- 1College of Mechanical and Electrical Engineering, Northeast Forestry University, Harbin, China
- 2Power Dispatching and Control Center, State Grid Heilongjiang Co., Ltd., Harbin, China
- 3Harbin Power Supply Company, State Grid Heilongjiang Co., Ltd., Harbin, China
- 4Suzhou Power Supply Company, State Grid Jiangsu Co., Ltd., Suzhou, China
- 5Changsha University of Science and Technology, Changsha, China
Enhancing the flexibility of power systems helps to balance the increasing renewable generation for achieving the rigid carbon reduction targets. The current flexible system in China can hardly meet the regulating demand of its ever-increasing renewable generation, especially in the Heilongjiang power grid (HPG), where the proportion of combined heat and power units that determine power generation by the heat requirement is enormous, while the peak shaving capacity in winter is limited. This research analyzes the necessity of establishing a new flexibility system for the HPG. Also, it introduces the new characteristics and the flexibility of the HPG. Then, it proposes classification and definition methods for the flexibility system of the HPG by referring to some similar power grids, e.g., the UK grid and the Danish grid. A simulation framework of power systems with flexibility enhancement is developed to perform quantitative evaluation of the HPG in different carbon reduction scenarios. The suggested flexibility system reorganizes and revises the principles of reserve classification, response time, and minimum reserve configuration. Simulation results verify the effectiveness of the proposed method through the measurement and analysis data of the HPG. This paper takes the HPG as an example to perform a quantitative analysis of the flexibility system. Also, it can provide tips and suggestions for other regions seeking carbon reductions.
1 Introduction
China sets the ambitious goals of achieving carbon emissions peak by 2030 and carbon neutrality by 2060 (Chen et al., 2018a), and constructing new types of power system is indispensable to achieving these great goals. Therefore, the rapid development of renewable energy, the bold electricity market reform, and the increasing proportion of regional power grids will significantly change the characteristics of the power system (Li et al., 2017; Guo and Zhao, 2018; Zhou et al., 2019; Guo et al., 2020). To cope with the various uncertainties occurring in the increasingly complex operating conditions, these issues desperately need a larger and more diverse active regulation capability (Ban et al., 2021). Therefore, the power sector performs a significant paradigm shift in the flexibility system to develop more measures to cope with the uncertainties like prediction deviations, power shortages, and operation accidents (Kondziella and Bruckner, 2016; Gandoman et al., 2018; Chen et al., 2020). For example, the Guide on Technology for the Power System. GB/T 38969-2020 (Li et al., 2021) reorganized and explained the contents related to various operating reserve in China. Likewise, some typical grid systems like the UK grid and the Danish grid made provisions in terms of the type of reserve (Child et al., 2019), response time, and minimum reserve configuration capacity to meet the active regulation needs of the power grid based on the operating characteristics of their respective systems. Besides, Tushar et al. (2014) reviews the development of power grids in countries and regions such as North America, Australia, and Europe, and they classified their reserve criteria in a more refined way. In general, the power systems are undergoing the transition from a simple reserve system to a diversified one (Li et al., 2018; Chen et al., 2018b; Munné-Collado et al., 2019). Therefore, quantifying the power systems’ flexibility requirements and capability is essential, taking into account decarbonization options for the power sector.
Flexibility refers to the extent to which a power system can modify electricity production or consumption in response to variability, expected or otherwise (Feng et al., 2022). Or, in order to provide a service within the energy system, it can be described as the modification of generation injection or consumption patterns in reaction to an external signal (price signal or activation) (Heydarian-Forushani and Golshan, 2020). At present, the proportion of flexible sources such as hydropower, pumped storage, and gas-fired power plants with adjustable performance in areas rich in renewable energy resources in China is low, and the system flexibility is insufficient (Kehler and Hu, 2011; Aghaei and Alizadeh, 2013; Song et al., 2021). Flexibility does not make coal-fired units clean, but making existing ones more flexible enables the integration of more renewable generation in the power system. Affected by factors such as unit performance design and electricity price mechanism, the maximum peak shaving range of coal-fired units in China is generally set at 50%. The minimum load and ramp rate indicators of conventional units are far behind the levels of leading countries, such as that in the UK grid and the Danish grid. In the Heilongjiang power grid (HPG), a typical representative energy system in the Northeastern China, the proportion of heating units is high, and the peak shaving capacity in winter is extremely limited (Richter et al., 2019). Besides, most of HPG’s cogeneration units are not gas-fired units and thus demonstrate limited flexibility. Next, the shortage of flexibility will not only be aggravated by the increasing differences between load peak and off-peak but also by the expansion of the heating area in the corresponding season. Therefore, the flexibility shortage severely restricts the development of renewable energy.
In summary, the current flexibility system of the HPG can hardly meet the increasing flexibility demand. Also, the power marketization process puts forward new requirements on the types and sources of flexibility. Therefore, it is necessary to study the flexibility system of the HPG with new operating characteristics. It will help to improve the safety and reliability of the HPG. Also, it will lay the foundation for the power market reform in the HPG. Considering the above issues, reviewing and revising the operating reserve system of the HPG is necessary to cope with the increasing operating complexity and to improve its flexibility. First, this paper introduces the data of HPG and analyzes the necessity of constructing a flexibility system for the HPG considering rigid carbon reduction targets. Then, the foreign typical power grid operating reserve systems are introduced and combine the existing domestic guidelines and regulations to construct a flexibility system for the HPG under the new situation. Also, it reorganizes the classification of the reserve, response time, and minimum reserve configuration principles and gives suggestions for revision. At last, the rationality of the proposed flexibility system is verified through the analysis of the actual operating data of the HPG.
2 Introduction of the Heilongjiang Power Grid and its Flexibility
This section illustrates the necessity of enhancing the flexibility system for the HPG, and it explores the new characteristics of the HPG.
