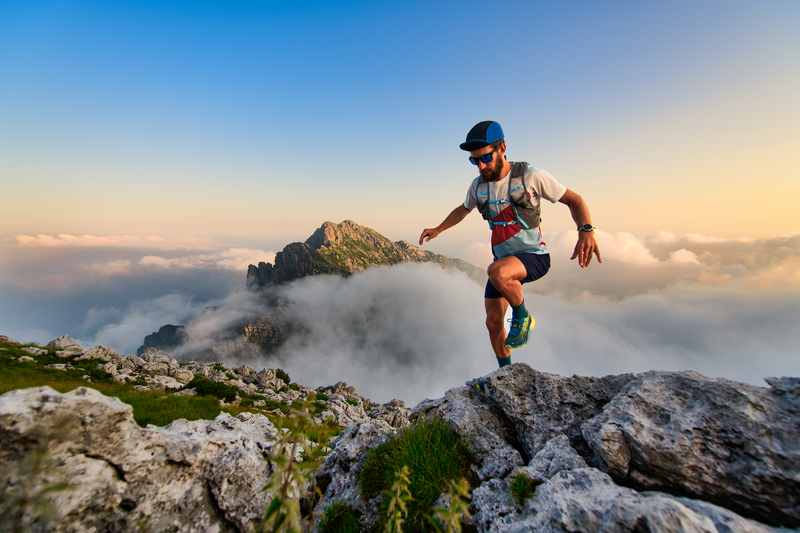
94% of researchers rate our articles as excellent or good
Learn more about the work of our research integrity team to safeguard the quality of each article we publish.
Find out more
MINI REVIEW article
Front. Energy Res. , 24 August 2022
Sec. Electrochemical Energy Storage
Volume 10 - 2022 | https://doi.org/10.3389/fenrg.2022.951701
The rapid development of modern electronic technology is in urgent need of further breakthroughs to actualize high-energy, high-power, and long cycling energy storage equipment. Carbon-based supercapacitors (CSs) are potential high-power devices that can stock electrical energy at the electrode–electrolyte interface rather than by diffusing ions inside electrodes. However, the commercial CSs using active carbon (AC) suffer from restricted energy densities on account of relatively small specific surface area, poor porosity, and low electrochemical activity. In recent years, various tactics have been applied to enhance the electrochemical properties of carbon-based electrode, and fruitful successes have been achieved. This mini review first introduces the concerned charge storage mechanisms of CSs, which is followed by a straightforward summary of the pivotal factors affecting the electrochemical performance. Then, the novel fabrication strategies of porous carbon at different dimensions are exemplified and summarized to prepare large-capacitance electrodes. The current challenges and promising future research for exploiting the state-of-the-art supercapacitors are also discussed.
Along with the rapid consumption of fossil fuels and surging global warming problem, the need to develop sustainable energy becomes increasingly urgent. Exploring efficient energy storage systems is key to greatly promote the utilization of renewable resources and mitigate green-house effect (Cao et al., 2017; Chen et al., 2020; Zhang et al., 2020a). As essential energy storage devices, supercapacitors (SCs), like other energy storage systems, have been widely concerned by academia and industry, and specific power/energy density of various types of energy storage systems is shown in Figure 1 (Wu et al., 2020). Different from commercial rechargeable batteries that depend on the reaction mechanism of the bulk phase of electrode material, SCs have large power density, good rate capability, long circular life, and fast charge/discharge because their energy conversion takes place between the surface of the electrode material and the adsorbed electrolyte layer at the interface. Therefore, SCs have been broadly used for electric vehicles, shape memory, smart instruments, and military purposes by supplying back-up power and obstructing power outages (Luo et al., 2020; Wu et al., 2020; Yin et al., 2020). On basis of the energy storage mechanisms (faradaic or non-faradaic redox reactions) and electrode material constitutes (symmetric or asymmetric systems), SCs can be mainly divided into three types: electric double-layer capacitors (EDLCs), pseudocapacitors (PCs), and asymmetric hybrid capacitors (AHCs). For most of the advanced commercial SCs, a variety of carbon-based materials with disparate physical–chemical properties are used as representative active electrode materials (Faraji and Nasir Ani, 2015; Li et al., 2020a). Of these, active carbon (AC), owing to its merits of large specific surface area, low production cost, nontoxic nature, and easy availability, is widely identified as a practical electrode material. Although AC-based SCs typically have large power density (>10 kW kg−1), the lower energy density (<20 Wh kg−1) compared to lithium-ion batteries (>250 Wh kg−1) is considered a major barrier to their further application in large-scale energy storage (Gopalakrishnan and Badhulika 2018; Cheng et al., 2020; Luo et al., 2021).
FIGURE 1. Specific power/energy density of various types of energy storage systems (Wu et al., 2020).
To address this problem, designing poriferous carbonaceous materials with controllable morphology and nanostructures has been considered to be one of the most effective ways. Highly nanostructured carbon materials can significantly enhance the double-layer capacitance and rate properties of the porosint in contrast with their bulk counterpart. Particularly, well-designed carbon materials, with specific pore size distribution and 1/2/3-dimensionally inter-connective pores, have been identified as being among the most attractive electrode materials. Hierarchically porous carbonaceous materials have plenty of advantages for CS applications: 1) Interconnecting voids maximize the active sites of chemical reactions and shorten the diffusion paths for ions and/or electrons, facilitating electrolyte transport and infiltration (Li et al., 2020b; Liu et al., 2020). 2) The nanometer-sized edge regions form small lattices and grain boundaries, thus improving the electrochemical capacitance of the carbonaceous material (Pang et al., 2021). 3) Active ordered nanostructures have high electrical conductivity and maintain better mechanical stability during the cycle (Dubey et al., 2020; Zhu et al., 2020). 4) Porous structure can be used as a host material, allowing high-capacitance guest material to be evenly dispersed in the pores (Dong et al., 2018; Ma et al., 2019; Tang et al., 2020; Zhang et al., 2020c). Besides, fine nanopore structure design and internal size distribution of the electrode materials are also key to effectively utilize specific surface area and further improve the capacitive performance. It is mainly supposed that the existence of tiny micropores in carbon matrix can highly add the specific surface area that plays an important role in improving specific capacitance. Nevertheless, the micropores with intricate channels and porous structures are not always advantageous to the electrochemical express of carbon electrodes. Some works have reported that micropores could contribute to parts of specific capacitance via the desolvation of electrolyte ions at relatively low rates, while these pores are not available at a higher discharge current density, thus causing poor rate performance (Eliad et al., 2005; Tian et al., 2021). That is to say, the achievement of capacity is determined by the accessible and effective surface area of the porous carbon materials, while the absorbing and releasing behavior of charges at the interface is related to the intrinsic pore structures of the electrode materials. Moreover, the introduction of heteroatoms (B, N, O, P, etc.,) into carbon substrate has vast effects on the capacitive performance in both organic and inorganic electrolyte ions (Liu et al., 2022; Zhang et al., 2022). According to previous investigation, heteroatoms doping can change the crystalline structures and electron delocalization of the carbon materials by improving chemical stability, electrical conductivity as well as electron–donor characteristics, which can even lead to pseudocapacitive reactions.
Many studies have reported the successful conversion of various precursors like biomass and polymer into 1/2/3-dimensional carbon-based functional materials with reconstructive micro-/nanostructures and tunable surface chemistry properties through physical/chemical methods. In this mini review, we first demonstrate three types of reaction mechanisms and some important intrinsic characteristics for well-designed porous carbon-based materials. Then, we discuss the latest progress of preparing porous carbon at 1/2/3- dimension and their electrochemical performance in CS field. The unique properties and limitations related to different dimensions were discussed, and the benefits of these characteristics for SCs applications were highlighted. Finally, the present challenges and future development trends of advanced electrode materials are prospected.
