- 1TIFAC-CORE, Vellore Institute of Technology, Vellore, India
- 2Department of Electrical and Electronics Engineering, Thiagarajar College of Engineering, Madurai, India
- 3CPS Technologies, Brisbane, QLD, Australia
- 4Steering Committee (Hydrogen Society of Australia), Perth, WA, Australia
- 5Department of Electrical and Electronic Engineering, Universiti Teknologi PETRONAS, Seri Iskandar, Malaysia
- 6Department of Electrical and Electronics Engineering, Agni College of Technology, Chennai, India
- 7Sustainable and Renewable Energy Engineering Department, University of Sharjah, Sharjah, United Arab Emirates
- 8Research Institute for Sciences and Engineering, University of Sharjah, Sharjah, United Arab Emirates
Conventional fuels for vehicular applications generate hazardous pollutants which have an adverse effect on the environment. Therefore, there is a high demand to shift towards environment-friendly vehicles for the present mobility sector. This paper highlights sustainable mobility and specifically sustainable transportation as a solution to reduce GHG emissions. Thus, hydrogen fuel-based vehicular technologies have started blooming and have gained significance following the zero-emission policy, focusing on various types of sustainable motilities and their limitations. Serving an incredible deliverance of energy by hydrogen fuel combustion engines, hydrogen can revolution various transportation sectors. In this study, the aspects of hydrogen as a fuel for sustainable mobility sectors have been investigated. In order to reduce the GHG (Green House Gas) emission from fossil fuel vehicles, researchers have paid their focus for research and development on hydrogen fuel vehicles and proton exchange fuel cells. Also, its development and progress in all mobility sectors in various countries have been scrutinized to measure the feasibility of sustainable mobility as a future. This, paper is an inclusive review of hydrogen-based mobility in various sectors of transportation, in particular fuel cell cars, that provides information on various technologies adapted with time to add more towards perfection. When compared to electric vehicles with a 200-mile range, fuel cell cars have a lower driving cost in all of the 2035 and 2050 scenarios. To stimulate the use of hydrogen as a passenger automobile fuel, the cost of a hydrogen fuel cell vehicle (FCV) must be brought down to at least the same level as an electric vehicle. Compared to gasoline cars, fuel cell vehicles use 43% less energy and generate 40% less CO2.
1 Introduction
Sustainable mobility is described as a transportation system that is ubiquitous, effective, clean, and ecologically beneficial. Whilst transportation is not having its own sustainable development goals (SDGs), it is critical for accomplishing other SDGs in order to reach desired growth and development. Top-scoring countries for the SDGs have more robust and long-term mobility policies in place, whilst countries with the lowest scores are chastised for having inadequate transportation infrastructure (Sum4all.org, 2021). Figure 1 depicts the SDGs that are directly or indirectly met by sustainable transportation.
The origin of “sustainable mobility” is from the broader definition of “Sustainable development”. “Sustainable development” is “development that meets current needs without jeopardizing the ability of future generations to meet their own needs” (World Commission on Environment and Development, 1987). The infographic (Figure 2) depicts the broad benefits of sustainable mobility (Ransformative-Mobility, 2021), which include energy security, economic development, environmental sustainability, and social wellbeing.
The literature has a number of researches on sustainable mobility. The scope of technology in fostering a change in behaviour toward sustainable transportation has been investigated (Klecha and Gianni, 2018; Chng, 2021). Gonzales Green port strategies for reducing negative externalities in the countryside has been investigated (Gonzalez Aregall et al., 2018). Table 1 lists the results of several studies on sustainable mobility.
1.1 Hydrogen: The Most Reliable Form of Energy
The global need for energy has risen intensely with the growth of the world’s population. This is because energy is required for all activities. The great majority of energy is imitative from fossil fuels, which are non-renewable resources that take longer to recharge or reoccurrence to their previous capacity. Energy imitative from fossil fuels is less costly; however, it has shortcomings when compared to renewable energy sources (Rohith et al., 2016).
Hydrogen is an emerging and almost established fuel source for cars (Apostolou and Xydis, 2019; Staffell et al., 2019; Falcone et al., 2021). The present state of the art and future possibilities of the burgeoning hydrogen-based market in road transportation, as well as an examination of existing hydrogen refuelling station technologies, have been explored (Apostolou and Xydis, 2019). The hydrogen economy offers a multi-sectoral view of low-cost clean energy and thorough decarbonization in process sectors. The ability to store hydrogen or derivatives is a game changer for the integration of high renewable energy source shares, resulting in beneficial effects on various SDGs through lower GHG and air pollution emissions (Falcone et al., 2021). Along with biofuels and electric cars, hydrogen is one of three key low-carbon transportation choices (EVs). Hydrogen avoids the negative effects of biofuels on land usage and air pollution, as well as the restricted range and long recharge periods associated with electric vehicles (Staffell et al., 2019). Hydrogen automobiles have been shown to have a threefold lower potential for global warming than other alternative technologies (Bicer and Dincer, 2017; Dincer, 2020; Apostolou and Welcher, 2021). In Denmark, variables that may influence public acceptability of hydrogen-powered cars have been explored. To that purpose, four primary hypotheses were proposed, assuming that variables such as technical and environmental knowledge, financial standing, and infrastructure have a direct impact on the societal acceptability of hydrogen-powered private road cars in the transportation sector. Most of the hypotheses, such as environmental awareness, limited refuelling infrastructure, and media backing for this sector, were supported by the findings (Apostolou and Welcher, 2021). To explore the effects of alternative cars on the environment and human health, a life cycle evaluation of methanol, hydrogen, and electric vehicles is done. The findings of this study demonstrate that owing to the manufacturing and maintenance phases, electric cars have higher human toxicity ratings. Because hydrogen has a higher energy density than methanol, hydrogen-powered cars are a more environmental sustainable alternative in terms of global warming and ozone layer depletion (Bicer and Dincer, 2017). The Covid-19 coronavirus has made it more important than ever for people to breathe cleaner air, drink cleaner water, eat cleaner food, and use cleaner energy. We were in a carbon age with hydrocarbon fuels until the coronavirus outbreak juncture in 2020, and now we must continue to change the driver to hydrogen, which is the start of the hydrogen age, in which the use of hydrocarbon fuels (fossil fuels) will decrease exponentially while the use of hydrogen energy will increase (Dincer, 2020). The Covid-19 has thrown the transition from the carbon (C) age to the emerging hydrogen (H2) age into disarray (Apostolou et al., 2018).
As a result, renewable resources, particularly hydrogen energy, are the most promising choices for meeting energy demands. Hydrogen is found mainly in plant materials and is rare in nature. Hydrogen is a non-metallic, nontoxic fuel that can provide more energy per unit of mass than gasoline (Abdalla et al., 2018). However, a substantial study is required to investigate and design onboard applications in order to use hydrogen as a fuel.
Hydrogen has lately emerged as a prospective energy carrier, and the organic chemical hydride approach offers significant advantages in terms of transportability and handling (Morel et al., 2015). The possibilities for combining stochastic power generation with hydrogen production, storage, and consumption is explained in (Korpas and Gjengedal, 2006).
1.2 Hydrogen as a Fuel in the Transportation Sector
“Centralized” production, where hydrogen is produced on a large scale and supplied to customers via truck or pipeline. “On-site” or “distributed” production, is where hydrogen is produced at the end-use site, generally through small-scale electrolysis or steam methane reforming (Meraj et al., 2020).
Hydrogen can also be converted to other energy carriers like electricity, methane, or liquid fuels, which incurs conversion costs and efficiency losses but allows access to existing energy distribution networks without requiring the construction of an extensive hydrogen distribution infrastructure. The relative cost of regional basic resources for hydrogen generation and policies is vital in determining the ideal hydrogen supply pathway. Transportation of hydrogen can be done using.
1) Pipelines (Weinmann, 1999).
2) Mobile by trucks, trains, vessels (Domashenko, 2002).
The use of hydrogen in onboard vehicles has hurdles owing to the high weight, volume, and cost of hydrogen. Furthermore, as the refuelling process continues, the life cycle of hydrides shortens, reducing the vehicles’ efficiency. Another disadvantage is the lack of adequate hydrogen storage system standards and protocols. The infrastructure to distribute hydrogen to the user requires entirely new infrastructure; the production and delivery systems must be integrated to reduce the cost of hydrogen delivery and distribution costs. At the moment, hydrogen transportation, storage, and delivery to the site of consumption are all related with inefficient energy use. Despite having some notable disadvantages, hydrogen is heavily used in several industries such as the transportation industry, power generation industry, and building industry instead of conventional fossil fuels (Reddy et al., 2020).