2.1 Introduction of the Heilongjiang Power Grid
Heilongjiang is located in Northeast China, with a 32 million population and a power supply area of about 4.7 × 105 km2. The overall installed generation capacity in Heilongjiang Province is redundant, and coal power units are mainly for heat supply with low utilization efficiency and severe surplus. At of the end of 2019, the installed capacity of coal power units in Heilongjiang Province accounted for 71.25% of the total installed power generation capacity and over 90% of the installed capacity of thermal power units. At present, Heilongjiang Province is rich in wind resources, with a potential development capacity of about 23,000,000 kW and an installed capacity of about 54,000,000 kW. By the end of 2019, wind power accounted for 19.07% of the total installed power supply in Heilongjiang Province, as shown in Figure 1. Heilongjiang Province is rich in solar energy resources, with an average of 2,400–2,800 h of sunshine per year. Also, the installed photovoltaic (PV) capacity in Heilongjiang Province developed rapidly and increased to 201 million kW by 2019. Heilongjiang Province demonstrates a high degree of hydroelectric development, with an installed hydroelectric capacity of 1,089,700 kW by the end of 2019, accounting for 3.4% of all installed power sources. By the end of 2020, 1,500,000 kW of biomass power plants and wind power accounts for 28.6% of the total installed capacity: see Figure 1.
The renewable power consumption of the HPG is limited by uncoordinated power planning, insufficient peak regulation capacity of the market incentive system, and other factors. Take wind power as an example, whose consumption capacity of the province is limited, and it fails to reach the fully guaranteed acquisition hours of renewable energy. The HPG bears a high wind curtailment rate exceeding 20%, and the wind curtailment rate increased from 15% in 2013 to 21% in 2015. Since 2016, the HPG implemented a series of measures to increase the capacity of renewable energy consumption, including constructing new power transmission lines and thermal power flexibility transformation pilots. Also, accordingly, the wind power utilization hours get close to the national average level, and the wind abandonment rate dropped to 1% in 2019, which improved the wind curtailment situation, as shown in Figure 2.
2.2 Flexibility of the Heilongjiang Power Grid
The HPG installed 9 GW wind power and 6 GW PV by the end of 2020. However, due to the high proportion of combined heat and power units, the minimum output of the HPG system is difficult to be compressed during the heating periods. The minimum output regulation of coal-fired units in the HPG is limited, and the minimum output can only be reduced to 50%. To ensure the heat supply, a very limited regulation capacity is left for wind power generation, even during high wind generation periods (Fang et al., 2016). In addition, the startup and shutdown time of coal units is also an important factor affecting flexibility. The startup and shutdown time of coal-fired units in the HPG is usually between 3 and 5 h. The long startup and shutdown time make the system unable to meet the demand for downward regulation when renewable energy is connected to the grid in a timely manner (Jiang et al., 2019). In general, the coal-fired units in Heilongjiang Province are redundant. They demonstrate limited regulation capability, and no gas-fired units exist with strong regulation capability, which makes the HPG lack the flexibility to consume high penetration of renewable energy.
To address the above issues, the flexibility of the HPG can be enhanced in the following ways.
From the grid side, the HPG needs to improve the transmission capacity of the interprovincial lines. During the 13th Five-Year Plan period, the HPG exhibited a surplus of electricity. The power transmission to other provinces was significant, which improved the wind power consumption capacity to a certain extent. In 2019, the northeast regional grid sent 11,264 GWh to the HPG, while the latter sent 20,854 GWh to the former. The cross-regional power exchange improves the wind power consumption capacity of the HPG. In the future, it is necessary to continue enhancing interprovincial transmission capacity, improving the utilization rate of transmission grids, and improving the flexibility system.
From the customer side, the degree of future customer-side flexibility in the HPG can be determined by multiple factors. The most important ones include the maturity of the electricity market, the ability to support smart electricity meters and innovative grid technologies, the scale of energy storage, and the number of deployable electric vehicles. From both the generation and consumption sides, the existing dispatching plan in the HPG is still managed in a planned economy. Various flexible resources are not motivated to play an active role in system balancing and regulation.
In general, the existing flexibility system of the HPG can hardly meet develop demands of renewable energy. The current dispatching mode mainly aims to guarantee the utilization hours of generating units and complete the annual power generation plan. Next, it makes the generating units unwilling to provide other services such as peak regulation. For example, units with higher energy efficiency and regulation capacity demonstrate little additional profits in the dispatching process, making them less motivated to improve their flexibility further. Therefore, when the balancing of power supply and load is tight, dispatching agencies are required to use mandatory measures like peak avoidance, power restriction, and lockout to keep a strenuous balance (Belderbos and Delarue, 2015).
2.3 New Characteristics of the Heilongjiang Power Grid
The operation characteristics of the HPG changed significantly due to issues like the rapid development of renewable energy, the increasing cross-regional power transmission, and the development of the electricity market. The resulted new situations and new challenges can be concluded as follows.
(1) The rapid development of distributed renewable energy in the HPG exacerbates active regulation capacity shortages. Meanwhile, the load volatility in the HPG is increasing. Therefore, the flexibility of the HPG needs to be further enhanced to maintain system frequency stability and power balance.
(2) The proportion of dispatching caliber of new energy will increase, and some of the peak hours of electricity consumption will face the situation of insufficient flexibility. Uncertainty of new energy output will directly affect the balance of supply and demand of electricity. During the winter and summer peak consumption periods, a shortage in the whole grid will occur during local periods and insufficient flexibility of some regional power grids.
(3) The rapid advancement of the electricity marketization process increased flexibility management compliance and refinement requirements. The HPG carried out a simulation operation. The other provinces and cities will also construct the electricity spot market one after another. Under the power market environment, the retention of flexibility will be more focused on economic benefits. This process will further enhance the compliance and refinement requirements for flexibility management.