The deposition and removal of electrostatic charges in EDLCs are caused by the accumulation and withdrawal of dynamic electrons in both the cathode and anode, which in turn is elicited by applied potentials across the entire device. The negatively and positively charged surfaces are counterbalanced by seizing counter-ions from the electrolyte solution, generating a dynamically balanced double layer of opposite charges, with no electrons transmitted between the layers during the charging/discharging processes (Bleda-Martínez et al., 2005). Currently, almost all commercial SCs use carbon for charging and discharging of double layer. The electrochemical capacities of these systems are directly related to the electrostatic charges of counter-ions at the electrode/solution interface without charge transmission, which is a desirable capacitance process (Figure 2) (Bokhari et al., 2020). Due to the low cost, availability, and variety of precursor materials, AC is still the most widely used carbon material in SC application. The electric energy comes from an electrochemical double layer, compared directly with a traditional parallel plate capacitor. When the electrode surface charge is excessive or insufficient, reversely charged ions in the solution accumulate or disperse close to the electrode/electrolyte interface to maintain electrical neutrality.
FIGURE 2. Working mechanism of EDLCs (Bokhari et al., 2020).
In the case of CSs, the electrochemical double-layer behavior on the surface of porous carbon electrode is much more complicated than that on infinitely planar surface. Actually before 2005, enlargement of specific surface area was viewed as the only effective way to enhance the energy storage in EDLC. However, after 2005, the significance of pore size distribution and nanoengineering of carbon was regarded as a quite vital project in the electrochemical performance (Eliad et al., 2001; Eliad et al., 2005). The size of the aperture greatly affects the ability of ions to enter the aperture because it, if too small in that case, is inaccessible and cannot devote to the double-layer capacitance (Jiang et al., 2012). The microscopic thickness of the double layer relies on the size of solvated ions and the concentration of the electrolyte.
According to the equation:
Another type of SC is PC, which appears on electrodes when the exertion of a potential generates faradaic current caused by electrosorption reactions that occur during chemisorption of electron-rich anions (e.g., B− and I−) (Yan et al., 2019; Ghotbi et al., 2021) or by the oxidation/reduction active substances (e.g., Co3O4, Nb2O5, RuO2, and other transition metal oxides) (Liu et al., 2008; Luo et al., 2017; Ma et al., 2019; Zhu 2022). In this electroadsorption reaction: M + A−⇌ MA(1−δ) + δ e−, A− anions on the electrode surface and the quantity of transferred δ e− are intensively relevant to the so-called “electroadsorption valence.” Besides, the exchange of charge across the double layers, rather than dispersing the charge statically over a restricted distance, leads to a reversible oxidation/reduction reaction (Oad + δ e− ⇌ Red). In this reaction, the amount of energy stored owing to δ e− transfer is obviously higher than EDLCs, even paralleled to that of a battery in some ways, because not only the electrode surface but also the interior electrode bulk are partially involved in charge storage (Figure 3). However, the slower reaction kinetics could lead to a loss of power density, and the cycling life of PCs is generally inferior to that of EDLCs due to structural changes caused by reversible reactions. Hybrid composite electrodes combining carbon with pseudocapacitive materials are promising to solve these problems, where carbon framework enhances the whole electrochemical performance of composite electrodes and the pseudocapacitance is attributed to conductive polymers or metallic oxides/hydroxides. In addition, in many reported porous carbon-based systems (Figure 4), electrosorption reactions arising from functional groups/heteroatoms greatly improve the pseudocapacitance characteristics of electrode materials. It is widely known that two different storage mechanisms, double-layer storage and Faraday capacitive behavior, always coexist in CS systems during charging/discharging. Generally, one storage mechanism is dominant and the other is weaker, depending on the nature of the material itself and the speed of the sweep. The comparison in terms of electrode materials, charge storage mechanism, and merits/shortcomings between EDLCs and PCs is shown in Table 1.
FIGURE 3. Energy storage mechanism demonstration and typical compounds: (A) pseudocapacitive-type materials and (B) battery-type materials (Zhu 2022).
FIGURE 4. Carbon materials with diverse morphologies doped with different heteroatoms at various content levels widely used in SCs (Feng et al., 2021).
TABLE 1. Comparison of different characteristics of several types of SCs (Li et al., 2019a).
AHCs are more progressive sort of SCs that can make up for the defects of limited energy/power density, cycle stability, and operating voltage of symmetric devices. Asymmetric systems are defined rather loosely, referring to any feasible chemical–physical (active mass ratio or thickness) or material distinctions of cathodes and anodes. Due to the synergistic combination of the working potential and electrochemical performance of individual electrodes, asymmetric system has extended working potential, better energy, and power density. For example, the asymmetric hybrid system in Figure 5 using battery-type cathodic materials and double-layer anodic materials shows high power and cyclic stability governed by the activated carbon electrode and high capacitance attributed to the faradaic electrode. The combination of double-layer carbon materials and pseudocapacitive materials is the most widely used to date (Yang and Zhou 2017; He et al., 2019; Chen et al., 2020), taking advantages of the best range of electrochemical activity related to each material for comprehensively improving the electrochemical performance of the whole device.
FIGURE 5. Illustration of electrochemical reaction process of NMMO/AC hybrid supercapacitor during charge and discharge (Zhao et al., 2020).
The main factors to be considered for AHC device design are the following: (1) electrode activity mass matching: m+/m− = (C+∆V+) / (C−∆V−) and the total capacitance calculation, where m (g) and V are the mass and working potential of cathode/anode, respectively, and C is the specific capacitance (F); (2) the Coulombic efficiency based on charge storage of two electrodes: Q+ = C+V+ and Q− = C− V−; (3) the working potential V = V+ + V−. V is usually restricted by the decomposition of the electrolyte or excessive oxidation/reduction of either electrode material. Therefore, using these equations, AHC device configurations can be designed in a better way.
CSs mainly rely on the accumulation of charges at the electrode/electrolyte interface to store energy. Therefore, porous carbon used as electrode materials not only needs to provide an abundant nanometer-scale platform conducive to charge contact but should also have a well-designed pore structure beneficial for electrolyte wetting and rapid ion transfer. In addition, various organic/inorganic functional groups on the surface of carbon substrate also have a great influence on the electrochemical performance of SCs.
According to the double-layer theory, a large surface area is required for a carbon-based electrode to achieve large capacitance with a large number of charged ions accumulating on the pore surface. The microporous structure plays a crucial role in high-energy storage by providing enough movement space by means of molecular sieve separation and controlled mass diffusion (Li et al., 2020a; Pang et al., 2021). For instance, AC-based materials have long been the priorities for SC electrodes due to their superior microporosity. These materials are usually derived from pre-carbonization process of multifarious detail carbon-rich precursors like porous polymer precursors (Lei et al., 2019; Lu et al., 2019; Lei et al., 2020) and consequent physical/chemical activation reaction to fabricate microporous structures. Physical activation is the most commonly employed preparation method for AC in large-scale industrial production. As is shown in Figure 6, the redox reaction between carbonaceous materials and activator like CO2, O2, and water steam (Nandi et al., 2012; Pallarés et al., 2018) occurs at a high temperature (700–1,300°C), leaving behind abundant pore structures on the surface and inside of carbon material, while chemical activation shown in Figure 7 refers to heat treatment process with chemical substances (KOH, H3PO4, ZnCl2, K2CO3, etc.,) at a relatively low temperature, forming rich pores and channels through a series of crosslinking or polycondensation reactions (A and Hegde, 2015; Li et al., 2015).
FIGURE 6. Carbonization of PAN monolith with CO2/air or air as activator (Nandi et al., 2012).
FIGURE 7. Activation process on ACs from fallen leaves by KOH and/or K2CO3 (Li et al., 2015).
Numerous experiments have showed that the electrical double-layer capacitance of most electrodes and their specific surface area is not a linear relationship, and the reasons may be that 1) when fabricating, different raw materials, activation process, and post-treatment methods will affect the structure of porous carbon. Even if the obtained specific surface area is similar, the efficiency of forming an electric double-layer may also be diverse; and 2) the size of ions in different electrolyte systems is obviously distinctive, especially hydrated ions and organic ions with larger diameters are difficult to enter micropores, and partial electrolyte ions are trapped as ineffective “dead mass” in the interior pores and channels. Therefore, the minimum micropore size that can be effectively utilized by the electrode will result in different actual capacitance.
