems in Chlamydomonas reinhardtii. Algal Res. 2, 321–332. doi:10.1016/j.algal.2013.09.002
Kumar, A., Perrine, Z., Stroff, C. L., Postier, B. A., Coury, D. T., Sayre, R. T., et al. (2016). Molecular tools for bioengineering eukaryotic microalgae. Curr. Biotechnol. 5, 93–108. doi:10.2174/2211550105666160127002147
Lammers, P. J., Huesemann, M., Boeing, W., Anderson, D. B., Arnold, R. G., Bai, X., et al. (2017). Review of the cultivation program within the national alliance for advanced biofuels and bioproducts. Algal Res. 22, 166–186. doi:10.1016/j.algal.2016.11.021
LaPanse, A. J., Krishnan, A., and Posewitz, M. C. (2015). Adaptive laboratory evolution for algal strain improvement: Methodologies and applications. Bioresour. Technol. 185, 269–275. doi:10.1016/j.algal.2020.102122
Laws, E. A., Taguchi, S., Hirata, J., and Pang, L. (1986). High algal production rates achieved in a shallow outdoor flume. Biotechnol. Bioeng. 28, 191–197. doi:10.1002/bit.260280207
Li, D., Zhao, Q., Wei, W., and Sun, Y. (2015). Improving high carbon dioxide tolerance and carbon dioxide fixation capability of Chlorella sp. by adaptive laboratory evolution. Bioresour. Technol. 185, 269–275. doi:10.1016/j.biortech.2015.03.011
Li, K., Cheng, J., Lu, H., Yang, W., Zhou, J., and Cen, K. (2017). Transcriptome based analysis on carbon metabolism of Haematococcus pluvialis mutant under 15% CO2. Bioresour. Technol. 233, 313–321. doi:10.1016/j.biortech.2017.02.121
Li, Y., Liu, D., López-Paz, C., Olson, B. J. S. C., and Umen, J. G. (2016). A new class of cyclin dependent kinase in Chlamydomonas is required for coupling cell size to cell division. Elife 5, e10767–28. doi:10.7554/elife.10767
Lucker, B. F., Temple, J. A., Panchy, N. L., Benning, U. F., Bibik, J. D., Neofotis, P. G., et al. (2022). Selection-enriched genomic loci (SEGL) reveals genetic loci for environmental adaptation and photosynthetic productivity in Chlamydomonas reinhardtii. Algal Res. 64, 102709.
Marrone, B. L., Lacey, R. E., Anderson, D. B., Bonner, J., Coons, J., Dale, T., et al. (2018). Review of the harvesting and extraction program within the national alliance for advanced biofuels and bioproducts. Algal Res. 33, 470–485. doi:10.1016/j.algal.2017.07.015
Melis, A. (2009). Solar energy conversion efficiencies in photosynthesis: Minimizing the chlorophyll antennae to maximize efficiency. Plant Sci. 177, 272–280. doi:10.1016/j.plantsci.2009.06.005
Mosey, M., Douchi, D., Knoshaug, E. P., and Laurens, L. M. L. (2021). Methodological review of genetic engineering approaches for non-model algae. Algal Res. 54, 102221. doi:10.1016/j.algal.2021.102221
Mucko, M., Padisák, J., Gligora Udovič, M., Pálmai, T., Novak, T., Medić, N., et al. (2020). Characterization of a high lipid-producing thermotolerant marine photosynthetic pico alga from genus Picochlorum (Trebouxiophyceae). Eur. J. Phycol. 55, 384–399. doi:10.1080/09670262.2020.1757763
Mussgnug, J. H., Thomas-Hall, S., Rupprecht, J., Foo, A., Klassen, V., McDowall, A., et al. (2007). Engineering photosynthetic light capture: Impacts on improved solar energy to biomass conversion. Plant Biotechnol. J. 5, 802–814. doi:10.1111/j.1467-7652.2007.00285.x
Naduthodi, M. I. S., Claassens, N. J., D’Adamo, S., van der Oost, J., and Barbosa, M. J. (2020). Synthetic Biology Approaches to enhance microalgal productivity. Trends Biotechnol. 39, 1019–1036. doi:10.1016/j.tibtech.2020.12.010
Negi, S., Barry, A. N., Friedland, N., Sudasinghe, N., Subramanian, S., Pieris, S., et al. (2016). Impact of nitrogen limitation on biomass, photosynthesis, and lipid accumulation in Chlorella sorokiniana. J. Appl. Phycol. 6, 803–812. doi:10.1007/s10811-015-0652-z
Negi, S., Perrine, Z., Friedland, N., Kumar, A., Tokutsu, R., Minagawa, J., et al. (2020). Light regulation of light-harvesting antenna size substantially enhances photosynthetic efficiency and biomass yield in green algae. Plant J. 103, 584–603. doi:10.1111/tpj.14751
Negi, S., Sayre, R. T., and Starkenburg, S. R. (2017). Productivity and bioproduct formation in phototropin knock/out mutants in microalgae. US: US patent. 10590398 B2.