In general, considering the new characteristics of the HPG, the flexibility capacity of the HPG will be challenging to meet the flexibility demand, and the power marketization process also puts forward new requirements on the type and source of flexibility. Therefore, it is necessary to investigate and sort out the operating reserve system of the existing typical power grid and provide suggestions for the construction of the operating reserve system of the HPG under the new operating characteristics, to further improve the regulation capacity of the HPG under the new power system development, guarantee the operational safety, and power supply reliability of the HPG.
3 Construction of Flexibility System of the Heilongjiang Power Grid
The UK grid and Danish grid are well in construction, and their generating units also need to meet heating requirement. This section investigates the operation of typical foreign power grids, i.e., the UK grid and the Danish grid. It analyzes the characteristics of the operating reserve system in different operating conditions to provide references for the construction of the flexibility system for the HPG.
3.1 Flexibility System of Typical Power Grids
3.1.1 UK Grid
The operating reserve of the UK grid mainly includes the accident reserve, fast reserve, and short-term operating reserve in its reserve system, and the purpose and requirements of the corresponding reserve settings are as accident reserve, fast reserve, and short-term operating reserve. Accident reserve deals with uncertainties in the availability of large power stations and errors in weather and load forecasts (Wilson et al., 2011). In real-time system operation, fast reserve copes with load forecast errors and new energy generation forecast errors. The UK grid requires a fast reserve unit to respond within 2 min after dispatching instructions are issued, reach full output within 4 min, and last for 15 min. The minimum capacity of the unit to provide fast reserve is 2.5 × 103 kW. Short-term operating reserve copes with load forecast errors and new energy generation forecast errors within the system, as well as to guarantee frequency stability at low frequencies of the system. The UK grid requires short-term operating reserve units to respond within 20 min of dispatching instructions and maintain full output for 2 h and up to 4 h. The minimum capacity of short-term operating reserve units available is 3 × 103 kW.
Minimum reserve configuration principle of the UK grid considers the uncertainty of operation accidents of the system and the prediction error of load and new energy output. The day-ahead operating reserve capacity
where
3.1.2 Danish Grid
The operating reserve of the Danish grid mainly includes primary reserve and secondary reserve (Li et al., 2021). Operating reserve copes with uncertain events such as load forecast errors and forced outages. In this setting, the operating reserve capacity refers to the reserve capacity of all units that can respond to the dispatching instruction and the load capacity that can be removed within 30 min. Besides, the units involved in the operating reserve include grid-connected units and offline units. Primary reserve copes with load forecasting errors, which need to be kept synchronously with the grid and can be put into operation within 10 min (Zheng et al., 2013). The primary reserve includes synchronous reserve and nonsynchronous reserve. Among them, synchronous reserve (i.e., spinning reserve) refers to the increase in output or reduction in a load of grid-connected units within 10 min. Non-synchronous reserve (i.e., quick-start reserve) refers to the generation capacity of offline units that are immediately started and connected to the grid within 10 min according to the dispatching instruction. Next, secondary reserve copes with uncertain events such as load forecast errors and forced outages on the generation side. The secondary reserve capacity refers to the unit reserve capacity that can respond to the dispatching command within 10–30 min and the load that can be removed. From the above classification and definition, it can be seen that the Danish grid classifies reserves mainly based on response time and takes into account both generation-side and load-side resources.
The day-ahead operating reserve capacity
where
The overview of the flexibility system of the UK grid and the Danish grid states that setting operating reserve needs to consider the effects of power shortages caused by load forecast errors, renewable energy fluctuations, and operation accidents. Besides, to maintain the system’s frequency stability and power balance, different time scales and different types of reserve services should be set to respond to the active power shortages. The operation characteristics of the UK grid, the Danish grid, and the HPG are comparable and analogous; therefore, replicating the structure of the foreign flexibility system is possible to meet the actual grid operation needs of the HPG. Still, it is necessary to study further and establish a flexibility system applying to the practical network conditions of the HPG.
3.2 Classification and Definition for Flexibility System of the Heilongjiang Power Grid
This section reorganizes and classifies the flexibility from reserve and response time perspectives. It draws on the successful experience of the typical flexibility system of foreign power grids, e.g., the UK grid and the Danish grid, and it considers the challenges faced by the HPG in terms of flexibility system enhancement and new issues newly released by Code on Security and Stability for Power System. GB 38755—2019 and Guide on Technology for Power System. GB/T 38969-2020. The operating reserve is divided into positive reserve and negative reserve. Also, the positive reserve is further classified into a 10-min reserve (including spinning reserve) and 30-min reserve, and the purpose and source of the corresponding reserve are introduced as follows.
(1) Operating reserve. The reserved capacity to balance the power imbalance of the grid caused by fluctuations in load and sources, grid accidents, and other uncertain future events to ensure safe operation of the grid and reliable power supply. The operating reserve needs to consider the impact of the distributed renewable energy with strong stochastic characteristics, and it involves both positive regulation capability and negative regulation capability.
(2) Spinning reserve. In order to cope with the load and power fluctuation, single large unit tripping, and multiple large units lost due to unplanned failure, etc., reserved upregulation capacity is immediately available for load. It is not subject to grid stability limits. The spinning reserve is included in the 10-min reserve. The total spinning reserve should neither be less than the maximum capacity of the largest unit nor be less than 2.5% of the highest forecast load of the power system.
(3) 10-min reserve. In order to guarantee the system reserve adequacy after the unit tripping, DC lockout, grid accident, and other events in the case of load and power (including new energy) fluctuation, the reserve capacity arranged can be fully transferred within 10 min. Also, it is not bound by the grid stability limit and can last at least 1 h. The total 10-min reserve of the whole grid is taken as the sum of the larger of the largest single capacity, the largest DC bipolar transmission power of the grid in operation at that time, and 2.5% of the highest forecast load of the whole grid.
(4) 30-min reserve. Reserved capacity that can be fully deployed by the power dispatching department within 30 min, is not bound by the grid stability limit, and can last at least 2 h. The 30-min reserve of each province and municipality is not less than the loss caused by the failure of a single facility. Also, it is not less than the maximum loss of regional power grids after the bipolar blocking.