This paper aims to present the future of hydrogen energy as a solution to sustainable mobility. Existing literatures are mainly focusing on utilization of hydrogen for a particular sector of transportation; the overall transportation sector is not addressed. In detail analysis of techno-economic-environmental aspects of Hydrogen as sustainable mobility solution is missing in recent literature. The goal of this research is to evaluate the potential of hydrogen energy as a solution for sustainable transportation and to analyse its environmental and social consequences. This review aims to introduce the preparation processes, storage method, and critical technical issues of its application in vehicles and related mobility sectors in a systematic manner, providing exciting insights into hydrogen-based energy, the potential large-scale deployment process on a global scale including techno-economic aspects, selected implemented projects, policies and challenges. The paper helps the policymakers and industries decide on choosing hydrogen as the future of sustainable mobility.
This paper is structured as follows. Section 2 provides an in-depth review of hydrogen as a fuel for transportation in many sectors and its environmental and technical elements. Section 3 deals with the generation and storage of hydrogen energy. Section 4 concludes the in-depth analysis to suggest the scope of hydrogen to be adopted as the future of sustainable mobility.
2 Environmental Aspects
Considering the fact of depletion of energy resources and the rise in global warming, challenges are encountered with the combustion of energy due to the transportation sector in the present time. Green House Gas (GHG) emissions are caused by the dominant conventional road transportations, which have existed for more than a century and has reached its upper saturation level. As per the International Energy Agency regulations, global carbon dioxide emissions must be decreased to limit the consequences of climate change (Zhao et al., 2020). To enhance the technology that has zero pollutant discharge and zero climate change effect, hydrogen Fuel cell-based vehicles production is being promoted by automotive industries. The government of various countries like the United States, Japan and South Korea have encouraged the production of Hydrogen vehicles since 2018 (Meng et al., 2021). The upcoming subsections discuss the environmental and technical aspects of hydrogen in the mobility sector and its scope.
Transportation is the second-largest source of pollution in terms of GHG emissions, posing a serious threat to human health (Roadmap to a Single European Transport Area-Towards a competitive and resource efficient transport system, 2021). The transportation sector accounts for 23% of total CO2 emissions. According to the report, the transportation sector would continue to rely on petroleum-based services for 90% of its fleet, with renewable energy sources accounting for only 10%. By 2050, carbon emissions from the transportation industry are predicted to be 33% more than they were in 1990 (Le Quéré et al., 2020).
2.1 Climatic Change and Greenhouse Gas Emissions
Climate change is the central point of focus in the present era due to the advancement of technologies in various sectors, including the transportation industries. The combustion of biofuels for transportation has captured the mobility sector for the last two centuries. Traditional combustion fuels by vehicles lead to the generation of pollutants and GHGs to the environment, which has various adverse effects (Engel, 2012; Zhongfu et al., 2015). Hydrogen is a carrier, like electricity, rather than an energy source, and the notion of “hydricity,” or the inherent interchangeability of electricity and hydrogen, has been established (Engel, 2012). Anthropogenic GHG emissions are directly linked to the global warming trend. Climate change caused by GHG emissions is one of the most serious environmental issues confronting modern society (Ding et al., 2018). In order to stabilize the climate, it is the need of the time to reduce the emissions significantly (Liu et al., 2019). CO2 is the major GHG contributor with a value of 76%, methane, while Nitrous Oxide and fluorinated gases together contributes the rest 24% (Global-Greenhouse-Gas-Emissions-Data, 2021). As illustrated in Figure 3, the global CO2 concentration is growing rapidly.
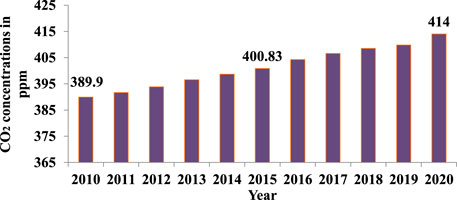
FIGURE 3. Concentration of CO2 in atmosphere in ppm (Climate-Change-Atmospheric-Carbon-Dioxide, 2021; Health-Topics, 2021)
The concentration of CO2 in the atmosphere is currently at 414.00 ppm, the highest in the previous 800 k years, and it is closely connected to global temperature. The world has committed to keeping global warming below 2°C, and this goal can be met with a minimal carbon budget. According to researchers, mankind can only emit 565 Gt of CO2 more and still meet the 2°C target—a limit that would be exhausted in 15 years if emissions continue at their current rate of 36.6 Gt CO2 per year (Liu et al., 2019). It is also predicted that seven million people die each year as a result of illnesses caused by air pollution (Global-Energy-Related-Co2-Emissions, 2021).
During COVID-19, there is a temporary decline in daily global CO2 emissions due to forced confinement. By early April 2020, daily global CO2 emissions had decreased by 17% compared to the mean levels in 2019, with surface traffic accounting for half of the decline (Saleem et al., 2021). However, in the post-COVID-19 scenario, things will be different. As a result, adopting a sustainable transportation strategy is critical.
Although GHGs are released by a variety of sources, those produced by automobiles can be reduced by employing alternative fuel vehicles (AFVs) or green vehicles. Advanced alternative fuel technologies have the potential to halve gasoline use while also cutting CO2 emissions and their associated environmental consequences. Although fuel-efficient technologies help vehicles perform better in terms of environmental performance, they cannot assist cut overall emissions. This is ought to the fact that technology cannot change consumer habits on its own. So, framing a strategy that encourages consumers to choose energy-efficient vehicles over traditional one is critical, as is ensuring the use of AFVs that complies with environmental pollution-reduction measures like carpooling and using low-CO2-emitting vehicles, public transportation, or bicycles to save fuel (Oliveira and Dias, 2020; García-Melero et al., 2021; Apostolou and Xydis, 2019).
2.2 Hydrogen as a Zero-Emission Source
Hydrogen energy follows a zero-emission policy towards the environment, making it a fundamental attraction for researchers and industries to study and develop hydrogen transport technologies. Additionally, hydrogen has become a truly sustainable energy resource because of the zero climate change effect, as hydrogen is a highly efficient, reliable, and soundless source of power.
The evaluation of hydrogen fuel transportation cannot be alone evaluated based upon the tailpipe gas emissions. The environmental aspects can be accounted for based on the vehicle’s wheel to tank evaluation (Concawe and JRC, 2007; Yang and Ogden, 2007; Bethoux, 2020). Natural hydrogen may become a viable economic option, making fuel cell vehicles a viable and ecologically acceptable alternative to battery electric vehicles (Bethoux, 2020). The European Commission’s Joint Research Centre, EUCAR, and CONCAWE have assessed the tank-to-wheels (TTW) energy usage and greenhouse gas emissions for a variety of future fuel and powertrain alternatives (Concawe and JRC, 2007). Moving our transportation sector away from petroleum-derived gasoline and diesel fuels and toward hydrogen derived from domestic primary energy resources can have a number of societal benefits, including lower well-to-wheels greenhouse gas emissions, zero point-of-use criteria air pollutant emissions, and less imported petroleum from politically sensitive areas (Yang and Ogden, 2007). Therefore, the accountability of hydrogen vehicles towards environmental effect has been studied and reported through one tool known as Ecoscore. The tool has been developed by the commission of the Flemish government (Sergeant. et al., 2009). This measurement is based upon the GHGs emissions, regulation of air quality and sound pollution. Apart from the three major aspects, two indirect aspects, including human lifecycle and ecosystem maintenance, are also included in the Ecoscore measurement scale (Van Mierlo et al., 2004). Environmental aspects of hydrogen vehicles are presented in Figure 4.
3 Technical Aspects
The study about hydrogen fuel vehicles has major issues related to high-pressure hydrogen storage. To tackle the problem of hydrogen storage, researchers have proposed the onboard hydrogen generation engines (Frenette and Forthoffer, 2009; Shusheng et al., 2020). The fuel cell electric vehicle is an onboard hydrogen-generation type in the design scheme that provides rapid hydrogen supply. Moreover, a self-heating reforming technology combining methanol vapour reforming and partial oxidation reforming being utilized (Özcan and Garip, 2020). The car is powered by a hybrid system that includes a lithium battery and a hydrogen fuel cell. The aforesaid approach is different from hydrogen storage fuel cell vehicles. It eliminates the hydrogenation process and the high-pressure hydrogen storage device, and drives the motor with the fuel cell as the primary power source, while the lithium battery as a backup. Based on the structure of the fuel cell electric vehicle designed in the literature (Li et al., 2016; Shusheng et al., 2020), the vehicle’s critical components, such as a hydrogen production system, electric drive system, auxiliary power supply, and management system, were evaluated, and their management and control techniques were described.
3.1 Production of Hydrogen
Hydrogen may be produced using both renewable and fossil fuel technologies. Steam reforming, partial oxidation, auto thermal oxidation, and gasification are all methods for producing hydrogen from fossil fuels. By gasifying biomass/biofuels and splitting water with solar or wind energy, hydrogen can be synthesized from renewable energy sources (Apostolou, 2020). The Hydrogen production sources and technologies are shown in Figure 5.