Neofotis, P., Huang, A., Sury, K., Chang, W., Joseph, F., Gabr, A., et al. (2016). Characterization and classification of highly productive microalgae strains discovered for biofuel and bioproduct generation. Algal Res. 15, 164–178. doi:10.1016/j.algal.2016.01.007
Ng, I. S., Keskin, B. B., and Tan, S. I. (2020). A critical review of genome editing and synthetic biology applications in metabolic engineering of microalgae and cyanobacteria. Biotechnol. J. 15, 1900228. doi:10.1002/biot.201900228
Ng, I. S., Tan, S. I., Kao, P. H., Chang, Y. K., and Chang, J. S. (2017). Recent developments on genetic engineering of microalgae for biofuels and bio-based chemicals. Biotechnol. J. 12, 1600644. doi:10.1002/biot.201600644
Okada, K., Fujiwara, S., and Tsuzuki, M. (2020). Energy conservation in photosynthetic microorganisms. J. Gen. Appl. Microbiol. 66, 59–65. doi:10.2323/jgam.2020.02.002
Olivares, J., Unkefer, C. J., Sayre, R. T., Magnuson, J. K., Anderson, D. B., Baxter, I., et al. (2016). Review of the algal biology program within the national alliance for advanced biofuels and bioproducts. Algal Res. 22, 187–215. doi:10.1016/j.algal.2016.06.002
Pang, M., Liu, K., and Liu, H. (2022). Evidence for mixotrophy in pico-chlorophytes from a new Picochlorum (trebouxiophyceae) strain. J. Phycol. 58, 80–91. doi:10.1111/jpy.13218
Perrine, Z., Negi, S., and Sayre, R. T. (2012). Optimization of photosynthetic light energy utilization by microalgae. Algal Res. 1, 134–142. doi:10.1016/j.algal.2012.07.002
Petroutsos, D., Tokutsu, R., Maruyama, S., Flori, S., Greiner, A., Magneschi, L., et al. (2016). A blue-light photoreceptor mediates the feedback regulation of photosynthesis. Nature 537 (7621), 563–566. PMID: 27626383. doi:10.1038/nature19358
Pfeifer, A., Mathes, T., Lu, Y., Hegemann, P., and Kottke, T. (2010). Blue light induces global and localized conformational changes in the kinase domain of full-length phototropin. Biochemistry 49, 1024–1032. doi:10.1021/bi9016044
Přibyl P Light is a crucial signal for zoosporogenesis and gametogenesis in some green microalgae. Eur. J. Phycol., 2013, 48, 106–115.