(5) Negative reserve. In response to load and power supply fluctuations, sudden loss of transmission lines, and other unexpected situations, the negative reserve can be fully transferred out within 10 min. Also, it is not limited by the grid stability constraints. The negative reserve capacity is 3% of the lowest forecast load in the normal conditions. In extreme natural weather conditions like holidays and typhoons, the negative reserve adjusts according to practical conditions.
3.3 Simulation Framework for Power System With Flexibility Enhancement
The framework of the power system operation simulation with flexibility enhancement is shown in Figure 3.
The power system operation simulation framework taking flexibility enhancement into account mainly consists of three major parts: input data, calculation links, and simulation results. The input data part forms the power system operation boundary conditions based on the practical data of the HPG, combined with the system load and dispatching operation mode of power system. Next, the maintenance schedule module arranges the maintenance scheduling considering the coordination between different regions and different types of power sources to ensure the steady operation of the system.
Based on the stochastic characteristics of renewable energy generation (like wind power or PV) and the installed capacity of renewable energy resources, the simulation module simulates the stochastic operation of renewable energy resources. Next, it generates a series of power generation time series consistent with the stochasticity, volatility, and spatial-temporal correlation of renewable energy power generation (Liu et al., 2016). Based on the input boundary conditions, unit maintenance plan, stochastic operation mode of renewable energy, and flexibility transform of thermal power units, the day-by-day operation simulation module considers the operation characteristics of thermal power and renewable energy. It simulates the dispatching operation of the system on a day-by-day basis (Wang et al., 2008; Ban et al., 2017). Based on the unit output information obtained from the daily operation simulation, the simulation results are calculated with the evaluation indexes such as unit power output, system operation cost, start and stop cost, etc.
4 Quantitative Evaluation of the Flexibility in the Heilongjiang Power Grid
In the HPG, the total electricity consumption in 2019 is 89,320,000 MWh, and the maximum hourly electricity consumption is 11,584 MW. According to the actual power supply installation and the heat supply constraint in 2019, 22,841 MW of coal power installation, 6,114 MW of wind power installation, and 2,012.85 MW of photovoltaic installation are retained. According to the National Energy Administration, Heilongjiang exhibits 2,323 h of a full generation of wind power and 1,459 h of a full generation of PV for the year. The specific parameters of 40% renewable energy penetration in Heilongjiang Province are designed as follows. The annual load and the installed capacity of coal power remain unchanged, and the installed capacity of wind power and PV in the power system increases to 13,145.1 and 3,558.6 MW, respectively. Six operation scenarios of the HPG are employed, which are shown in Table 1, to implement the quantitative evaluation of the power system flexibility. Also, the renewable energy penetration is calculated by referring to the ratio of total renewable energy generation to total electricity consumption.
(1) Baseline scenario. Baseline scenario (renewable energy penetration is 19%).
(2) Baseline+ scenario. Thermal units under the baseline scenario with deep peaking flexibility modification: assume that the minimum output of 5,150 MW coal-fired units can be achieved after flexibility modification is 30%.
(3) Baseline++ scenario. Equal coal power flexibility capacity replacement to gas power under the baseline scenario: 5,150 MW of coal-fired units can be improved by flexibility enhancement with 1,030 MW of regulation capacity. This scenario directly replaces its equal capacity with 1,030 MW of gas power.
(4) Carbon reduction scenario. 40% renewable energy penetration with coal-fired unit flexibility enhancement, i.e., 3,020 MW of coal-fired units will demonstrate more flexibility by deep peak shaving transformation.
(5) Carbon reduction+ scenario. 40% renewable energy penetration with 5,150 MW coal-fired unit flexibility enhancement, namely, carbon reduction scenario, and an additional 1,030 MW of gas-fired units.
(6) Carbon reduction++ scenario. 40% renewable energy penetration with 5,150 MW coal-fired unit flexibility enhancement and 1,030 MW of gas-fired units, namely, carbon reduction+ scenario, and additional flexibility of energy storage and demand response (DR). The penetration of DR can reach 5% of the peak load and 1,500 MW/9,000 MWh energy storage is installed to help balancing the peak and valley loads.

TABLE 1. Scenario settings for evaluation of power system flexibility in the Heilongjiang power grid .
Calculations of the renewable energy penetration is according to the ratio of total renewable energy generation to total electricity requirement, as shown in Eq. 3
where
The simulation results are listed in Tables 2 and 3. In Table 2, Ewind and Cwind represent generation and curtailment of wind power, EPV and CPV represent generation and curtailment of PV, and φwind and φPV represent wind power penetration and PV penetration. In Table 3, ΔTCFU and ΔCCFU represent changes in annual startup/shutdown times and cost of coal-fired units, ΔTGFU and ΔCGFU represent changes in annual startup/shutdown times and cost of gas-fired units, ΔC0 CFU represents idle cost of coal-fired units, and ΔCFE CFU represents estimated flexibility enhancement cost range of retrofitting coal-fired units, while ΔCNEW GFU represents estimated invest cost range for installing gas-fired units.
In the baseline+ scenario, coal-fired units with deep peak shaving transformation, namely, the minimum output is reduced to 30% of the total generation capacity, can accommodate more renewable generation, i.e., wind power. Also, the wind power generation increases by 81 GWh compared with the baseline scenario, and the wind power curtailment decreases from 473 to 392 GWh. By comparison, the wind power generation in the baseline++ scenario increases by 292 GWh compared with that of the baseline scenario, and the wind power curtailment decreases from 473 GWh in the baseline scenario to 181 GWh. In addition, gas-fired units can significantly reduce the startup/shutdown frequency of coal-fired units. Also, the number of startup/shutdown operations of coal power units in the baseline++ scenario reduces by 83.56% compared with that in the baseline scenario. Therefore, the startup/shutdown cost reduces by 86.52%, as shown in Table 3. Next, it shows that gas-fired units demonstrate significantly better performance than that of coal-fired units deep peak shaving transformation concerning flexibility enhancement. The startup/shutdown times and cost of coal-fired units are higher than those of gas-fired units. However, it also should be noted that the investment cost of gas-fired units, i.e., ¥0.3052 billion to ¥0.3329 billion, is much higher than that of retrofitting coal-fired units, i.e., ¥0.0357 billion to ¥0.0512 billion.