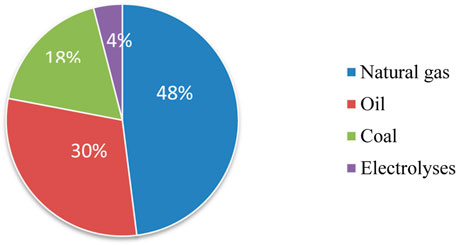
FIGURE 5. Hydrogen production sources (Arat and Sürer, 2017)
The extraction of hydrogen from coal is the highest among all sources, approximately 21.5 billion tons/year, which need to be replaced by renewable resources.
3.1.1 Categorization of Hydrogen Based on the Source of Generation
By the adaptation of different technology and considered sources, hydrogen production has been categorized into three types according to the literature and study reports (von Döllen et al., 2021; Noussan et al., 2021). The utilization of major sources for the production of hydrogen has introduced the color conceptualization. By the application of fossil fuel for the generation of hydrogen leads to the emission of CO2 and greenhouse gases. This technology of hydrogen production and its utilized source refers to grey hydrogen (Ivanenko, 2020). Blue hydrogen was introduced, while the grey hydrogen production approach was used to lower the quantity of greenhouse gas emissions in hydrogen production (Mari et al., 2016; Dickel, 2020). The utilization of fossil fuel, industrial gas, by-product gas, natural gas for hydrogen production for sustainable mobility as energy resources generally emit pollutants, and greenhouse gases to the environment (Jovan and Dolanc, 2020; Luo et al., 2020; Schiro et al., 2020). In a case study of a Slovenian hydro power plant, the possibility for green hydrogen generation was examined. If it is not burdened by different environmental fees, hydrogen can be competitive in the transportation sector (Jovan and Dolanc, 2020). Renewable hydrogen generation is a reliable alternative since this energy vector can be quickly created from electricity and injected into existing natural gas infrastructure, allowing for storage and transit (Schiro et al., 2020). The economic analysis of hydrogen was applied to hydrogen produced by natural gas, coal, and water electrolysis and conveyed in the form of high-pressure hydrogen gas or cryogenic liquid hydrogen. The cost of hydrogen produced from natural gas and coal is now cheaper, but it is heavily influenced by the cost of hydrogen purification and the price of carbon trading. Given the impact of future production technologies, raw material costs, and rising demands for sustainable energy development on hydrogen energy costs, it is suggested that renewable energy curtailment be used as a source of electricity and multi-stack system electrolyzers be used as large-scale electrolysis equipment, in combination with cryogenic liquid hydrogen transportation or on-site hydrogen production (Luo et al., 2020). To reduce these pollutants affecting the atmosphere, literature has reported various technological modifications for hydrogen generation. Therefore, the utilization of renewable energy resources for hydrogen generation has been reported in recent works of literature (Boretti, 2020; Manna et al., 2021; Rabiee et al., 2021). Green hydrogen is also resultants of electrolyzers produced by renewable energies. It has been also noticed that green hydrogen can be also produced from bioenergy such as biomethane and biomass combustion. As the green hydrogen generated from various methodologies has net-zero gas emission, researchers and industries have more attention towards its production advancement (Manna et al., 2021; Rabiee et al., 2021). Categories of Hydrogen generation is presented in Figure 6.
Hydrogen production from different sources and emission from it is tabulated in Table 2.
At present, coal is the primary source of hydrogen extraction, but the process emits GHGs. Hydrogen extraction through photocatalytic water decomposition with solar energy is the least popular process, with 1.8 billion tons of hydrogen annually. Table 2, concludes that the hydrogen production from photocatalytic water decomposition with solar energy is emission-free and the most sustainable path.
3.1.2 Water Electrolysis to Generate Hydrogen
Water as a feedstock is one of the most environmentally beneficial ways to produce hydrogen as it releases only oxygen as a by-product during processing. Green hydrogen is hydrogen produced by the breakdown of water using renewable energy sources. Electrolysis is currently the most established commercially accessible process for producing hydrogen from water. Water electrolysis is the process of breaking down water (H2O) into its constituent’s hydrogen (H2) and oxygen (O2) using electric current (Hydrogen-Production-Through-Electrolysis, 1927). Positive ions (H+) are drawn to the cathode, whereas negative ions (OH-) are drawn to the anode by the electric potential. Alkaline water electrolysis (AEL), proton exchange membrane (PEM) water electrolysis, solid oxide water electrolysis (SOE), and alkaline anion exchange membrane (AEM) water electrolysis are some of the water electrolysis procedures (Chi and Yu, 2018) as depicted in Figure 7.
Comparative analysis of different water electrolysis processes to generate hydrogen (Hydrogen-Production-By-Electrolysis-Ann-Cornell, 2017; Hydrogen-Production-Through-Electrolysis, 2017; Articlelanding, 2020) is tabulated in Table 3.
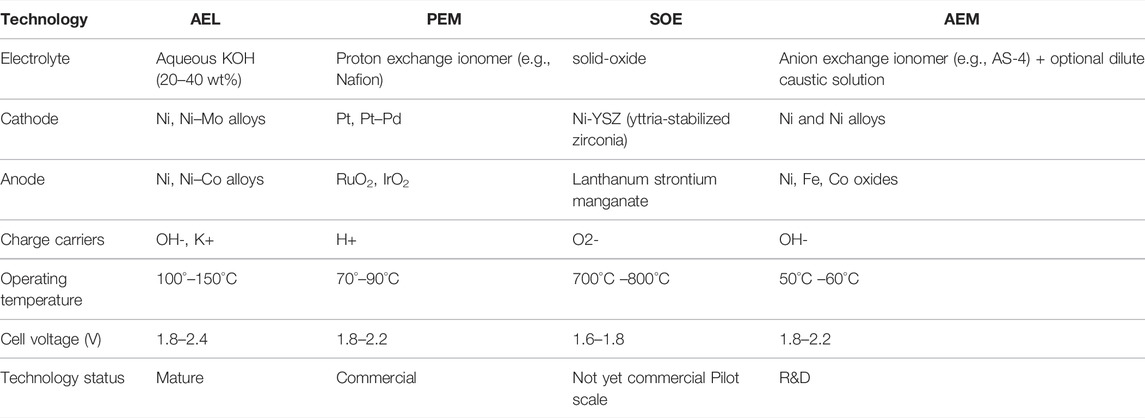
TABLE 3. Comparative analysis of different types of water electrolysis process to generate hydrogen.
Use of AEM water electrolysis could allow low-cost transition metals to replace traditional noble metal electrocatalysts (Pt, Pd, Ru, and Ir). AEM electrolysis has garnered special interest due to its high power efficiency, membrane stability, durability, ease of handling, and low-cost hydrogen-production method (Vincent and Bessarabov, 2018), despite being a developing technology. Aside from the high energy consumption induced by the rise in electrolysis voltage generated by the bubbles developed during the electrolysis process (Hu et al., 2019), high energy consumption is another barrier of hydrogen synthesis from water electrolysis. Hydrocarbons can be used in water electrolysis to reduce energy usage. Cheap metals or nonmetal composite materials, such as Ni, should be the electrodes’ likely future direction.
The following are the major future directions to be investigated in the water electrolysis process of hydrogen generation:
• In-depth investigation of the reaction process in order to improve hydrogen generation efficiency and achieve conversion by combining chemical and electrical energy;
• Reduction in energy intake in the electrolysis process of water using renewable energy;
• In-depth investigation of the reaction process in order to improve the efficiency of hydrogen production;
• Improvements in electrode stability and corrosion resistance for increased longevity and lower electrode costs;
• Development of new catalytic electrodes and catalysts to improve reaction efficiency (Gao et al., 2019; Huang et al., 2019).
Hydrogen power is a promising technique for storing fluctuating Renewable Energy (RE) to establish a 100% renewable and sustainable hydrogen economy (Dawood et al., 2020). Hydrogen can be stored as gas or liquid form. High-pressure tanks (350.00–700.00 bar tank pressure) are primarily used to store hydrogen as a gas. Cryogenic temperatures are required to store hydrogen as a liquid (Hydrogen Storage, 2021). Sapru (2002) have given an summary on hydrogen storage systems, based on storage tanks integrated with fuel cells.
3.2 Hydrogen Storage
Hydrogen holds excellent potential to be an energy carrier, especially for fuel cell applications. With high calorific value, it is also termed as regenerative and environmentally friendly fuel. Additionally, it has energy density of 142 ML/kg, which is three times of petroleum (47 MJ/kg) (Kaur et al., 2016). This makes hydrogen as the most efficient fuel to replace petroleum-based vehicular. Thus, fossil fuel reliability can be reduced to fulfil all the global energy demands (Muir and Yao, 2011). Carbon and Hydrogen cycle are shown in Figure 8. The combustion process is shown below in blue arrows. The cycle shows how CO2 released causes global warming (presented by black arrows). On the other hand, the hydrogen cycle is presented by green arrows and pointing towards renewable energy sources (Kaur et al., 2019).