The purpose of adjusting the pore diameter of carbon material is to transport adequate electrolyte ions into the electrode so that the carbon surface can be fully utilized. Kornyshev’ group (Kondrat et al., 2012) analyzed the relationships among energy density, pore size, and voltage window (Figure 8). Collectively, at a certain pore size, the maximum energy density increases with the increasing opening voltage, and it reaches saturation at a high voltage when the pore could not continue to accommodate additional electrolyte ions. This saturation energy density increases (at high voltages) as the pores get larger because more charges can be stored. Thus, large pores are desirable at high voltages. However, at low voltages, the energy density for large pores is smaller because an electro-neutral region is formed at the center of the large pore, not contributing to energy storage.
FIGURE 8. (A) Energy density of carbon materials with different pore sizes depends on the potential window. (B) The optimal pore size increases with increasing operating voltage window and (C) passes a maximum (Kondrat et al., 2012).
Hierarchical porous architectures composed of interconnecting micro/meso/macro-pores are more preferable for high-performance CSs, considering the co-evolutionary effect of ample micropores as ion adsorption sites and interconnecting meso/macro-pores to ensure rapid ion transport to achieve significantly synergistic improvement of energy/power density. Precisely, the tiny micropores (<0.5 nm) mainly help to form a huge aggregation platform through the molecular sieve separation and controlled ion-diffusion, while the meso- (2–50 nm)/macropores (>50 nm) serve as shortcuts for ion diffusion to the precise micropores, which can minimize the transport path and interface resistance of electrolyte as shown in the model diagram in Figure 9 (Yang and Zhou 2017). Moreover, the wide-open and interoperable pore structures intensify the successive ion-diffusion dynamics within the hollows, and then they provide achievable possibilities of designing internal construction for compact devices with exceptionally large volume capacity. Therefore, the focus of current research is to explore high-quality precursors and develop simple and efficient preparation methods to achieve the overall control of the pore size distribution of carbon materials.
FIGURE 9. NPC electrodes of symmetric SCs rich in micropores/mesoporous/macropores (Yang and Zhou 2017).
The surface characteristics of carbon materials have vast effects on their capacitive behavior, such as the wettability, interface resistance, and self-discharge behavior. These properties are regularly relevant to impurity residues and functional groups on the surface of carbon matrix during synthesis. The electrochemical performance of the carbon-based electrode was improved via doping the carbon materials with heteratomic (B, N, O, P, etc.,) functions (Gao et al., 2019; Hu et al., 2019; Li et al., 2021). Carbon backbones doped with heteroatoms show great upgrades to the electrochemical performance of CSs. Taking the classical N-doping in graphene as an example (Figure 10), different doping sites have great influences on electrochemical properties of carbon matrix. First, miscellaneous heteroatoms are bound to the carbon substrates to generate positive partial charges on adjacent carbon atoms/heteroatoms with low Sanderson electronegativity, exposing polarized electrode surfaces, and these active sites promote their interactions with electrolyte ions and further boost adsorption. Second, heteroatoms act as electron donors to replenish lone pair electrons into the delocalized carbon matrix for enhancing intrinsic conductivity, and to lower the valence band, contributing to the long-term operation at the Fermi level and high electron cloud density. Third, the embeddedness of pseudo-active heteroatoms is beneficial to proton adsorption and enables bare carbon to possess significant Faraday pseudocapacitance due to electrochemical redox reaction.
FIGURE 10. Examples of distinctive N-containing functional groups in graphene (Yang and Zhou 2017).
As is shown in Figure 11, doping designs in carbon-based materials are multitudinous. The co/multi-doping of disparate heteroatoms (Deng et al., 2021) may induce collaborative contributions caused by spin and charge reallocation, which is beneficial for the dense electron activity density and hence enhances the overall electrochemical representation. However, excessive or highly active heteroatom doping under immature preparation methods and conditions may bring about the unformed porosity or skeleton collapse of the functionalized carbon, hence causing decayed performances. Therefore, plenty of research efforts emphasize on matching the suitable preparation methods and conditions according diverse precursor features to realize the synergistic advancement of heteratomic doping and porous structure. Functionalized carbon is usually prepared by special post-processing of the as-prepared carbon material or self-doping through thermal decomposition of heteroatom-rich precursors such as biomass sources and organic salts. The self-doping strategy is highly praised for allowing uniform distribution of heteroatoms and multiple species, whereas fairly limited to the thermodynamic stability of the reaction process and the structural stability of the precursors in contrary to widely used post-treatment methods.
FIGURE 11. Illustration of different doping designs in graphene (Hu et al., 2019).
In recent years, with explosive in-depth study, self-doping strategies have been subdivided into adding additional dopants (like melamine and phosphoric acid) to carbon precursors prior to pyrolysis, or directly pyrolyzing organic salt/polymer precursors composed of building blocks containing heteroatoms. Complementary dopants can not only supply a large number of exterior atoms but also act as catalysts, activators, or structurally oriented agents. Another ideal way to develop highly doped functionalized carbons is through straightforward pyrolysis of desirable metal organic framework (Chen et al., 2019), polymer precursors (Lei et al., 2019), etc.
A large surface area is regularly advantageous for carbon electrodes as abovementioned, whereas the electrode capacitance does not always amplify along with increasing surface area because the electrolyte ions are not necessarily in full contact with all tortuous pore alleys and weeny micropore, especially when the surface area surpasses 1,500 m2 g−1. Constructing plentiful active adsorption sites on surface morphology will affect the ionic diffusion path, help alleviate the surface difficult-to-reach barrier to promote charge storage, and improve the structural stability. Great research efforts have accordingly focused on constituting multifarious carbon morphologies with the delicate control over the surface topography and internal texture. In general, carbon nanostructures with different morphologies, like one-dimensional (1D) nanofibers (Cai et al., 2016), nanotubes (Li et al., 2020b); two-dimensional (2D) nanosheets (Ran et al., 2021), graphene (Gopalakrishnan and Badhulika 2018); three-dimensional (3D) hierarchical porous (Liu et al., 2016), and core-shell structures (Zhu et al., 2020) have been extensively studied as high-performance CS electrode materials. These specially designed layered/hollow porous nanostructures have the advantages of more electroactive sites, ideal porosity, and larger electrode/electrolyte interfaces, which endow appreciable electrochemical performance.
The fiber structure has a high Young’s modulus (138 GPa) and strength (∼2–3 GPa), providing a great mechanical foundation for the progress of high-strength and even free-standing electrodes or membranes. At the same time, the 1D linear channel can offer a direct electron transfer path as opposed to a granular or spherical electrode (Hwang et al., 2020; Zhou et al., 2020). Hollow tubular structures can be regarded as electrolyte reservoirs with higher specific surface areas and more active sites (Cao et al., 2018; Yang et al., 2019). Therefore, 1D fibrous or tubular porous carbon materials are considered the most feasible electrode form and have been fabricated by various strategies for SCs.