In scenarios with 40% penetration of renewable energy, the addition of gas-fired units on top of the flexibility enhancement of coal-fired units can further reduce the wind power curtailment from 8,088 to 7,532 GWh: see Table 2. Since gas-fired units demonstrate lower startup/shutdown costs, higher ramp capacity, and faster response-ability, the startup/shutdown frequency of gas-fired units increases by 825.29% compared with the baseline scenarios. Therefore, the startup/shutdown cost of gas-fired units increases by 850.00%. However, coal-fired units’ startup/shutdown frequency decreases by 12.63%, making the system still demonstrating better performance. Furthermore, the additional flexibility of energy storage and DR in the carbon reduction++ scenario increases the wind power generation, while reducing the wind power curtailment. The system performance is obviously better than that in the carbon reduction+ scenario that only exhibits flexibility enhancement of coal power units. Next, wind power generation increases by 2,673 GWh. The proportion of wind power generation to all power generation increases from 32.99% to 41.48%: see Table 3. The proportion of PV generation increase from 4.79% to 8.45%, and no PV generation curtailment occurs. The number of startup/shutdown operations and corresponding costs of coal-fired units decrease by 27.22% and 25.04%, respectively, while the number of startup/shutdown operations of gas-fired units increases by only 9.36%: see Table 3.
Typical scheduling results in three scenarios are given in Figures 4–6. In the baseline scenario, coal-fired units dominate the output profiles: see Figure 4. When the renewable energy penetration reaches 40%, the first peak load time shifts from 7:00 to 10:00: see Figure 5. The system needs coal-fired units to start quickly to increase the upward regulation capacity, but the coal power units exhibit a long startup time and can hardly respond to the system load in time. Besides, as the number of startups and shutdowns increases, it will cause more startup and shutdown costs, which will also affect the life of units. Also, the deep peak regulation flexibility enhancement of coal-fired units can no longer meet the demand of downward regulation, and the wind power generation curtailment will increase. DR can play a significant role of peak shaving during peak periods, as shown in Figure 6. After adding DR, the system load is equivalent to a part of the reduction during the peak hours. That is, the orange line show that DR during peak hours directly reduces peak load and accordingly reduces the need for upward flexibility. In general, the coal-fired units only generate at the minimum output, a portion of the units are in the state of shutdown, and gas-fired units can start quickly and respond to system peak demand, while energy storage can guarantee peak-load demand. The combination of coal-fired units flexibility, gas-fired units flexibility, energy storage, and DR provides more regulation capability for the power system.
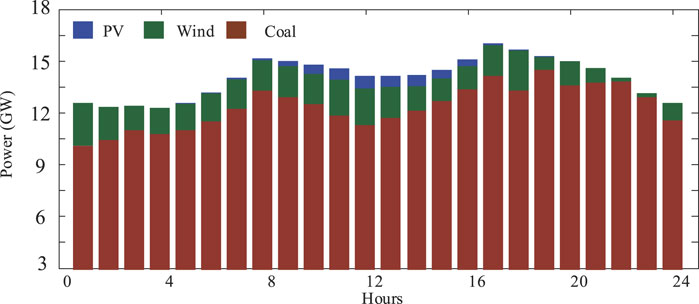
FIGURE 4. Power components of the Heilongjiang power grid on a typical winter day under in baseline scenario.
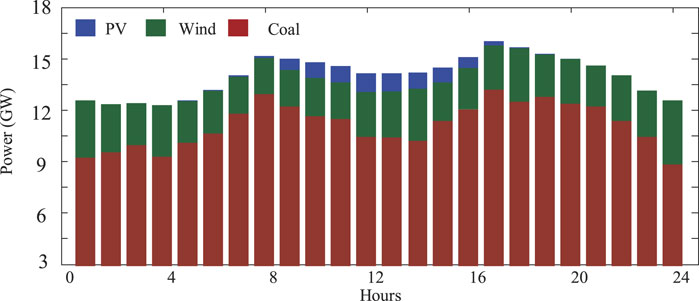
FIGURE 5. Power components of the Heilongjiang power grid on a typical winter day in carbon reduction + scenario.
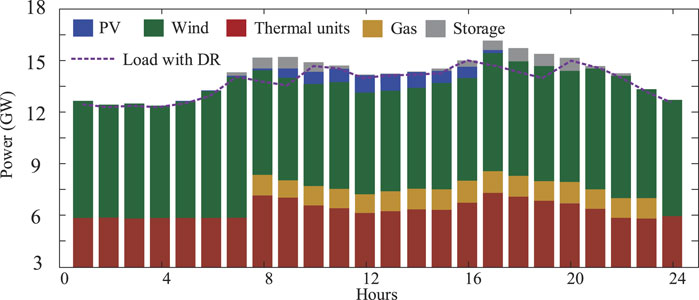
FIGURE 6. Power components of the Heilongjiang power grid on a typical winter day in carbon reduction ++ scenario.
In general, the flexibility of the power system is enhanced by the modified coal-fired units, in which the system features improved downward regulation capability, reduced costs in startup and shutdown, and exhibit better cost-effectiveness in comparison to the gas-fired units. However, with the further development of renewable energy in the future, flexibility enhancement of coal-fired units, even with additional gas-fired units, can hardly meet the demand of renewable energy consumption, which requires various flexible resources. Gas power, energy storage, DR, and grid mutual aid can rapidly change power output at peak and low load periods and coordinate with wind and PV power output characteristics, allowing the system to maintain operational safety and reliability in a more economical way (Orfanos et al., 2013; Palmintier and Webster, 2016; Takeshita et al., 2021; Li et al., 2022).