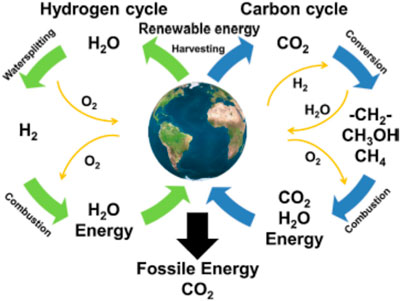
FIGURE 8. Carbon and hydrogen cycle (Muir and Yao, 2011)
Hydrogen energy has also been projected as a widespread resolution for a secure energy future to increase energy security and strengthen developing countries’ economies (Marrero-Alfonso et al., 2009). Various technological, significant scientific, and economic challenges must be overcome before hydrogen can be used as a clean fuel source and the transition from a carbon-based fossil fuel energy system to a hydrogen-based economy can be completed (Shashikala, 152012).
Additionally, in the transportation sector, hydrogen storage technologies are in consideration and gradually move towards designing highly efficient systems. For example, specific criteria are looked into, such as thermal stability of the system, gravimetric and volumetric densities and cost of the operating systems. Many of these sectors are being worked on, and improvements in hydrogen production and storage for various automotive applications have been made and deployed (Shang and Chen, 2006).
3.2.1 Hydrogen Storage Methods
Hydrogen storage methods can be categorized into three groups, as shown in Figure 9. Molecular hydrogen can be stored as (1) a gas or a liquid without any significant physical or chemical bonding to other materials; (2) molecular hydrogen can be adsorbed onto or into material and held in place by relatively weak physical van der Waals bonds; (3) atomic hydrogen can be covalently bound (absorbed). The spread of hydrogen fueling stations across the transportation network, as well as investment in hydrogen fueling stations, can lead to increased profits (El-Taweel et al., 2019).
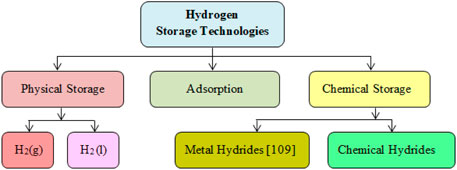
FIGURE 9. Hydrogen storage technologies (Andersson and Grönkvist, 2019; Lototskyy et al., 2017)
3.2.1.1 Compressed/Physical Hydrogen Storage
Hydrogen is stored at high pressure and in compressed form and specifically designed hydrogen cylinders reinforced by carbon fibre that can withstand very high pressure. Various concerns should be handled before using this technology, like high-pressure requirements, low volumetric density, energy required to compress hydrogen gas, and cylinder weight and to reduce the overall cost (Sakintuna et al., 2007).
Hydrogen can also be stored in cyro-compressed form by cooling hydrogen gas to −253°C; this process increases the volumetric storage capacity of hydrogen gas by 4 times. However, this process is highly energy intensive due to energy requirements for compressing and liquifying hydrogen gas. There are further limitations, such as liquid hydrogen being very volatile and potentially forming an explosive combination with air if evaporated. Thus, this system should be designed to cover all the safety concerns (Sakintuna et al., 2007). Figure 10 shows hydrogen gas in the form of compressed gas and cryogenic liquid.
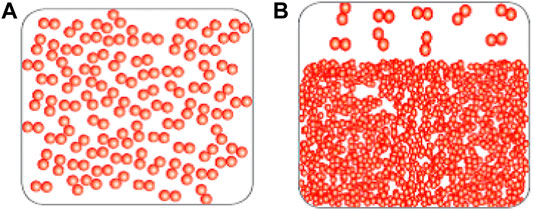
FIGURE 10. (A) Compressed hydrogen gas storage and (B) cryo-compressed hydrogen gas storage (Sakintuna et al., 2007)
The weight, volume, cost, efficiency, codes, and standards are the primary problems in hydrogen storage. New materials, particularly polymers, must be developed as barrier materials to limit hydrogen leakage in storage tanks with high energy-to-weight ratios (Macher et al., 2021).
3.2.1.2 Material Based Hydrogen Storage
In materials, hydrogen is generally stored as absorption, adsorption, and chemical reaction. If hydrogen is stored on the surface, then the phenomenon is called adsorption, and if it is stored within the solids, it is called absorption. The main difference is in the density as it increases from adsorption to absorption. Adsorption is further divided into chemisorption and physisorption based on their mechanism. Physiosorbed hydrogen is weakly bonded than chemisorbed hydrogen molecules. Also, it involves highly porous materials with high surface areas to efficiently uptake and release hydrogen molecules from the materials, such as metal hydride hydrogen storage.
However, absorption involves hydrogen atoms attached with strong bonds within the chemicals. Here, hydrogen is stored in large amounts with small quantities of materials also could be released at low temperature and pressure. For example, in complex and chemical hydrides, hydrogen is absorbed in the materials, as shown in Figure 11 (Klanchar et al., 2004).
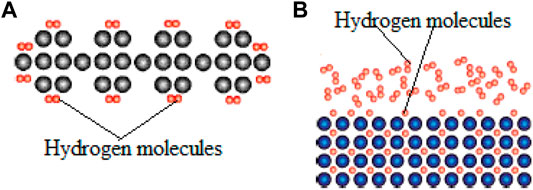
FIGURE 11. (A) Surface adsorption and (B) Surface absorption (Klanchar et al., 2004)
3.2.1.3 Chemical Hydrogen Storage Pathway
When hydrogen is generated and released through the chemical reaction, then the storage technology is defined as chemical hydrogen storage. The basic reactions involve the reaction of chemical hydrides with water and alcohols. However, this technology suffers lack of reversible onboard reactions and require spent fuel and by-products to be removed off-board. Here, hydrogen is strongly bonded as hydrogen atoms within the molecular structures of the chemical compounds, as presented in Figure 12. Therefore, for hydrogen generation and storage, a chemical reaction is required.
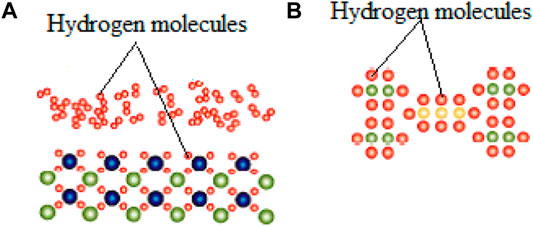
FIGURE 12. (A,B): Absorption in complex chemical hydrides (Klanchar et al., 2004).
As hydrogen is stored in chemical hydrides, these hydrides in the form of materials have high gravimetric and volumetric densities. Thus, hydrogen is released in the form of chemical reactions. There are two methods of hydrogen release; the first is hydrolysis, and the second is thermolysis. The former one requires low temperature and pressure, and the theoretical storage efficiency is very high. The latter one involves highly sophisticated technologies and energy requirements to break the hydrides by thermal pathways. Considering that few common chemical hydrides that release hydrogen by hydrolysis pathway are sodium, lithium, magnesium, calcium, titanium hydrides, and few of complex hydrides are sodium borohydride, lithium aluminium hydride and lithium borohydride (Klanchar et al., 2004).
Figure 13 presents various hydrides per their volumetric storage densities, with AlH3 having the highest value and pressurized tanks with the lowest values (Graetz, 2012). As shown in Figure 14.
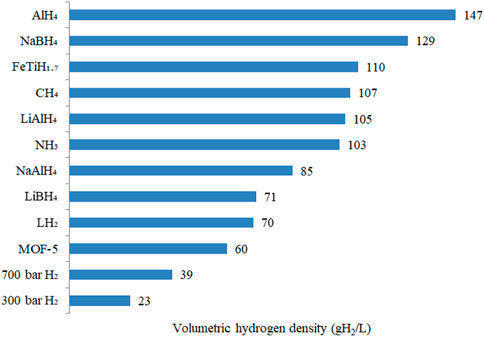
FIGURE 13. Volumetric hydrogen density of various hydrogen storage methods (Graetz, 2012).
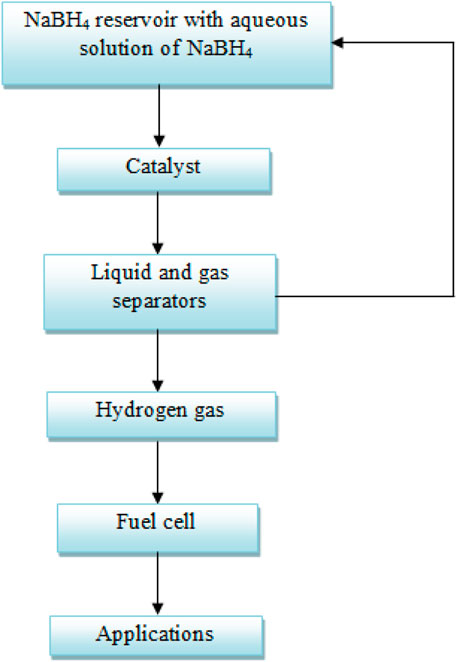
FIGURE 14. Flow diagram of solid-state hydrogen generation system (Demirci and Miele, 2009).