Biomass precursors are the main source of fiber carbon with extensive availability, reproducibility, and low toxicity. Significantly, their inherent uniformity and fine biological structure are the natural advantages of preparing electrode materials with well-defined and controllable morphologies; the abundance of basic elements such as C, S, N, and P in living organisms endows biomass-derived carbon ideal surface properties. Cao et al. (Cao et al., 2018) reported the preparation of hierarchical porous N-doped carbon microtubules by low-temperature pre-carbonization and KOH chemical activation (Figure 12). The millimeter-long tubular carbon material provides ultra-long electron and ion channels, while a large number of nanoholes in the fiber walls generated by KOH activation allow ions to move quickly through the walls, enhancing the surface area for charge accumulation. In this work, as the electrode materials for SCs, the hollow carbon microtubes treated with 6 M KOH at 650°C (HCMT-650) show high-capacitance (292 F g−1) under the current density of 1 A g−1 and outstanding rate capability up to 10 A g−1. Kapok has the highest hollow rate (97%), providing greater available surface and shorter transport pathway. However, the naturally thin wall (only 0.74 mm) is prone to rupture by coinstantaneous activation and carbonization at a high temperature over 500°C. In order to preserve the natural hollow structure of kapok as much as possible, the preparation of kapok-derived hollow carbonaceous microtubules by pre-stabilization and simultaneous activation-carbonization with (NH4)2HPO4 is a feasible method. (NH4)2HPO4 is not only a poring agent and N/P source but also a critical cross-linking agent to fix the original structure and protect the hollow morphologies of kapok, improving designed carbon yield. SCs assembled by HCMT-650 electrode in 1 M TEABF4/PC electrolyte showed a great rate performance of 140 F g−1 at the current density of 1.0 A g−1 with 82% capacitance retention and large energy/power delivery of 48.3 W h kg−1 and 27 kW kg−1, respectively. These results indicate that the original porous tubular structures have superior morphologies for promoting both the rate performance and long lifespan of SCs. Therefore, the capacitive behavior of traditional AC with poor ionic dynamics can be prominently improved by appropriately designing the structure.
FIGURE 12. Schematic representation of the synthesis procedure for HCMTs (Cao et al., 2018).
In addition, some polymer precursors have also been investigated to fabricate nanotubes of polypyrrole (Zhang and Manohar 2005), polyaniline (Zhou et al., 2009), and covalent organic frameworks (Gole et al., 2018) through template-free self-assembly or soft /hard-template technologies. Yang’s group reported a hydrothermally self-templated synthesis process of polyimide nanotubes with a submicron-sized rectangular section. As is shown in Figure 13, naphthalene-1,4,5,8-tetracarboxylic dianhydride (NTCDA) was first hydrolyzed to its corresponding acid, and then, it interacted with 1,3,5-tris(4-aminophenyl) benzene (TAPB) by an acid–base reaction in water. The monomer salt precipitate obtained was dispersed in purified water and substantially transferred to a high-pressure reactor for hydrothermal polycondensation. More interestingly, after the pyrolysis of polyimide nanotubes at 800°C in argon, the hollow morphology of N-doped carbon tubes could be well preserved. The SCs in this work display specific capacitances of 147 and 110 F g−1 at the current density of 1.0 and 20 A g−1, respectively. The high capacitance retentions (about 75%) after a 20-fold rate increase indicate a high rate capability. The energy density of the whole device is 17.2 Wh kg−1 and 13.3 Wh kg−1, corresponding to the power density of 500 W kg−1 and 20,000 W kg−1, respectively.
FIGURE 13. Schematic illustration of (A) two-step synthetic routes and (B) the proposed formation mechanism of polyimide tubes (Lei et al., 2020).
Recent studies have reported that hybrid materials formed by combining graphene fibers and carbon nanotubes (CNTs) can boost the electrical and thermal conductivity, mechanical flexibility, and charge transmission benefiting from their strong π–π stacking. This phenomenon gives rise to an increase in conductivity of 450 ± 20 S cm−1, tensile strength of ∼630 M Pa as well as specific capacitance of ∼31.5 F g−1. Cheng’s group loaded CNTs on the substrate of two-dimensional graphene sheets by CVD process (Figure 14), and constructed CNTs-G hybrid fiber electrodes with great areal capacitance (1.2–1.3 mF cm−2) and excellent flexibility (the CV behavior is still unchanged even after 200 times of bending) (Cheng et al., 2013). Considering the risk of short circuit caused by exposed fiber electrodes, Kou et al. synthesized polyelectrolyte-coated graphene-nanotube sheath-core composite fibers by coaxial wet spinning assembly. The polyelectrolyte sheath can effectively provide the short-circuit protection in the hybrid fibers (Kou et al., 2014).
FIGURE 14. Diagram of preparation process: (A) GO+Fe3O4 in glass pipeline; (B) G/Fe3O4 fiber and (C) CNT/G fiber (Cheng et al., 2013).
However, the performance of these carbon-based nanofibers/tubes or hybrid fiber electrodes is still difficult to meet people’s increasing demand for high energy density. Hence, exploring 1D carbon materials combined with pseudocapacitive materials, such as Nb2O5 embedded strategy and RuOx(OH)y redox type, is of great significance to improve the energy density of SCs. The 1D tubular structure, especially CNTs, usually serve as an ion-buffering tank to promote the storage of charge on the inner surface and cut down the ionic diffusion path. Also, with good thermodynamic, electrical, and mechanical traits as well as surface properties, it is an ideal substrate material for filling inorganic species into internal tubular structures or attaching to the outer surface of CNTs. However, due to the weak and unclear interactions between CNTs and different inorganic species, uneven distribution of nanoparticles is a thorny problem; so, a new strategy for efficient combination of CNTs and functional materials remains a challenge.
Two-dimensional (2D) carbon materials with rich sp2 hybridization possess the following merits: 1) The powerful in-plane covalent bond properties endow 2D-layered structures with large in-plane conductivity, which can speed up the electrons transfer in the plane (Gopalakrishnan and Badhulika 2018). 2) The special single/few-atom layered structure with nanoscale thickness and widely open plane not only provides a vast specific surface area and abundant active sites for great capacitance performance but also increases high electrode/electrolyte contact interfaces, decreasing ion-diffusion resistance for excellent power achievement (Bi et al., 2019; Zhang et al., 2020a). 3) For 2D graphene or carbon nanosheets with hierarchical pore structure, the internal incompact space can heavily buffer the volume expansion during the charging/discharging process, so as to achieve long-period stability (Li et al., 2019b). In recent years, graphene-like materials with representative 2D structures have been widely investigated as SC electrodes with appreciable capacitance performance.
For instance, Shao et al. reported a simple and cost-effective synthesis method of 2D-layered porous N-doped O-rich carbon nanosheets (NOCN) which is shown in Figure 15 (Ran et al., 2021). First, 4MgCO3·Mg(OH)2·5H2O (MCB) was inserted into the mixture precursor of urea and citric acid via dissolution and recrystallization. In the pyrolysis process, the intermediate products are evenly stripped by the gaseous substance, forming a 2D nanosheet structure. At the same time, the fabricated MgO and N atoms were in situ enclosed into the carbon framework, respectively. The optimized NOCN900 materials possess unique 2D lamellar features, high SBET of 1804.2 m2 g−1 as well as hierarchical pores. It deliveries a large specific capacitance (232 F g−1 at the current density of 0.5 A g−1) and a great capacitance retention (71.5% at the current density of 20 A g−1) in KOH electrolyte solution, which can be attributed to the fast ion diffusion conferred by the 2D architecture and the pseudocapacitance contribution from N/O co-doping group. The as-assembled SCs display the maximum energy density of 17 Wh kg−1 and maximum power density of 9000 W kg−1 in Na2SO4 neutral electrolyte. Also, it still maintains 92% capacitance even after 10,000 charge and discharging cycles, indicating its desirable cycling stability. This study provides a sustainable and cost-efficient approach for the application of 2D porous carbon electrode in the field of energy storage.
FIGURE 15. Schematic illustration of the synthetic procedure for NOCN (Ran et al., 2021).
In addition, numerous 2D graphene-like carbon nanosheets have been widely fabricated by high-efficiency processes using sustainable and inexpensive biomass as raw materials. Owing to their simple and economical manufacturing methods, these materials display an excellent potential for future CS development of 2D electrodes. Badhulika’s team studied a new peeled cucumber carbon source to prepare economic carbon nanosheets through controllable green synthesis strategies of carbon hydrogel and aerogel intermediate products (Figure 16) (Gopalakrishnan and Badhulika 2018). Compared with other traditional biocarbon synthetic strategies, this unique synthesis method is more convenient and environment-friendly. Carbon-based hydrogels and aerogels here are synthesized completely green, without the introduction of any toxic and expensive chemicals, while avoiding the usage of additional porogens such as KOH, ZnCl2, and CaO. In this context, the as-obtained graphene-like carbon flakes with numerous edge active sites have a capacitive performance of 143 F g−1 in three-electrode structure of the aqueous electrolyte, and the capacity retention rate of the nanosheet electrode is still up to 97% even after 1,000 charging/discharging cycles.