5 Discussions and Suggestions
5.1 Discussions
(1) Thermal power flexibility transformation can hardly support medium and long-term power system flexibility demand by itself, in terms of comprehensive regulation capability and technical-economic performance. Although thermal power flexibility transformation will significantly improve the upward and downward flexibility with acceptable cost, fully meeting all the flexibility demands for a future power system with extremely high proportion of renewable energy is still impossible. Another thing worth noting is that gas-fired units demonstrate a high-power supply efficiency, short startup and shutdown time, and fast climbing rate than coal-fired units, and they are the best peaking power source in terms of regulation characteristics. Also, gas-fired units will provide strong support for power system flexibility with the decrease of construction cost and gas price. Therefore, gas-fired units demonstrate potentials to play an import role in future flexibility system.
(2) The development of different types of flexible resources varies and the potential of source-grid-load-storage flexibility needs to be further released. On the resource side, the completed coal units can reduce the minimum stable output to 20–30% of their rated capacity, through technical modifications such as thermal-electrolytic coupling and low-pressure combustion stabilization, but their climbing rate is slow. Next, coal power flexibility transformation has been developed on a pilot basis, but the scale of the transformation is still insufficient, and the flexibility potential has not been fully released. On the grid side, grid interconnection and mutual aid can take advantage of the nonsimultaneous nature of electricity consumption in each region for load adjustment, reduce spare capacity, improve power quality, enhance the system’s ability to withstand accidents more economically, consume more scenic volatile power sources, and improve grid security and power supply reliability.
(3) The current power market mechanism limits the system flexibility enhancement for the future power system with high penetration of renewable energy. In the HPG, for example, the existing coal unit deep peaking flexibility transformation can reduce coal unit startup and shutdown and enhance its downward adjustment capability. Administrative means can compensate for its deep peaking cost in the auxiliary service market. However, with high renewable energy penetration in the future, the demand for flexibility cannot be met by deep peak regulation of coal power alone. Next, the existing market mechanism for ancillary services cannot actively stimulate other units to actively participate in system flexibility regulation. It is necessary to stimulate various flexible resources such as gas and electricity, energy storage, and DR to adjust their output according to load changes through the electricity market price mechanism and also introduce flexibility regulation products in the auxiliary service market, so that various resources can obtain certain economic benefits. Using market competition, they can make up for the lack of flexibility in real-time dispatching and, at the same time, obtain certain economic benefits. Therefore, improving the electricity spot market and ancillary services market is essential to enhance the flexibility of the power system.
5.2 Suggestions
(1) Establishing a reasonable path for medium and long-term flexibility enhancement. The current thermal power system is not flexible enough, while the installed capacity of renewable energy is increasing year by year, showing a serious mismatch between the two. Retrofitting thermal power can significantly improve system flexibility at this stage. However, as the flexibility demand rises rapidly, it is not advisable to install more coal-fired units to support a high penetration of renewable energy. In the long run, developing other flexible resources such as gas-fired units, energy storage hydropower, and DR is reasonable to promote the renewable energy.
(2) Performing medium- and long-term power system planning considering the coordination and unification of source-grid-load-storage flexibility resources development. It is necessary to accurately assess the future flexibility demand, incorporate the flexibility enhancement targets into medium- and long-term power planning, and promote the coordinated development of source-grid-load-storage flexibility resources. Besides, it is necessary to invest in power supply construction reasonably according to flexibility needs and guide power plants to adopt more flexible operation modes. The DR participation fields should be expanded, and DR incentive rates and penalties should be improved to accelerate implementation. Besides, energy storage, including electrochemical, water, gas, and thermal storage, should be emphasized and accelerated as flexible resources at the planning stage.
(3) Taking measures to explore the flexibility potential of source-grid-load-storage. It is necessary to explore the flexibility potential of various existing resources from the power supply side, the grid side, and the user side. On the power supply side, the flexibility potential of the thermal power units needs to be fully utilized to enhance the system’s flexibility by changing the operation mode of the existing units, making equipment flexibility modifications, and innovating flexible power generation methods. Next, on the grid side, the utilization of existing transmission lines needs to be improved. In particular, the extrahigh-voltage network needs to be brought into play to restructure the grid pattern and reduce the additional flexibility demand due to grid blockage. On the user side, the tariff can be used to achieve better user-side flexibility regulation. For energy storage, it is necessary to optimize the incremental amount and pay attention to its coordinated development and application on the power, grid, and user sides.
(4) Adapting power markets to fully tap the flexibility potential of thermal power plants and developing more flexibility regulation products. Real-time markets with fast response times need to be created to shorten transaction time scales to the minute level, enabling the market to make timely and rapid price judgments with volatile renewable energy generation. More complete compensation mechanisms, considering characteristics such as fast climbing capability, minimum upward and downward climbing time, response accuracy, and reasonable pricing of flexible resources, are required to reduce the incentive for inflexible operating power plants and realize the superiority of flexibility resources. Besides, the power system can adopt load demand-side management, spare capacity sharing, and interprovincial mutual assistance to peak-load shifting and further guide demand-side electricity consumption through price mechanisms such as time-sharing tariffs and peak tariffs.
6 Conclusion
More variable power production increases the flexibility requirements placed on the overall power system, both on the supply and demand sides. This paper studies the flexibility enhancement methods for a regional power system, i.e., the HPG, considering issues like operation characteristics, renewable energy development, load fluctuation, and the electricity market reform. Next, by referring to similar power grids, it reorganizes the definition and classification principles of reserve capacity, response time, and minimum reserve. Besides, it performs a quantitative evaluation of the flexibility system in the HPG by developing a simulation framework, and then, it makes some practical discussions and suggestions to improve the flexibility of the HPG. The effectiveness of the proposed methods is demonstrated through the historical and forecasted data of the HPG, and it will also provide references for developing flexibility systems for other regional power grids.