Rajvanshi, M., and Sayre, R. T. (2020). Recent advances in algal biomass production. London: IntechOpen, Biomass. Available at: doi:10.5772/intechopen.94218https://www.intechopen.com/online-first/recent-advances-in-algal-biomass-production
Richmond, A., Lichtenberg, E., Stahl, B., and Vonshak, A. (1990). Quantitative assessment of the major limitations on productivity of Spirulina platensis in open raceways. J. Appl. Phycol. 2, 195–206. doi:10.1007/bf02179776
Saroussi, S., Karns, D. A. J., Thomas, D. C., Bloszies, C., Fiehn, O., Posewitz, M. C., et al. (2019). Alternative outlets for sustaining photosynthetic electron transport during dark-to-light transitions. Proc. Natl. Acad. Sci. U. S. A. 166, 11518–11527. doi:10.1073/pnas.1903185116
Sarwer, A., Hamed, S. M., Osman, A. I., Jamil, F., Al-Muhtaseb, A. H., Alhajeri, N. S., et al. (2022). Algal biomass valorization for biofuel production and carbon sequestration: A review. Environ. Chem. Lett. doi:10.1007/s10311-022-01458-1
Sayre, R. T. (2010). Microalgae: The potential for carbon capture. Bioscience 60, 722–727. doi:10.1525/bio.2010.60.9.9
Shokravi, H., Shokravi, Z., Heidarrezaei, M., Ong, H. C., Rahimian Koloor, S. S., Petrů, M., et al. (2021). Fourth generation biofuel from genetically modified algal biomass: Challenges and future directions. Chemosphere 2021, 131535. doi:10.1016/j.chemosphere.2021.131535
Subramanian, S., Barry, A. N., Pieris, S., and Sayre, R. T. (2013). Comparative energetics and kinetics of autotrophic lipid and starch metabolism in chlorophytic microalgae: Implications for biomass and biofuel production. Biotechnol. Biofuels 6, 150–162. doi:10.1186/1754-6834-6-150
Sun, H., Zhao, W., Mao, X., Li, Y., Wu, T., and Chen, F. (2018). High-value biomass from microalgae production platforms: Strategies and progress based on carbon metabolism and energy conversion. Biotechnol. Biofuels 11, 227. doi:10.1186/s13068-018-1225-6
Unkefer, C. J., Sayre, R. T., Magnuson, J. K. J., Anderson, D. B., Baxter, I., Blaby, I. K., et al. (2017). Review of the algal biology program within the national alliance for advanced biofuels and bioproducts. Algal Res. 22, 187–215. doi:10.1016/j.algal.2016.06.002
Vecchi, V., Barera, S., Bassi, R., and Dall’osto, L. (2020). Potential and challenges of improving photosynthesis in algae. Plants (Basel).Plants 9, 67. doi:10.3390/plants9010067
Wang, Y., Duanmu, D., and Spalding, M. H. (2011). Carbon dioxide concentrating mechanism in Chlamydomonas reinhardtii: Inorganic carbon transport and CO2 recapture. Photosynth. Res. 109, 115–122. doi:10.1007/s11120-011-9643-3
Weissman, J. C., and Goebel, R. P. (1987). Factors affecting the photosynthetic yield of microalgae. 1986 aquatic species program annual report. Available at: https://www. osti. gov/biblio/66852 89.
Weyer, K., Bush, D., Darzins, A., and Willson, B. (2010). Theoretical maximum algal oil production. Bioenerg. Res. 3, 204–213. doi:10.1007/s12155-009-9046-x
Yang, B., Liu, J., Ma, X., Guo, B., Liu, B., Wu, T., et al. (2017). Genetic engineering of the Calvin cycle toward enhanced photosynthetic CO2 fixation in microalgae. Biotechnol. Biofuels 10, 229–241. doi:10.1186/s13068-017-0916-8
Zhang, B., and Wu, J. (2021). Meng F. Adaptive laboratory evolution of microalgae: A review of the regulation of growth, stress resistance, metabolic processes, and biodegradation of pollutants. Front. Microbiol. 12, 737248. doi:10.3389/fmicb.2021.737248
Keywords: microalgae, biomass, biofuels, photosynthesis, genetics, carbon capture
Citation: Subramanian S and Sayre RT (2022) The right stuff; realizing the potential for enhanced biomass production in microalgae. Front. Energy Res. 10:979747. doi: 10.3389/fenrg.2022.979747
Received: 27 June 2022; Accepted: 23 August 2022;
Published: 13 September 2022.
Edited by:
Blake Hovde, Biosciences Division, Los Alamos National Laboratory (DOE), United StatesReviewed by:
Weiqi Fu, Zhejiang University, ChinaTonmoy Ghosh, Indian Institute of Technology Indore, India
Copyright © 2022 Subramanian and Sayre. This is an open-access article distributed under the terms of the Creative Commons Attribution License (CC BY). The use, distribution or reproduction in other forums is permitted, provided the original author(s) and the copyright owner(s) are credited and that the original publication in this journal is cited, in accordance with accepted academic practice. No use, distribution or reproduction is permitted which does not comply with these terms.
*Correspondence: Richard T Sayre, cmljaGFyZHRzYXlyZUBnbWFpbC5jb20=