The catalyst for the reaction is supplied in the form of an aqueous solution of NaBH4 as a chemical hydride. This solution is run through a separator, which separates the pure hydrogen gas from the rest of the mixture. This pure hydrogen gas is then pumped into the fuel cell, where it can be used. After the recycling procedure, the by-products might be returned to the liquid reservoir and used again (Demirci and Miele, 2009).
As a clean resource, hydrogen energy might minimise energy savings and emissions caused by the use of fossil fuels, and it will likely play an increasingly important role in the future (Zhang et al., 2019a). In recent decades, the PEMFC (proton exchange membrane fuel cell or polymer electrolyte membrane fuel cell), which effectively transforms the chemical energy inherent in hydrogen into electricity without producing pollutants, has piqued interest in automotive applications (Ogungbemi et al., 2021).
3.3 Proton Exchange Fuel Cells
FCVs (fuel cell vehicles) powered by PEMFC have recently reached mass production, such as Toyota’s Mirai, Honda’s Clarity, and Hyundai’s NEXO. Performance should be increased at a cheaper cost to improve its commercial uses.
The European Union and the US alone stand out alone in the majority to consume all petroleum products and energy demands. This has led to the development of alternative energy sources, with the best ones stated as hydrogen, synthetic fuels and biofuels. These energy sources are investigated for their suitability to sustain a clean form of energy (Ogungbemi et al., 2021). The source where hydrogen is produced from renewable energy to electricity by PEM fuel cells are under investigation. The PEM cells are capable of producing sufficient power to sustain commercial and residential usage under varying temperatures. For example, a PEM fuel cell generator with Na metal and water chemical reaction generated hydrogen with minimum emissions and noise. This have extended its use to medium and high duty vehicular applications. Despite all such uses, PEM fuel cells face few disadvantages (Ijaodola et al., 2019) and studies are in progress based on economics, policy framework and advancements in electrolysis process to facilitate the use of PEM fuel cells for vehicular and general use. Due to myriads of advantages like low operating temperature, solid electrolyte and high power density, durability and reliability proton exchange, fuel cells can be used in several areas like on-site hydrogen generation, automotive, and portable electronic devices as discussed. The parameters of PEM fuel cells are based on operating conditions, and to accurately estimate its characteristics; research is also in progress with efficient mathematical modelling. It can disclose more about operating parameters linked with the PEM fuel cells (Rao et al., 2019a; Kandidayeni et al., 2019). Studies are also in progress to study the dynamic loading on the performance of PEMFC (Zhang et al., 2019b; Huang et al., 2020). The situation arises when unreasonable loading conditions increase and could even lead to failure (Mayyas et al., 2014) thus, adding to the disadvantages of the fuel cells. This could be explained as when PEM FC is used as a mechanical power source, it undergoes dynamic loading and response voltage becomes lower than the steady-state conditions; subsequently, the voltage increases gradually. This could lead to unfavourable operations of PEM fuel cells, and thus, dynamic performance needs to be studied.
Proton Exchange fuel cells have wide application in various sectors like power plants, transportation, digital devices etc. However, the short life span due to the degradation and reusability of fuel cells limits its applications in the commercial sector (Chen et al., 2019). Considering the lifespan of fuel cells in mobile applications, it is 3,000 h, but the demand rises to 5000 h to be used commercially. As per DOE (United States Department of Energy), the set future goals for transportation and stationary applications of PEM fuel cells as 5,000 h and 40000 h, respectively, by 2020 with performance degradation that should be less than 10% (Ren et al., 2020). The considerable difference between stationary and transportation can be attributed to different designs as fuel cells in vehicles encounters harsh conditions like the open-circuit voltage, dynamic load, startup and shutdown, overload and freezing thaw. Thus, the decay of fuel cells in vehicular applications is also thoroughly studied by developing various test protocols. With the performance of fuel cells, cost factors are also in consideration like The Strategic Analysis Inc. studied the most influencing factors on the cost of FC’s in 2012 and 2017 (Li et al., 2020). The report (2012) concluded that the fuel cell stack and platinum loading are the most important factors which affect the cost of the fuel cells. Since then, the study has also focused on a low Pt-based catalyst that made significant development in Pt-M alloys, Pt-based core shell, and Pt-based nanostructure. Gradually this development led to Pt-free catalysts like carbon alloy catalysts in commercial markets in Japan. Thus, it can be stated that PEM fuel cells holds the potential to establish a hydrogen economy for a secure and sustainable future.
4 Hydrogen Based Mobility
Promotion of hydrogen vehicles as the future transportation platform has been chosen due to its zero-pollutant discharge characteristic. Hydrogen fuel cell vehicle technology has the scope to various categories of mobility sectors. This technology can replace lightweight vehicles, heavy goods vehicles, heavy passenger vehicles, trains, and unmanned vehicles. Adopting the concept of hydrogen fuel for heavy vehicles has attracted New Zealand and Paris to meet the zero climate change commitments. As reported in (MBIE, 2019), the proper examination of feasibility for adopting clean hydrogen for heavy vehicles exceeds 30 tons has been discussed in (Concept Consulting Group, 2021; Perez et al., 2021).
Apart from the development of hydrogen fuel, heavy and very heavy-duty vehicles, public lightweight vehicles, and passenger buses require a shift towards utilization of clean hydrogen (Topler and Lordache, 2017; Air Liquide Will Build the First High-pressure Hydrogen Refueling Station for Long-haul Trucks, 2021). Air Liquide has announced the opening of Europe’s first high-pressure hydrogen filling station, which will support the first fleet of long-haul hydrogen vehicles (Air Liquide Will Build the First High-pressure Hydrogen Refueling Station for Long-haul Trucks, 2021). This investment is in line with the Group’s objective of accelerating hydrogen energy adoption through large-scale initiatives, notably in the heavy vehicle category. Vulnerabilities of Hydrogen Energy in Emerging Markets describes strategies and developments for hydrogen civilization efforts implemented by various stakeholders in different countries and at different stages of the development cycle, including authorities, institutes, research, industry, and individuals (Topler and Lordache, 2017). Considering these facts, Germany has taken the lead in the global market for the commercialization of Hydrogen vehicles, along with the collaborators from Japan, Korea and the United States (Galich and Marz, 2012; Trencher and Edianto, 2021). Hydrogen and fuel cell technologies have the potential to help create a more environmentally friendly and emission-free transportation and energy system (Galich and Marz, 2012). Policymakers and automotive players throughout the world attempt to expedite the electrification of road transport using hydrogen (Trencher and Edianto, 2021). They examined and contrasted the factors impacting the production and market penetration of privately owned fuel cell electric passenger vehicles (FCEVs) and fuel cell electric buses (FCEBs) in public transportation fleets.
4.1 Hydrogen Vehicles
In the present time, railway is the most economical transportation preferred by the common citizen as well as it is also used for goods transportation. The conventional railway depends upon the fossil fuels leads to the emission of GHGs and the generation of sound pollution. In the line, to meet zero-emission and zero sound railway transportation, InnoTrans in 2016 of Berlin developed the Coradia iLint. This has been commercialized and launched in 2020 to run for 100 km between Cuxhaven, Bremerhaven, Bremervoerde and Buxtehude in northern Germany (Low et al., 2020). Understanding the requirement and need for replacing clean hydrogen fuel transportation with the second largest railway network in the world, the Indian Railway has also started developing and testing passenger fuel cell train set (Jhunjhunwala et al., 2018).
Conventional fuel engines utilized in the shipping industries release the air pollutants and GHGs into the environment. In the regulation of these harmful gases, International Maritime Organization (IMO) has passed an article to prevent pollution from the ships under (World’s First Hydrogen Train Runs Route in Germany, 2021; Traction: India to trial fuel cell trainset, 2021). Enhanced efficiency of the marine fuel cells for various applications of onboard ships has motivated the researchers to focus on hydrogen fuel-based marine engines. Electricity generation, emergency power supply and power propulsion are the major power requirement in an onboard ship, which can be generated through clean hydrogen fuel cells by replacing conventional fuels (Sattler, 2000; IMO, 2012a; IMO, 2012b). Fuel cells have a lot of potential for usage on ships. Fuel cells on merchant ships and naval surface ships can be used for a variety of purposes, including: (1) emergency power generation; (2) electric energy generation, particularly in waters and harbours with strict environmental regulations; (3) small power output for propulsion in special operating modes (e.g., very quiet run); and (4) electric power generation for the ship’s network and, if necessary, the propulsion network on ships equipped with fuel cells (e.g., naval vessels as all-electric ships, AES) (Sattler, 2000). The actual requirement, replacement and advantage of combustion fuel engine by clean hydrogen fuel cell-based engine have been discussed in detail in (Leo et al., 2010; Tronstad et al., 2017). Submarines are now the most common marine use of fuel cells. In this industry, hydrogen/oxygen polymer electrolyte membrane (PEM) fuel cells are often utilized (Leo et al., 2010). The scope of hydrogen fuel in different mobility sectors is depicted in Figure 15.