FIGURE 16. Schematic diagram for the synthesis route of carbon nanosheets from cucumber (Gopalakrishnan and Badhulika 2018)
Although the introduction of 2D graphene and its analogues aforementioned helps to promote the specific capacitance and cycling stability of the electrode, the irreversible restack and agglomeration of graphene layers during charge and discharge can affect their hierarchical porous architecture, thus impeding ion transport and electrolyte entry into the carbon network, further resulting in deterioration of the electrode performance. In addition, electrochemically active materials are difficult to be firmly embedded on flat graphene sheets, and inert additives and binders are always required, which can greatly affect the overall performance of the final electrode.
In contrast, 3D graphene frameworks with interpenetrating microstructures have displayed a great potential to achieve high-performance SCs. The unique 3D shape, high porosity structure, and excellent specific surface area not only supply an accessible region for electrolyte diffusion, a shortcut for charge migration, but also offer an excellent platform for the loading of active materials. Due to their superior electrical, physical, and mechanical manifestations, these 3D graphene frameworks can be directly used as non-binder, flexible, and free-standing SC electrode materials, which will achieve higher specific capacities, strong rate abilities, and reliable cycle performance (Zhou et al., 2019; Van Ngo et al., 2020; Zhang et al., 2020b; Yao et al., 2021). Nowadays, a variety of methods for synthesizing have been covered, such as hydrothermal method, templated method, chemical vapor deposition, freeze drying, and chemical crosslinking.
Liu et al. (Liu et al., 2022) fabricated polypyrrole nanoarrays (PPy NAs) via an oversimplified electrochemical deposition technique (Figure 17). O and N co-doped 3D carbon nanoarrays (D-CNAs) were carbonized to promote the electrical conductivity of the materials and enhance the charge storage capacity of EDLCs. In addition, the synergistic impact of O and N co-doping as well as the exposure of electrochemical active sites in 3D nanoskeletons reduces the charge transfer resistance, thus making D-CNAs a strong pseudocapacitance effect. Using pollution-free and resource-rich NaCl solution as the electrolyte, the capacitance of D-CNAs electrode can reach 480 F g−1 at 1 A g−1 with 77.1% capacity retention at 10 A g−1. The as-assembled symmetric D-CNA SCs have good cycle stability and great capacitance retention of 90% after 10000 cycles at 10 A g−1.
FIGURE 17. Growth process of carbon paper-based NAs: (A) ionization and adsorption of pyrrole and TsOH in an aqueous solution; (B) under an electric field, pyrrole and TsOH adsorbed each other on the carbon paper, resulting in an oriented arrangement and oxidized chain, finally forming nucleation growth sites; (C) electrodeposition of PPy NAs; and (D) carbonization to obtain D-CNAs (Liu et al., 2022).
Zhang et al. reported a 3D-printed porous carbon aerogel with ultrahigh surface area, which is prepared through the direct ink writing (DIW) approach using cellulose nanocrystal (CNC)–based ink in Figure 18. CNCs are combined with a silica microsphere suspension to fabricate a uniform and highly viscous ink, exhibiting shear thinning characteristics owing to H-bond fracture. CNCs are desirable materials for 3D printing attribute to their accessibility in nature and the peculiar properties in aqueous solution. First, abundant hydroxyl groups (six per unit) in cellulose molecule are bonded with water to form strong hydrogen bond, which greatly increases the water retention rate of cellulose matrix in aqueous solution even up to 98%. Second, nanocellulose can also work as a surfactant, assisting in the dispersion of various other materials in aqueous solution due to its extremely negative ζ potential (ca. −60 mV). Third, the high Young’s modulus of nanocellulose (ca.150 GPa) enables it to retain the original structure after drying and annealing. The prepared 3D-printed multiscale porous carbon aerogels have an open porous structure and excellent electrochemical properties at ultralow temperatures (−70°C). The assembled SCs in this work exhibit outstanding capacitance of 148.6 F g−1 at the scan rate of 5 mV s−1. More importantly, it still maintains a capacitance of 71.4 F g−1 at a high scan rate of 200 mV s−1, 6.5 times higher than that of the non-3D-printed carbon aerogels.
FIGURE 18. (A) Schematic illustration of the fabrication of 3D-printed multiscale porous carbon aerogels. (B) Digital image of the 3D-printed CNCs/SiO2 aerogels with a shape of “UCSC.” (C) Digital image of the 3D-printed multiscale porous carbon aerogels with different thicknesses (Yao et al., 2021).
Recently, some novel techniques have been reported to explore 3D-nanostructured hybrid composite by combining the 1D-CNTs with 2D-graphene sheets (Balamurugan et al., 2016; Gole et al., 2018). The hybrid 3D-graphene network creatively improves vast specific surface area to covalently anchor other 1D nanomaterials, and this provides a concept of “hierarchy” for carbon nanomaterials. In addition, the connection of CNTs with graphene sheets adds the basal plane spacing distance within graphene layers and also creates more defects that are quite favorable for charge transfer. Although a lot of progress has been made in the exploration of 3D porous carbon electrodes, they still have many restrictions for practical SC application caused by limited capacitance and numerous multi-macro pores which greatly lessen compaction density and result in the low areal/volume energy and power density. To overcome these problems, it is a promising direction to further improve the energy performance of SCs by combining preferable 3D networks with various pseudocapacitive materials to maximize the available specific surface area and realize the whole improvement of electrochemical performance.
In summary, we conclude the recent progress of porous carbon with different microscopic dimensions in CSs. Hierarchical porous carbon materials of different dimensions have various and unique properties, which enables them promising electrodes. As just noted in this mini review, 1D carbon structures have shorter incessant ion/electron transport routes, which is conducive to high rate capacities of CSs. Since the specific surface area of electrode greatly affects the electrochemical capacitance of energy storage device, investigations for improving the free diffusion of ions across the entire electrode and the surface reachability for the electrolyte are of great importance. The merits of 1D-carbon materials can be further amplified when using 2D graphene or carbon nanosheets as electrodes. With the increase of spatiality, the carbon facets having numerous effective electroactive sites are exposed to a large extent in the electrolyte solution, which can greatly promote overall performances of SCs. Thus, 3D-carbonous materials with interpenetrating microstructures have become one of the most promising candidate electrodes for the applications of high-performance CSs. Although many progresses have been made in recent years in the preparation of hierarchical porous carbon with different pore size distributions, dimensions, and surface properties for high-performance CSs, there are still some problems to be solved in the future:
1) Although SCs have the advantages of fast charging and discharging process and long circular life, they are still restrained by relatively low electrochemical capacitance, incomplete accessibility of active sites, and narrow operating voltage (the potential windows of the whole devices mentioned in this mini review (EDLCs and PCs) are all less than 2 V), significantly affecting the improvement of energy density and power density. In order to obtain high energy/power density, the design of novel electrode of carbon matrix composite material is an important direction for the development of SCs in the future. For example, carbon networks are combined with pseudocapacitive material to expand the working voltage, so as to further improve the capacitance of SC device. For pseudocapacitive materials, especially transition metal chalcogenides (TMCs), poor electrical conductivity and large charge transfer resistance caused by structure pulverization during cycling are the main factors limiting material properties. Therefore, the rational design of 1/2/3-dimensional carbon materials and TMCs as composite nanostructures to enhance electrochemical kinetics and decrease charge transfer resistance has great challenges. Porous carbon with a suitable pore size distribution could improve the contact between the active substance and electrolyte and reduce the ion transport length (“Different dimensions of porous carbon-based electrode materials for SCs” section). Also, the introduction of conductive polymer can improve the conductivity of TMC electrode and prevent TMC structure damage and dissolution in electrolyte.