Data Availability Statement
The original contributions presented in the study are included in the article/supplementary material, and further inquiries can be directed to the corresponding authors.
Author Contributions
Conceptualization, MB and QL; methodology, HX; software, QL; validation, QL and MB; formal analysis, MB; investigation, XS; resources, JG; data curation, QL; writing—original draft preparation, QL; writing—review and editing, MB; visualization, MB and QL; supervision, HJ; project administration, JG; All authors have read and agreed to the published version of the manuscript.
Conflict of Interest
Authors HJ and JG were employed by the company Power Dispatching and Control Center, State Grid Heilongjiang Co., Ltd. Author YuL was employed by the company Harbin Power Supply Company, State Grid Heilongjiang Co., Ltd. Author HX was employed by the company Suzhou Power Supply Company, State Grid Jiangsu Co., Ltd.
The remaining authors declare that the research was conducted in the absence of any commercial or financial relationships that could be construed as a potential conflict of interest.
Publisher’s Note
All claims expressed in this article are solely those of the authors and do not necessarily represent those of their affiliated organizations, or those of the publisher, the editors, and the reviewers. Any product that may be evaluated in this article, or claim that may be made by its manufacturer, is not guaranteed or endorsed by the publisher.
References
Aghaei, J., and Alizadeh, M.-I. (2013). Demand Response in Smart Electricity Grids Equipped with Renewable Energy Sources: A Review. Renew. Sustain. Energy Rev. 18, 64–72. doi:10.1016/j.rser.2012.09.019
Ban, M., Bai, W., and Zhu, L. (2021). Optimal Scheduling for Integrated Energy-Mobility Systems Based on A Renewable-To-Hydrogen Station and Tank Truck Fleets. IEEE Trans. Industry Appl. 58 (2), 2666–2676. doi:10.1109/TIA.2021.3116117
Ban, M., Yu, J., Shahidehpour, M., and Yao, Y. (2017). Integration of Power-To-Hydrogen in Day-Ahead Security-Constrained Unit Commitment with High Wind Penetration. J. Mod. Power Syst. Clean. Energy 5 (3), 337–349. doi:10.1007/s40565-017-0277-0
Belderbos, A., and Delarue, E. (2015). Accounting for Flexibility in Power System Planning with Renewables. Int. J. Electr. Power & Energy Syst. 71, 33–41. doi:10.1016/j.ijepes.2015.02.033
Chen, X., Lv, J., McElroy, M. B., Han, X., Nielsen, C. P., and Wen, J. (2018b). Power System Capacity Expansion under Higher Penetration of Renewables Considering Flexibility Constraints and Low Carbon Policies. IEEE Trans. Power Syst. 33 (6), 6240–6253. doi:10.1109/TPWRS.2018.2827003
Chen, X., McElroy, M. B., and Kang, C. (2018a). Integrated Energy Systems for Higher Wind Penetration in China: Formulation, Implementation and Impacts. IEEE Trans. Power Syst. 33 (2), 1309–1319. doi:10.1109/TPWRS.2017.2736943
Chen, Z., Hu, Y., Tai, N., Tang, X., and You, G. (2020). Transmission Grid Expansion Planning of a High Proportion Renewable Energy Power System Based on Flexibility and Economy. Electronics 9 (6), 966. doi:10.3390/electronics9060966
Child, M., Kemfert, C., Bogdanov, D., and Breyer, C. (2019). Flexible Electricity Generation, Grid Exchange and Storage for the Transition to a 100% Renewable Energy System in Europe. Renew. Energy 139, 80–101. doi:10.1016/j.renene.2019.02.077
Fang, X., Hu, Q., Li, F., Wang, B., and Li, Y. (2016). Coupon-Based Demand Response Considering Wind Power Uncertainty: A Strategic Bidding Model for Load Serving Entities. IEEE Trans. Power Syst. 31 (2), 1025–1037. doi:10.1109/TPWRS.2015.2431271
Feng, C., Liang, B., Li, Z., Liu, W., and Wen, F. (2022). Peer-to-Peer Energy Trading under Network Constraints Based on Generalized Fast Dual Ascent. IEEE Trans. Smart Grid 3053, 1. doi:10.1109/TSG.2022.3162876
Gandoman, F. H., Ahmadi, A., Sharaf, A. M., Siano, P., Pou, J., Hredzak, B., et al. (2018). Review of FACTS Technologies and Applications for Power Quality in Smart Grids with Renewable Energy Systems. Renew. Sustain. Energy Rev. 82, 502–514. doi:10.1016/j.rser.2017.09.062
Guo, H., Davidson, M. R., Chen, Q., Zhang, D., Jiang, N., Xia, Q., et al. (2020). Power Market Reform in China: Motivations, Progress, and Recommendations. Energy Policy 145, 111717. doi:10.1016/j.enpol.2020.111717
Guo, Y., and Zhao, C. (2018). Islanding-aware Robust Energy Management for Microgrids. IEEE Trans. Smart Grid 9 (2), 1301–1309. doi:10.1109/TSG.2016.2585092
Heydarian-Forushani, E., and Golshan, M. E. H. (2020). Quantitative Flexibility Assessment of a Comprehensive Set of Demand Response Programs. Int. J. Electr. Power Energy Syst. 116, 105562. doi:10.1016/j.ijepes.2019.105562
Jiang, W., Yang, K., Yang, J., Mao, R., Xue, N., and Zhuo, Z. (2019). A Multiagent-Based Hierarchical Energy Management Strategy for Maximization of Renewable Energy Consumption in Interconnected Multi-Microgrids. IEEE Access 7, 169931–169945. doi:10.1109/ACCESS.2019.2955552