The target to achieve limited emission fuel to protect the climate, world aviation industries have also focused on clean hydrogen as an efficient candidate for short and long-range aviation and space transportation (de-Troya et al., 2016). The research and development in aviation and space sector industries have reported continuous progress for choosing clean hydrogen fuel. The aviation and space industries of the United States (Committee on Air Force and Department of Defense Aerospace Propulsion Needs, 2006; Cecere et al., 2014; van Biert et al., 2016), Japan (Dawson, 2004; Dale Reed and Lister, 2011), Europe (Negoro, 2007), India (Sekigawa and Mecham, 1996; Chopinet et al., 2011), China (Rao et al., 2019b) and Russia (Lele, 2006) have already reported the developmental progress for hydrogen fuel technology adaptation.
The advancement of transportation in different segments and requirements has led to the development of unmanned vehicles. The most popular sector under this category is unmanned cars and unmanned Arial vehicles (UAV) (Tan, 2013). For UAV applications, various countries have focused the efficiency to cover longer distances and enhanced performance by replacing conventional fuel with clean hydrogen fuels (Sergienko, 1993; Wang et al., 2013). The development of Hydrogen mobility in different sectors are tabulated in Table 4.
It has been studied and reported that utilization of hydrogen fuel cell vehicles has been pointed in the United States and 5,899 hydrogen vehicles developed for commercialization (Bayrak et al., 2020). For the promotion of hydrogen utilized vehicles, companies like Toyota Mirai, Honda Clarity, Renault–Nissan, General Motors and Honda have formed alliances for the joint production (Giacoppo et al., 2017; Dudek et al., 2021).
4.2 Fuel Cells Electronic Vehicles
Vehicle manufacturers began producing hydrogen fuel passenger vehicles in 2002 (Tanç et al., 2018) due to an increase in the number of researchers interested in Fuel Cell Electronic Vehicles (FCEVs). They’ve been manufacturing a variety of models up to now. In addition to passenger automobiles (Lee et al., 2019; Tanç et al., 2020), these manufacturers are known to work with light commercial vehicles (Matulić et al., 2019), buses (de Miranda et al., 2017), and trucks (Lee et al., 2018). Table 5, lists all commercially available FCEVs, as well as their manufacturers and special features.
In recent years, the majority of passenger car manufacturers have started developing FCEVs. General Motors, Toyota, and Honda produce their own FC stacks, whereas Ford, Mazda, DaimlerChrysler, Mazda, Hyundai, Fiat, and Volkswagen purchase them from FC manufacturers. It is apparent from the specifications of available FCEVs that battery hybridization is currently favoured. Furthermore, automakers such as Honda, Hyundai, and Mercedes have recently developed plug-in FCEVs. Proton Exchange Membrane Fuel Cell is the most prevalent FC stack, and its efficiency for FCEVs is improving continuously. Detail specifications of the commercialized presently dominating FCEV for sale or leasing (Wasserstoffautos, 2021) are tabulated in Table 6.
For a shift from a carbon-based (fossil fuel) energy system to a hydrogen-based economy, three key technological hurdles (Chang et al., 2019) must be overcome that are as follows.
1) To compete with other options, the cost of efficient and sustainable hydrogen generation and transport must be considerably decreased.
2) In order to, offer an appropriate driving range, new generations of hydrogen storage technologies for vehicle applications must be created.
3) Fuel-cell and other hydrogen-based technologies must be less expensive while having a longer useful life.
In this context, the future market of hydrogen transportation and distribution are determined mainly by four factors (Edwards et al., 2008; Olabi et al., 2021): (a) Cost of hydrogen in future, (b) the rate of advancement of various hydrogen-based technologies, (c) restriction in GHG emission, and (d) the cost of competing for alternative transportation systems. Hydrogen has the potential to be a long-term option for sustainable mobility with several social, economic, and environmental benefits (Forsberg, 2005). It can minimize reliance/dependency on fossil fuels and reduce carbon emissions from the transportation industry in the long run.
4.3 Techno Economic Aspects
The cost and performance competitiveness of fuel cell electric vehicles (FCEV) in the car industry will determine their future. FCEV adoption in the present transportation industry is still modest. Many governments have yet to take a firm stance on hydrogen for transportation. In this regard, comprehensive energy plans for the road transportation sector are required. The use of energy systems modelling (ESM) to support energy planning is frequently advised in this context, since it provides a scientific basis for the prospective evaluation of energy systems based on technical and economic factors across time (Bhattacharyya and Timilsina, 2010). Furthermore, by using life-cycle sustainability variables in the future evaluation, ESM studies might be improved (García-Gusano et al., 2016). A variety of pathways and important conversion technologies for biomass and organic solid waste to hydrogen have been investigated (Aziz, 2021). The potential for a techno-economic and environmental assessment of the hydrogen production mix that might meet the hydrogen demand for road transport under various scenarios for FCEV penetration in Spain has been addressed (Navas-Anguita et al., 2020). Due to the reasonable costs of natural gas and the maturity of the technology, the hydrogen demand associated with the eventual penetration of FCEV in the Spanish road transport system may be totally met by conventional steam reforming of natural gas. The worldwide view on hydrogen energy systems, on the other hand, refers to a hydrogen economy based on environmentally friendly solutions. Lin et al. (Lin et al., 2013) used a cost-based consumer choice model to examine the market adoption and social advantages of FCEVs from 2015 to 2050. For the Indian urban driving cycle, Shakdwipee and Banerjee compared fuel cell cars against petrol and CNG automobiles (Manish Shakdwipee, 2006). Primary energy consumption (MJ/km), CO2 emissions (kg CO2/km), and cost (Rs./km) were used as comparison criteria. Fuel cell vehicles, they discovered, are more energy efficient and environmentally friendlier than gasoline automobiles. Fuel cell vehicles consume 43% less energy and emit 40% less CO2 than gasoline automobiles. A techno-economic analysis is conducted to assess the feasibility of deploying Fuel Cell Electric Trucks (FCET) on the Oslo-Trondheim route in Norway (Diva-Portal, 2022). The output of the infrastructure’s techno-economic model, which included various configurations and combinations of both hydrogen producing units (HPU) and hydrogen refuelling stations (HRS), was given in the form of a cost curve function based on the FCET’s fleet size. The cheapest set-up was found, consisting of a 350-bar HRS for a type 3 onboard tank with hydrogen production connected directly to it. Future cost curves for FCETs and infrastructure that indicate development in 2030 were investigated. Chen and Melaina (Chen and Melaina, 2019) established a techno-economic analysis framework to analyse the cost and performance of main vehicle technologies (internal combustion, hybrid, plug-in hybrid, battery and fuel cell electric) under various advancement scenarios for the years 2035 and 2050. Based on a 5-years or 15-years ownership term, their findings suggest that the prices per mile for FCEVs are 36% or 22% more than those of regular gasoline automobiles in the 2035 scenarios. FCEVs have 15-years ownership costs that are equivalent to gasoline automobiles with comparable engineering performance in 2050 scenarios. Fuel cell cars have a cheaper driving cost in all of the 2035 and 2050 scenarios when compared to electric vehicles with a 200-mile range. To encourage the use of hydrogen as a passenger car fuel, the cost of an FCV must be reduced to at least the same level as that of an electric vehicle.