2) Flexible carbon-based SCs are an important demand in the market expansion of electronic products. Developing common and effective fabrication strategies to weave them at an appropriate scale remains a challenge. Different dimensions of functionalized carbon can be combined to construct electrode materials for SCs with full use of each building block. These combinations could result in composite performance better than the individual components. In addition, flexible packaging improvements are also significant for the success of desirable flexible energy storage systems. A thinner, watertight/airtight, flexible package that protects electrolytes from bleeding will help improve their overall volumetric energy density and enable them more compatible. For instance, polydimethylsiloxane-based sealing plastics or other stretchable elastomers can greatly satisfy the requirements of mechanical properties. As for flexible electrodes based on biomass, textiles, polymers etc., they can be prepared by printing technology, chemical vapor deposition, electrostatic spinning technology, electrochemical deposition, and other methods. Flexible, stretchable electrodes are usually prepared by coating the electrode materials on stretchable matrix or embedding them in stretchable matrix. However, under strain, the electrochemical performance of flexible devices is often greatly affected and it is difficult to maintain stable cycling. Therefore, how to ensure the electrochemical achievement of flexible devices under limiting strain is still a challenge.
3) The industrial production of new electrode materials still faces some challenges. i) High specific surface area is the main characteristic that affects the capacitance of SCs. With high loading in commercial production, ultra-high specific surface area will inevitably lead to a significant decrease in volume/area energy density. Therefore, how to balance specific surface area and compaction density is a difficult problem. ii) Another factor restricting the industrialization development of novel materials for SCs is energy consumption and the high economic cost caused by the carbonization and activation process of electrode materials. Thus, it is critical to develop new simplified procedures. iii) Blade coating is the most widely used electrode slurry deposition process in large-scale production. However, it is hard to obtain ultra-thin electrodes with special patterns. Technologies currently under development, such as 3D printing and inkjet printing, are desirable options for implementing such electrodes.
YL: conceptualization, investigation, and writing—original draft. HC: writing—review and editing. LL: conceptualization, funding acquisition, supervision, writing—original draft, and writing—review and editing.
The authors declare that the research was conducted in the absence of any commercial or financial relationships that could be construed as a potential conflict of interest.
All claims expressed in this article are solely those of the authors and do not necessarily represent those of their affiliated organizations, or those of the publisher, the editors, and the reviewers. Any product that may be evaluated in this article, or claim that may be made by its manufacturer, is not guaranteed or endorsed by the publisher.
A, D., and Hegde, G. (2015). Activated carbon nanospheres derived from bio-waste materials for supercapacitor applications – A review. RSC Adv. 5 (107), 88339–88352. doi:10.1039/c5ra19392c
Balamurugan, J., Duy Thanh, T., Kim, N. H., and Hee Lee, J. (2016). Facile synthesis of 3D hierarchical N-doped graphene nanosheet/cobalt encapsulated carbon nanotubes for high energy density asymmetric supercapacitors. J. Mat. Chem. A Mat. 4 (24), 9555–9565. doi:10.1039/c6ta03132c
Bi, Z., Kong, Q., Cao, Y., Sun, G., Su, F., Wei, X., et al. (2019). Biomass-derived porous carbon materials with different dimensions for supercapacitor electrodes: A review. J. Mat. Chem. A Mat. 7 (27), 16028–16045. doi:10.1039/c9ta04436a
Bleda-Martínez, M. J., Maciá-Agulló, J. A., Lozano-Castelló, D., Morallón, E., Cazorla-Amorós, D., Linares-Solano, A., et al. (2005). Role of surface chemistry on electric double layer capacitance of carbon materials. Carbon 43 (13), 2677–2684. doi:10.1016/j.carbon.2005.05.027
Bokhari, S. W., Ahmad, H. S., Sherrell, P. C., Yue, X., Maletira Karumbaiah, K., Wei, S., et al. (2020). Advances in graphene-based supercapacitor electrodes. Energy Rep. 6, 2768–2784. doi:10.1016/j.egyr.2020.10.001
Cai, J., Niu, H., Wang, H., Shao, H., Fang, J., He, J., et al. (2016). High-performance supercapacitor electrode from cellulose-derived, inter-bonded carbon nanofibers. J. Power Sources 324, 302–308. doi:10.1016/j.jpowsour.2016.05.070
Cao, X., Tan, C., Sindoro, M., and Zhang, H. (2017). Hybrid micro-/nano-structures derived from metal-organic frameworks: Preparation and applications in energy storage and conversion. Chem. Soc. Rev. 46 (10), 2660–2677. doi:10.1039/c6cs00426a
Cao, Y., Xie, L., Sun, G., Su, F., Kong, Q-Q., Li, F., et al. (2018). Hollow carbon microtubes from kapok fiber: Structural evolution and energy storage performance. Sustain. Energy Fuels 2 (2), 455–465. doi:10.1039/c7se00481h
Chen, C. R., Qin, H., Cong, H. P., and Yu, S. H. (2019). A highly stretchable and real-time healable supercapacitor. Adv. Mat. 31 (19), e1900573. doi:10.1002/adma.201900573
Chen, M., Wang, L., Sheng, X., Wang, T., Zhou, J., Li, S., et al. (2020). An ultrastable nonaqueous potassium‐ion hybrid capacitor. Adv. Funct. Mat. 30 (40), 2004247. doi:10.1002/adfm.202004247
Cheng, F, Yang, X, Zhang, S, and Lu, W (2020). Boosting the supercapacitor performances of activated carbon with carbon nanomaterials. J. Power Sources 450, 227678. doi:10.1016/j.jpowsour.2019.227678
Cheng, H., Dong, Z., Hu, C., Zhao, Y., Hu, Y., Qu, L., et al. (2013). Textile electrodes woven by carbon nanotube-graphene hybrid fibers for flexible electrochemical capacitors. Nanoscale 5 (8), 3428. doi:10.1039/c3nr00320e
Deng, H, Wang, L, Li, S, Zhang, M, Wang, T, Zhou, J, et al. (2021). Radial pores in nitrogen/oxygen dual‐doped carbon nanospheres anode boost high‐power and ultrastable potassium‐ion batteries. Adv. Funct. Mat. 31 (51), 2107246. doi:10.1002/adfm.202107246
Dong, S., Li, Z., Xing, Z., Wu, X., Ji, X., Zhang, X., et al. (2018). Novel potassium-ion hybrid capacitor based on an anode of K2Ti6O13 microscaffolds. ACS Appl. Mat. Interfaces 10 (18), 15542–15547. doi:10.1021/acsami.7b15314
Dubey, P, Shrivastav, V, Maheshwari, P H., and Sundriyal, S (2020). Recent advances in biomass derived activated carbon electrodes for hybrid electrochemical capacitor applications: Challenges and opportunities. Carbon 170, 1–29. doi:10.1016/j.carbon.2020.07.056
Eliad, L, Salitra, G, Abraham, S, and Aurbach, D (2001). Ion sieving effects in the electrical double layer of porous carbon electrodes- estimating effective ion size in electrolytic solutions. J. Phys. Chem. B 105, 6880–6887. doi:10.1021/jp010086y