Kehler, J. H., and Hu, M. (2011). Planning and Operational Considerations for Power System Flexibility. IEEE Power Energy Soc. General Meet. 2011, 1–3. doi:10.1109/PES.2011.6039859
Kondziella, H., and Bruckner, T. (2016). Flexibility Requirements of Renewable Energy Based Electricity Systems - A Review of Research Results and Methodologies. Renew. Sustain. Energy Rev. 53, 10–22. doi:10.1016/j.rser.2015.07.199
Li, J., Liu, F., Li, Z., Shao, C., and Liu, X. (2018). Grid-side Flexibility of Power Systems in Integrating Large-Scale Renewable Generations: A Critical Review on Concepts, Formulations and Solution Approaches. Renew. Sustain. Energy Rev. 93, 272–284. doi:10.1016/j.rser.2018.04.109
Li, Y., Han, M., Yang, Z., and Li, G. (2021). Coordinating Flexible Demand Response and Renewable Uncertainties for Scheduling of Community Integrated Energy Systems Witctric Vehicle Charging Station: A Bi-level Approach. IEEE Trans. Sustain. Energy 12, 4. doi:10.1109/tste.2021.3090463
Li, Y., Wang, R., and Yang, Z. (2022). Optimal Scheduling of Isolated Microgrids Using Automated Reinforcement Learning-Based Multi-Period Forecasting. IEEE Trans. Sustain. Energy 13 (1), 159–169. doi:10.1109/TSTE.2021.3105529
Li, Z., Shahidehpour, M., Aminifar, F., Alabdulwahab, A., and Al-Turki, Y. (2017). Networked Microgrids for Enhancing the Power System Resilience. Proc. IEEE 105 (7), 1289–1310. doi:10.1109/JPROC.2017.2685558
Liu, C., Lee, C., Chen, H., and Mehrotra, S. (2016). Stochastic Robust Mathematical Programming Model for Power System Optimization. IEEE Trans. Power Syst. 31 (1), 821–822. doi:10.1109/TPWRS.2015.2394320
Munné-Collado, I., Aprà, F. M., Olivella-Rosell, P., and Villafáfila-Robles, R. (2019). The Potential Role of Flexibility during Peak Hours on Greenhouse Gas Emissions: A Life Cycle Assessment of Five Targeted National Electricity Grid Mixes. Energies 12 (23), 4443. doi:10.3390/en12234443
Orfanos, G. A., Georgilakis, P. S., and Hatziargyriou, N. D. (2013). Transmission Expansion Planning of Systems with Increasing Wind Power Integration. IEEE Trans. Power Syst. 28 (2), 1355–1362. doi:10.1109/TPWRS.2012.2214242
Palmintier, B. S., and Webster, M. D. (2016). Impact of Operational Flexibility on Electricity Generation Planning with Renewable and Carbon Targets. IEEE Trans. Sustain. Energy 7 (2), 672–684. doi:10.1109/TSTE.2015.2498640
Richter, M., Oeljeklaus, G., and Görner, K. (2019). Improving the Load Flexibility of Coal-Fired Power Plants by the Integration of a Thermal Energy Storage. Appl. Energy 236, 607–621. doi:10.1016/j.apenergy.2018.11.099
Song, M., nejad, R. R., Sun, W., and Sun, W. (2021). Robust Distribution System Load Restoration with Time-dependent Cold Load Pickup. IEEE Trans. Power Syst. 36 (4), 3204–3215. doi:10.1109/TPWRS.2020.3048036
Takeshita, T., Aki, H., Kawajiri, K., and Ishida, M. (2021). Assessment of Utilization of Combined Heat and Power Systems to Provide Grid Flexibility Alongside Variable Renewable Energy Systems. Energy 214, 118951. doi:10.1016/j.energy.2020.118951
Tushar, M. H. K., Assi, C., Maier, M., and Uddin, M. F. (2014). Smart Microgrids: Optimal Joint Scheduling for Electric Vehicles and Home Appliances. IEEE Trans. Smart Grid 5 (1), 239–250. doi:10.1109/TSG.2013.2290894
Wang, J., Shahidehpour, M., and Li, Z. (2008). Security-constrained Unit Commitment with Volatile Wind Power Generation. IEEE Trans. Power Syst. 23 (3), 1319–1327. doi:10.1109/TPWRS.2008.926719
Wilson, I. A. G., McGregor, P. G., Infield, D. G., and Hall, P. J. (2011). Grid-connected Renewables, Storage and the UK Electricity Market. Renew. Energy 36 (8), 2166–2170. doi:10.1016/j.renene.2011.01.007
Zheng, Q. P., Wang, J., Pardalos, P. M., and Guan, Y. (2013). A Decomposition Approach to the Two-Stage Stochastic Unit Commitment Problem. Ann. Oper. Res. 210 (1), 387–410. doi:10.1007/s10479-012-1092-7
Keywords: power grid, flexibility, carbon, Heilongjiang, renewable energy
Citation: Liu Q, Ban M, Jiang H, Liu Y, Xu H, Guo J, Liu Y and Shi X (2022) Flexibility System for Heilongjiang Power Grid Considering Carbon Reduction Targets. Front. Energy Res. 10:933904. doi: 10.3389/fenrg.2022.933904
Received: 13 July 2022; Accepted: 03 June 2022;
Published: 13 July 2022.
Edited by:
Meng Song, Southeast University, ChinaReviewed by:
Changsen Feng, Zhejiang University of Technology, ChinaFu Shen, Kunming University of Science and Technology, China
Yi Zhao, Shenyang Institute of Engineering, China
Copyright © 2022 Liu, Ban, Jiang, Liu, Xu, Guo, Liu and Shi. This is an open-access article distributed under the terms of the Creative Commons Attribution License (CC BY). The use, distribution or reproduction in other forums is permitted, provided the original author(s) and the copyright owner(s) are credited and that the original publication in this journal is cited, in accordance with accepted academic practice. No use, distribution or reproduction is permitted which does not comply with these terms.
*Correspondence: Mingfei Ban, YmFubWluZ2ZlaUBuZWZ1LmVkdS5jbg==; Xingyu Shi, c3h5X2NzdXN0QGNzdXN0LmVkdS5jbg==