4.4 Selected Implemented Projects, Policies and Challenges
Throughout the world many demonstration projects are implemented on hydrogen mobility. Some important implemented projects are discussed here. Han (2014) investigated the hydrogen fuel cell car demonstration projects in China, as well as their marketing methods. Their research indicated that hydrogen fuel cells are the most promising technology for reducing urban air pollution, saving energy, achieving sustainable mobility, and promoting technical change in the automobile sector. The Chinese government has adopted an ambitious strategy and is providing significant financial assistance for the development of hydrogen and related technologies. Aditiya and Aziz examined the possibility of establishing an inter-state hydrogen energy system on selected countries in the Asia-Pacific region, based on individual evaluations from the nexus of technology, social, and economic perspectives, and utilising the respective strengths to identify an inter-state hydrogen network strategy in the Asia-Pacific region, dubbed the “Asia-Pacific Hydrogen Valley” (Aditiya and Aziz, 2021). Indonesia, Malaysia, Brunei Darussalam, the Philippines, Singapore, Vietnam, Thailand, Japan, South Korea, Australia, and New Zealand are among the countries assessed. According to the findings, countries with active hydrogen policies and high R&D capacity may lead the strategy, whilst countries with high primary energy supply capacity and an economic edge would aid the group in catering energy and commercial resources, respectively. The feasibility of using hydrogen cars in various modes of transportation, including personal automobiles, taxis, and shared mobility, was investigated (Turon., 2020). Hype is the first hydrogen-powered taxi fleet in the world. The first five cars were introduced to the system on 7 December 2015 at COP 21 by Société du Taxi Electrique Parisien (“STEP”) (Hype, 2019). The fleet now consists of about 100 cars. Before the end of 2020, 600 cars are expected to be in use. The system’s taxis have a range of more than 500 km. As a result, their charging time might be as long as 5 min (Hype, 2019). In 2016, the first attempts were made to develop a car-sharing system based on hydrogen-powered vehicles. The Linde Group commenced operations at that time by launching a service under the BeeZero brand in Munich, Germany. The system has a 50-vehicle fleet. Unfortunately, the system failed to work in June 2018 after 2 years of operation (Gas World Portal, 2018). The corporation claims that economic unprofitability was the cause for its demise. Unfortunately, one of the issues that car-sharing companies face is this type of issue (Gas World Portal, 2018). This is because car-sharing is a new type of urban transportation that is now being developed among today’s communities that are accustomed to owning rather than renting a car (Turoń and Cokorilo, 2018). The introduction of hydrogen automobiles in the form of zero-emission buses is another alternative that allows the vehicle to reach the biggest number of people. A bus that uses electric energy generated by hydrogen in fuel cells or merely the engine whose cycle does not result in the production of greenhouse gases or other substances covered by the greenhouse gas emission management system (Polish Electromobility Act, 2018). An operator operating in Cologne or Wuppertal, Germany, is an example of how this type of bus may be implemented. Furthermore, this mode of transportation was so well received that a tender for the supply of a fleet of 40 cars was signed. Despite numerous dubious appraisals and public worries, primarily due to ignorance, hydrogen-powered cars appear to have a chance to becoming a viable alternative to conventional automobiles. The current condition of such vehicle use in various nations reflects a growing interest in green transportation technology and the hunt for diverse solutions that can assist transportation’s long-term growth.
Many nations have strong hydrogen support policies, and hydrogen energy will become an essential element of the future global energy plan. Japan, the European Union, the United States, and South Korea all responded enthusiastically and pushed aggressively, with national policy support focusing on hydrogen energy fuel cell cars. Foreign subsidy programmes primarily targeted the consumption connection and were paid in the form of a purchase tax credit or a purchase subsidy. The United States is the first country to use hydrogen and fuel cells as an energy source. It first proposed the notion of “hydrogen economy” in 1970, and in 1990, it passed the Hydrogen Research, Development, and Demonstration Act. The National Roadmap for Hydrogen Energy Development was announced by the US Department of Energy in November 2002, kicking off the methodical execution of the National Hydrogen Energy Plan (Wang et al., 2015). The United States of America designated October 8 as National Hydrogen and Fuel Cell Day of Remembrance in 2018. The total number of fuel-cell cars sold and leased in the United States as of 1 April 2020 was 8,285. Japan has implemented a variety of beneficial regulations aimed at speeding up the commercialization of hydrogen energy and fuel cells, with encouraging outcomes. Japan was the first country in the world to establish a comprehensive government strategy for the development of hydrogen and fuel cell technology, and the Basic Hydrogen Energy Strategy 2017 recommended that the government prepare for hydrogen and fuel cell development. Japan aims to build 320 hydrogen refuelling stations in 2025 and 900 in 2030, according to the Basic Hydrogen Energy Strategy issued in late 2017 (Wei and Chen, 2020). The Japanese government has spent hundreds of billions of yen on development and promotion of hydrogen and fuel cell technologies during the last 30 years. The EU sees hydrogen energy as a critical component of energy security and transformation. The EU Fuel Cell and Hydrogen Joint Action Plan (FCH JU) initiative offers major funding for the development and promotion of national energy and fuel cells across Europe. For the years 2014–2020, the entire budget was €665 million (European Commission, 2020). In Europe, there were 152 hydrogen refuelling stations in service by the end of 2018, with expectations to increase to 770 in 2025 and 1,500 in 2030, with roughly 1,080 fuel cell passenger cars being deployed. Since 2014, China has enacted a number of policies and measures reflecting the country’s commitment to the growth of the hydrogen and fuel cell industries, as well as the obvious trend of Chinese policies supporting the hydrogen industry’s development. According to the Ministry of Industry and Information Technology’s (MIIT) 2018 fuel cell vehicle subsidy criteria, the state provides up to 200,000, 300,000, and 500,000 yuan in subsidies for fuel cell passenger cars, medium commercial vehicles, and large commercial vehicles, respectively (Liu and Zhong, 2019). As a first step toward the National Hydrogen Mission, the Indian government announced the first phase of its Green Hydrogen Policy in 2021. The mission’s goal is to turn India into a green hydrogen centre that will assist the country reach its climate goals. It aims to produce five million metric tonnes per annum (MMTPA) of green hydrogen by 2030, as well as build renewable energy capacity in the process (Power-Technology, 2022).
Successful implementation of Hydrogen policy required extensive R&D to overcome the technical challenges to expedite the acceptance of hydrogen as future of sustainable mobility. The majority of hydrogen is now generated in a traditional manner, coming from the burning of fossil fuels, which emits a significant quantity of CO2. As a result, the primary difficulty is to create hydrogen utilising sustainable energy sources. This is a major step forward in the direction of green hydrogen. Only a few recharge stations exist across the world. Even though some governments are willing to invest in the building of hydrogen charging stations, demand remains low, and these terminals do not now make enough profit. Hydrogen produced from renewable sources is highly costly and inefficient when compared to hydrogen produced from natural gas. Furthermore, hydrogen is still exceedingly explosive. It must be kept and transported in big containers under pressure. This creates security, logistical, and financial issues that continue to obstruct its usage (Solarimpulse, 2022). According to hydrogen features and behaviour, hydrogen monitoring needs, including international partnerships and formal agreements, legislation, codes, and standards, Foorginezhad et al. (Foorginezhad, 2021) investigated the safety difficulties with hydrogen fuel cell cars. The detection performance of hydrogen sensor types relevant to fuel cell cars, such as catalytic hydrogen, electrochemical, semi-conductive metal-oxide, thermal conductivity, optical, palladium (alloy) film-based, and combination technology-based sensors, is also reviewed. Finally, future options for sensing and monitoring technologies, as well as obstacles ahead in the use of hydrogen fuel cells in automobiles as a replacement for traditional equivalents, are presented.
5 Conclusion
To reduce climate change and the adverse effect of pollutants from conventional fuel vehicles, sustainable transportation development and commercialization have evolved rapidly in the last few years. The purpose of this study is to draw attention towards the sustainable mobility and implementation of sustainable development since there is substantial potential for establishing convergence between climate change mitigation efforts and sustainable development goals in the transportation sector. Focusing on the rise in environmental concerns like greenhouse gas emissions and environmental sustainability, hydrogen energy-based technology is considered the potential for future transportation. Technical aspects presenting hydrogen generation and storage methods reveal that hydrogen is the only future fuel satisfying the criteria for sustainable mobility and designing hydrogen-based vehicles.
The review also presents exciting insights into hydrogen-based vehicles in the marine, railways and aerospace industry and concludes that hydrogen-based fuel cell vehicles should be commercialized worldwide. The review findings would also guide academia about various technical features of fuel cell electric vehicles, and they would benefit from recommending more advanced technologies for the coming future. However, the transportation and distribution of hydrogen is another significant challenge, and this is a crucial consideration while transitioning to a hydrogen economy. Investigation into hydrogen fuel vehicles and their utilization in different mobility sectors have been rigorously reviewed. Undeveloped hydrogen technologies have a high implementation cost for proper commercialization, discouraging vehicle manufacturers from adopting the technology.
Nevertheless, a potentially significant advantage in terms of zero-emission to climate has attracted the researchers for its early development and progress to enhance more widespread. Different nations’ governments must coordinate their energy requirements for the future to increase the use of hydrogen as a transportation fuel. Policy and regulatory measures and increased worldwide funding for research and commercialization initiatives would undoubtedly pave the way for taking the first steps toward a hydrogen economy, which guarantees energy security. Hydrogen holds much promise in the transportation industry if the appropriate steps and procedures are taken to make it safe, dependable, and robust.
Author Contributions
Writing—Original draft, conceptualization, analysis, visualization and data curation, SC; writing—original draft, visualization, investigation, methodology, SD; resources, supervision and data curation, RE; Writing—original draft, visualization, validation and investigation, AK; Writing—Original draft, visualization, review and editing, DE; review and editing, SM; data curation, PK; review and editing, ZS. All authors have read and agreed to the published version of the manuscript.