Eliad, L, Salitra, G, Abraham, S, and Aurbach, D (2005). On the mechanism of selective electroadsorption of protons in the pores of carbon molecular sieves. Langmuir 21, 3198–3202. doi:10.1021/la049238h
Faraji, S, and Nasir Ani, (2015). The development supercapacitor from activated carbon by electroless plating—a review. Renew. Sustain. Energy Rev. 42, 823–834. doi:10.1016/j.rser.2014.10.068
Feng, X, Bai, Y, Liu, M, Li, Y, Yang, H, Wang, X, et al. (2021). Untangling the respective effects of heteroatom-doped carbon materials in batteries, supercapacitors and the ORR to design high performance materials. Energy Environ. Sci. 14 (4), 2036–2089. doi:10.1039/d1ee00166c
Gao, P, Lu, Y, Deng, S, Cui, X, Zhang, Q, Yang, Y, et al. (2019). Simultaneous polymerization enabled the confinement of size‐adjustable TiO2 nanocrystals in S‐doped carbons for high‐rate anode materials. Energy Technol. 7 (9), 1900247. doi:10.1002/ente.201900247
Ghotbi, Y, Javanmard, A, and Hassan, S (2021). Layered nanoreactor assisted to produce B-doped and P-doped 3D carbon nanostructures for supercapacitor electrodes. J. Energy Storage 44, 103514. doi:10.1016/j.est.2021.103514
Gole, B., Stepanenko, V., Rager, S., Grune, M., Medina, D. D., Bein, T., et al. (2018). Microtubular self-assembly of covalent organic frameworks. Angew. Chem. Int. Ed. 57 (3), 846–850. doi:10.1002/anie.201708526
Gopalakrishnan, A, and Badhulika, S (2018). Ultrathin graphene-like 2D porous carbon nanosheets and its excellent capacitance retention for supercapacitor. J. Industrial Eng. Chem. 68, 257–266. doi:10.1016/j.jiec.2018.07.052
He, C, Jiang, Y, Zhang, X, Cui, X, and Yang, Y (2019). A simple glucose‐blowing approach to graphene‐like foam/NiO composites for asymmetric supercapacitors. Energy Technol. 8 (1), 1900923. doi:10.1002/ente.201900923
Hu, C., Lin, Y., Connell, J. W., Cheng, H. M., Gogotsi, Y., Titirici, M. M., et al. (2019). Carbon-based metal-free catalysts for energy storage and environmental remediation. Adv. Mat. 31 (13), e1806128. doi:10.1002/adma.201806128
Hwang, J., Ejsmont, A., Freund, R., Goscianska, J., Schmidt, B., Wuttke, S., et al. (2020). Controlling the morphology of metal-organic frameworks and porous carbon materials: Metal oxides as primary architecture-directing agents. Chem. Soc. Rev. 49 (11), 3348–3422. doi:10.1039/c9cs00871c
Jiang, D. E., Jin, Z., Henderson, D., and Wu, J. (2012). Solvent effect on the pore-size dependence of an organic electrolyte supercapacitor. J. Phys. Chem. Lett. 3 (13), 1727–1731. doi:10.1021/jz3004624
Kondrat, S., Pérez, C. R., Presser, V., Gogotsi, Y., and Kornyshev, A. A. (2012). Effect of pore size and its dispersity on the energy storage in nanoporous supercapacitors. Energy Environ. Sci. 5 (4), 6474. doi:10.1039/c2ee03092f
Kou, L., Huang, T., Zheng, B., Han, Y., Zhao, X., Gopalsamy, K., et al. (2014). Coaxial wet-spun yarn supercapacitors for high-energy density and safe wearable electronics. Nat. Commun. 5, 3754. doi:10.1038/ncomms4754
Lei, S., Cui, X., Liu, X., Zhang, X., Han, X., Yang, Y., et al. (2020). Hydrothermally self-templated synthesis of rectangular polyimide submicrotubes and promising potentials in electrochemical energy storage. Chem. Commun. 56 (9), 1429–1432. doi:10.1039/c9cc09526h
Lei, S., Lu, Y., Zhang, X., Gao, P., Cui, X., Yang, Y., et al. (2019). A molecular engineering approach to pore-adjustable nanoporous carbons with narrow distribution for high-performance supercapacitors. Chem. Commun. 55 (16), 2305–2308. doi:10.1039/c8cc10186h
Li, S. Y., Deng, H. L., Chu, Z. L., Wang, T., Wang, L., Zhang, Q. S., et al. (2021). Fast-charging nonaqueous potassium-ion batteries enabled by rational construction of oxygen-rich porous nanofiber anodes. ACS Appl. Mat. Interfaces 13 (42), 50005–50016. doi:10.1021/acsami.1c15524
Li, T., Ma, R., Lin, J., Hu, Y., Zhang, P., Sun, S., et al. (2019a). The synthesis and performance analysis of various biomass‐based carbon materials for electric double‐layer capacitors: A review. Int. J. Energy Res. 44 (4), 2426–2454. doi:10.1002/er.5061
Li, X., Li, H., Fan, X., Shi, X., and Liang, J. (2020b). 3D‐Printed stretchable micro‐supercapacitor with remarkable areal performance. Adv. Energy Mat. 10 (14), 1903794. doi:10.1002/aenm.201903794
Li, X., Chen, M., Wang, L., Xu, H., Zhong, J., Zhang, M., et al. (2020a). Nitrogen-doped carbon nanotubes as an anode for a highly robust potassium-ion hybrid capacitor. Nanoscale Horiz. 5 (12), 1586–1595. doi:10.1039/d0nh00451k
Li, Y-T, Yu-Tong, P, Lu, L-M, Xu, S-H, and Ren, T-Z (2015). Hierarchical porous active carbon from fallen leaves by synergy of K2CO3 and their supercapacitor performance. J. Power Sources 299, 519–528. doi:10.1016/j.jpowsour.2015.09.039
Li, Z-X., Yang, B-L., Zou, K-Y., Kong, L., Yue, M-L., Duan, H-H., et al. (2019b). Novel porous carbon nanosheet derived from a 2D Cu-MOF: Ultrahigh porosity and excellent performances in the supercapacitor cell. Carbon 144, 540–548. doi:10.1016/j.carbon.2018.12.061
Liu, B, Zhang, H, Yang, Z, Zhang, P, Ji, W, Liu, J, et al. (2022). Facile fabrication of oxygen and nitrogen co-doped 3D-carbon nanoarrays for high performance environmentally friendly wireless charging integration supercapacitor. J. Energy Storage 49, 104082. doi:10.1016/j.est.2022.104082
Liu, X, Xu, W, Zheng, D, Li, Z, Zeng, Y, Lu, X, et al. (2020). Carbon cloth as an advanced electrode material for supercapacitors: Progress and challenges. J. Mat. Chem. A Mat. 8 (35), 17938–17950. doi:10.1039/d0ta03463k
Liu, Y, Zhao, W, and Zhang, X (2008). Soft template synthesis of mesoporous Co3O4/RuO2·xH2O composites for electrochemical capacitors. Electrochimica Acta 53 (8), 3296–3304. doi:10.1016/j.electacta.2007.11.022
Liu, Y., Shi, Z., Gao, Y., An, W., Cao, Z., Liu, J., et al. (2016). Biomass-swelling assisted synthesis of hierarchical porous carbon fibers for supercapacitor electrodes. ACS Appl. Mat. Interfaces 8 (42), 28283–28290. doi:10.1021/acsami.5b11558
Lu, Y, Zhang, Q, Lei, S, Cui, X, Deng, S, Yang, Y, et al. (2019). In situ templating approach to fabricate small-mesopore-dominant S-doped porous carbon electrodes for supercapacitors and Li-ion batteries. ACS Appl. Energy Mat. 2 (8), 5591–5599. doi:10.1021/acsaem.9b00777
Luo, G, Li, H, Zhang, D, Gao, L, and Tong, L (2017). A template-free synthesis via alkaline route for Nb2O5/carbon nanotubes composite as pseudo-capacitor material with high-rate performance. Electrochimica Acta 235, 175–181. doi:10.1016/j.electacta.2017.03.112
Luo, H., Chen, M., Cao, J., Zhang, M., Tan, S., Wang, L., et al. (2020). Cocoon silk-derived, hierarchically porous carbon as anode for highly robust potassium-ion hybrid capacitors. Nanomicro. Lett. 12 (1), 113. doi:10.1007/s40820-020-00454-w
Luo, X, Chen, S, Hu, T, Chen, Y, and Li, F (2021). Renewable biomass‐derived carbons for electrochemical capacitor applications. SusMat 1 (2), 211–240. doi:10.1002/sus2.8
Ma, Y, Hou, C, Zhang, H, Zhang, Q, Hu, L, Wu, S, et al. (2019). Three-dimensional core-shell Fe3O4/Polyaniline coaxial heterogeneous nanonets: Preparation and high performance supercapacitor electrodes. Electrochimica Acta 315, 114–123. doi:10.1016/j.electacta.2019.05.073
Nandi, M., Okada, K., Dutta, A., Bhaumik, A., Maruyama, J., Derks, D., et al. (2012). Unprecedented CO2 uptake over highly porous N-doped activated carbon monoliths prepared by physical activation. Chem. Commun. 48 (83), 10283. doi:10.1039/c2cc35334b
Pallarés, J, González-Cencerrado, A, and Arauzo, I (2018). Production and characterization of activated carbon from barley straw by physical activation with carbon dioxide and steam. Biomass Bioenergy 115, 64–73. doi:10.1016/j.biombioe.2018.04.015
Pang, Z, Li, G, Xiong, X, Ji, L, Xu, Q, Zou, X, et al. (2021). Molten salt synthesis of porous carbon and its application in supercapacitors: A review. J. Energy Chem. 61, 622–640. doi:10.1016/j.jechem.2021.02.020
Ran, F, Yang, X, Xu, X, Li, S, Liu, Y, Lu, S, et al. (2021). Green activation of sustainable resources to synthesize nitrogen-doped oxygen-riched porous carbon nanosheets towards high-performance supercapacitor. Chem. Eng. J. 412, 128673. doi:10.1016/j.cej.2021.128673
Tang, J, Mathis, T, Zhong, X, Xiao, X, Wang, H, Anayee, M, et al. (2020). Optimizing ion pathway in titanium carbide MXene for practical high‐rate supercapacitor. Adv. Energy Mat. 11 (4), 2003025. doi:10.1002/aenm.202003025
Tian, W., Li, Y., Zhou, J., Wang, T., Zhang, R., Cao, J., et al. (2021). Implantable and biodegradable micro-supercapacitor based on a superassembled three-dimensional network Zn@PPy hybrid electrode. ACS Appl. Mat. Interfaces 13 (7), 8285–8293. doi:10.1021/acsami.0c19740
Van Ngo, T., Mahmoud, M., Thanh Tung, T., Campbell, C., and Losic, D. (2020). 329, 135104. doi:10.1016/j.electacta.2019.135104Hybridization of MOFs and graphene: A new strategy for the synthesis of porous 3D carbon composites for high performing supercapacitorsElectrochimica Acta
Wu, N, Xue, B, Pan, D, Dong, B, Wei, R, Naik, N, et al. (2020). Recent advances of asymmetric supercapacitors. Adv. Mat. Interfaces 8 (1), 2001710. doi:10.1002/admi.202001710
Yan, P, Yan, L, Zhao, S, Zuo, Z, Wang, X, Wang, C, et al. (2019). Fluorine-doped graphene/nanosized carbide-derived carbon composites for high-performance supercapacitor. Nano 14 (08), 1950099. doi:10.1142/s1793292019500991
Yang, M., and Zhou, Z. (2017). Recent breakthroughs in supercapacitors boosted by nitrogen-rich porous carbon materials. Adv. Sci. (Weinh). 4 (8), 1600408. doi:10.1002/advs.201600408
Yang, Z., Tian, J., Yin, Z., Cui, C., Qian, W., Fei, W., et al. (2019). Carbon nanotube- and graphene-based nanomaterials and applications in high-voltage supercapacitor: A review. Carbon 141, 467–480. doi:10.1016/j.carbon.2018.10.010
Yao, B., Peng, H., Zhang, H., Kang, J., Zhu, C., Delgado, G., et al. (2021). Printing porous carbon aerogels for low temperature supercapacitors. Nano Lett. 21 (9), 3731–3737. doi:10.1021/acs.nanolett.0c04780
Yin, J, Zhang, W, Alhebshi, N A., Numan, S, and Alshareef, H N. (2020). Synthesis strategies of porous carbon for supercapacitor applications. Small Methods 4 (3), 1900853. doi:10.1002/smtd.201900853
Zhang, R, Zhang, W, Shi, M, Li, H, Ma, L, Niu, H, et al. (2022). Morphology controllable synthesis of heteroatoms-doped carbon materials for high-performance flexible supercapacitor. Dyes Pigments 199, 109968. doi:10.1016/j.dyepig.2021.109968
Zhang, S., Liu, H., Yu, J., Li, B., and Ding, B. (2020a). Multi-functional flexible 2D carbon nanostructured networks. Nat. Commun. 11 (1), 5134. doi:10.1038/s41467-020-18977-6
Zhang, X. F., Cui, X., Lu, C-H., Hong, L., Zhang, Q., He, C., et al. (2020b). Conjugated polyimide-coated carbon nanofiber aerogels in a redox electrolyte for binder-free supercapacitors. Chem. Eng. J. 401, 126031. doi:10.1016/j.cej.2020.126031
Zhang, X., and Manohar, S. K. 2005. Narrow pore-diameter polypyrrole nanotubes. J. Am. Chem. Soc. 2005 (127): 14156–14157. doi:10.1021/ja054789v
Zhang, Y., Hu, Y., Wang, Z., Lin, T., Zhu, X., Luo, B., et al. (2020c). Lithiation‐induced vacancy engineering of Co3O4 with improved faradaic reactivity for high‐performance supercapacitor. Adv. Funct. Mat. 30 (39), 2004172. doi:10.1002/adfm.202004172
Zhao, X, Li, G, Feng, M, and Wang, Y (2020). Semi-continuous electrochemical extraction of lithium from brine using CF-NMMO/AC asymmetric hybrid capacitors. Electrochimica Acta 331, 135285. doi:10.1016/j.electacta.2019.135285
Zhou, C, Han, J, and Guo, R. 2009. Synthesis of polyaniline hierarchical structures in a dilute SDS:HCl solution- nanostructure-covered rectangular tubes. Macromolecules, 42:1252–1257. doi:10.1021/ma802191n
Zhou, J., Chen, M., Wang, T., Li, S., Zhang, Q., Zhang, M., et al. (2020). Covalent selenium embedded in hierarchical carbon nanofibers for ultra-high areal capacity Li-Se batteries. iScience 23 (3), 100919. doi:10.1016/j.isci.2020.100919
Zhou, W., Du, Y., Zeng, J., Liu, F., and Zhu, Y. (2019). A modified "gel-blowing" strategy toward the one-step mass production of a 3D N-doped carbon nanosheet@carbon nanotube hybrid network for supercapacitors. Nanoscale 11 (16), 7624–7633. doi:10.1039/c8nr10348h
Zhu, F, Liu, W, Liu, Y, and Shi, W (2020). Construction of porous interface on CNTs@NiCo-LDH core-shell nanotube arrays for supercapacitor applications. Chem. Eng. J. 383, 123150. doi:10.1016/j.cej.2019.123150
Keywords: supercapacitors, energy storage mechanisms, electrode–electrolyte interface, electric double-layer, porous carbon, different dimensions
Citation: Liu Y, Chen H and Li L (2022) Applications and challenges of porous carbon with different dimensions in supercapacitors—a mini review. Front. Energy Res. 10:951701. doi: 10.3389/fenrg.2022.951701
Received: 01 June 2022; Accepted: 30 June 2022;
Published: 24 August 2022.
Edited by:
Wenli Zhang, Guangdong University of Technology, ChinaReviewed by:
Dhanasekaran Vikraman, Dongguk University Seoul, South KoreaCopyright © 2022 Liu, Chen and Li. This is an open-access article distributed under the terms of the Creative Commons Attribution License (CC BY). The use, distribution or reproduction in other forums is permitted, provided the original author(s) and the copyright owner(s) are credited and that the original publication in this journal is cited, in accordance with accepted academic practice. No use, distribution or reproduction is permitted which does not comply with these terms.
*Correspondence: Liqing Li, bGlxaW5nbGlAY3N1LmVkdS5jbg==
Disclaimer: All claims expressed in this article are solely those of the authors and do not necessarily represent those of their affiliated organizations, or those of the publisher, the editors and the reviewers. Any product that may be evaluated in this article or claim that may be made by its manufacturer is not guaranteed or endorsed by the publisher.
Research integrity at Frontiers
Learn more about the work of our research integrity team to safeguard the quality of each article we publish.