Conflict of Interest
Author AK was employed by the companies CPS Technologies and Steering Committee (Hydrogen Society of Australia).
The remaining authors declare that the research was conducted in the absence of any commercial or financial relationships that could be construed as a potential conflict of interest.
Publisher’s Note
All claims expressed in this article are solely those of the authors and do not necessarily represent those of their affiliated organizations, or those of the publisher, the editors and the reviewers. Any product that may be evaluated in this article, or claim that may be made by its manufacturer, is not guaranteed or endorsed by the publisher.
References
Abdalla, A. M., Hossain, S., Nisfindy, O. B., Azad, A. T., Dawood, M., and Azad, A. K. (2018). Hydrogen Production, Storage, Transportation and Key Challenges with Applications: A Review. Energy Convers. Manag. 165, 602–627. doi:10.1016/j.enconman.2018.03.088
Aditiya, H. B., and Aziz, M. (2021). Prospect of Hydrogen Energy in Asia-Pacific: A Perspective Review on Techno-Socio-Economy Nexus. Int. J. Hydrogen Energy 46 (Issue 71), 35027–35056. doi:10.1016/j.ijhydene.2021.08.070
Air Liquide Will Build the First High-pressure Hydrogen Refueling Station for Long-haul Trucks (2021). Air Liquide Will Build the First High-Pressure Hydrogen Refueling Station for Long-Haul Trucks in Europe-July 2020. Availableat: https://www.businesswire.com/news/home/20200630005806/en/Air-Liquide-Will-Build-the-First-High-pressure-Hydrogen-Refueling-Station-for-Long-haul-Trucks-in-Europe (Accessed on July 29, 2021).
Andersson, J., and Grönkvist, S. (2019). Large-scale Storage of Hydrogen. Int. J. Hydrogen Energy 44 (23), 11901–11919. doi:10.1016/j.ijhydene.2019.03.063
Apostolou, D., Enevoldsen, P., and Xydis, G. (2018). Supporting Green Urban Mobility – the Case of a Small-Scale Autonomous Hydrogen Refuelling Station. Int. J. Hydrogen Energy 44 (20), 9675–9689.
Apostolou, D. (2020). Optimization of a Hydrogen Production – Storage – Re-powering System Participating in Electricity and Transportation Markets. A Case Study for Denmark. Appl. Energy 265, 114800.
Apostolou, D., and Welcher, S. N. (2021). Prospects of the Hydrogen-Based Mobility in the Private Vehicle Market. A Social Perspective in Denmark. Int. J. Hydrogen Energy 46 (9), 6885–6900. doi:10.1016/j.ijhydene.2020.11.167
Apostolou, D., and Xydis, G. (2019). A Literature Review on Hydrogen Refuelling Stations and Infrastructure. Current Status and Future Prospects. Renew. Sustain. Energy Rev. 113, 109292. doi:10.1016/j.rser.2019.109292
Arat, H. T., and Sürer, M. G. (2017). State of Art of Hydrogen Usage as a Fuel on Aviation. Eur. Mech. Sci. 2 (1), 20–30. doi:10.26701/ems.364286
Articlelanding (2020). Articlelanding. Availableat: https://pubs.rsc.org/en/content/articlelanding/2020/se/c9se01240k.
Ates, F., and Ozcan, H. (2020). Turkey’s Industrial Waste Heat Recovery Potential with Power and Hydrogen Conversion Technologies: A Techno-Economic Analysis. Int. J. Hydrogen Energy.
Automobiles.Honda (2021). Automobiles.Honda. Available at: https://automobiles.honda.com/clarity-plug-in-hybrid (Accessed on August 28, 2021).
Aziz, M. (2021). Hydrogen Production from Biomasses and Wastes: a Technological Review. Int. J. Hydrogen Energy 46, 33756–33781.
Bayrak, Z. U., Kaya, U., and Oksuztepe, E. (2020). Investigation of PEMFC Performance for Cruising Hybrid Powered Fixed-Wing Electric UAV in Different Temperatures. Int. J. Hydrogen Energy 45 (11), 7036–7045. doi:10.1016/j.ijhydene.2019.12.214
Bethoux, O. (2020). Hydrogen Fuel Cell Road Vehicles and Their Infrastructure: An Option towards an Environmentally Friendly Energy Transition. Energies 13 (22), 6132. doi:10.3390/en13226132
Bhattacharyya, S. C., and Timilsina, G. R. (2010). A Review of Energy System Models. Int. J. Energy Sect. Manage 4, 494–518. doi:10.1108/17506221011092742
Bi, Y., Hu, H., Li, Q., and Lu, G. (2010). Efficient Generation of Hydrogen from Biomass without Carbon Monoxide at Room Temperature - Formaldehyde to Hydrogen Catalyzed by Ag Nanocrystals. Int. J. Hydrogen Energy 35 (13), 7177–7182. doi:10.1016/j.ijhydene.2009.12.142
Bicer, Y., and Dincer, I. (2017). Comparative Life Cycle Assessment of Hydrogen, Methanol and Electric Vehicles from Well to Wheel. Int. J. Hydrogen Energy 42 (6), 3767–3777. doi:10.1016/j.ijhydene.2016.07.252
Bicer, Y., and Khalid, F. (2020). Life Cycle Environmental Impact Comparison of Solid Oxide Fuel Cells Fueled by Natural Gas, Hydrogen, Ammonia and Methanol for Combined Heat and Power Generation. Int. J. Hydrogen Energy 45 (5), 3670–3685. doi:10.1016/j.ijhydene.2018.11.122
Biresselioglu, M. E., Demirbag Kaplan, M., and Yilmaz, B. K. (2018). Electric Mobility in Europe: A Comprehensive Review of Motivators and Barriers in Decision Making Processes. Transp. Res. Part A Policy Pract. 109, 1–13. doi:10.1016/j.tra.2018.01.017
Boretti, A. (2020). Production of Hydrogen for Export from Wind and Solar Energy, Natural Gas, and Coal in Australia. Int. J. Hydrogen Energy 45, 3899–3904. doi:10.1016/j.ijhydene.2019.12.080
Cao, L., Yu, I. K. M., Xiong, X., Tsang, D. C. W., Zhang, S., Clark, J. H., et al. (2020). Biorenewable Hydrogen Production through Biomass Gasification: A Review and Future Prospects. Environ. Res. 186, 109547. doi:10.1016/j.envres.2020.109547
Cecere, D., Giacomazzi, E., and Ingenito, A. (2014). A Review on Hydrogen Industrial Aerospace Applications. Int. J. Hydrogen Energy 39 (20), 10731–10747. doi:10.1016/j.ijhydene.2014.04.126
Chang, X., Ma, T., and Wu, R. (2019). Impact of Urban Development on Residents' Public Transportation Travel Energy Consumption in China: An Analysis of Hydrogen Fuel Cell Vehicles Alternatives. Int. J. Hydrogen Energy 44 (30), 16015–16027. doi:10.1016/j.ijhydene.2018.09.099
Chen, K., Laghrouche, S., and Djerdir, A. (2019). Degradation Model of Proton Exchange Membrane Fuel Cell Based on a Novel Hybrid Method. Appl. Energy 252 (113439), 113439. doi:10.1016/j.apenergy.2019.113439
Chen, Y., and Melaina, M. (2019). Model-based Techno-Economic Evaluation of Fuel Cell Vehicles Considering Technology Uncertainties. Transp. Res. Part D Transp. Environ. 74, 234–244. doi:10.1016/j.trd.2019.08.002
Chi, J., and Yu, H. (2018). Water Electrolysis Based on Renewable Energy for Hydrogen Production. Chin. J. Catal. 39 (3Mar), 390–394. doi:10.1016/s1872-2067(17)62949-8
Chng, S. (2021). Advancing Behavioural Theories in Sustainable Mobility: A Research Agenda. Urban Sci. 5 (2), 43. doi:10.3390/urbansci5020043
Chopinet, J. N., Lassoudie` re, F., Fiorentino, C., Alliot, P., Guedron, S., Supie´, P., et al. (2011). Results of the Vulcain X Technological Demonstration. 62nd Int. Astronaut. Congr. 8, 6289–6298.
Climate-Change-Atmospheric-Carbon-Dioxide (2021). Climate-Change-Atmospheric-Carbon-Dioxide. Available at: https://www.climate.gov/news-features/understanding-climate/climate-change-atmospheric-carbon-dioxidehttps://www.noaa.gov/ (Accessed on May 30, 2021).
Committee on Air Force and Department of Defense Aerospace Propulsion Needs (2006). A Review of United States Air Force and Department of Defense Aerospace Propulsion Needs. Washington, DC: The National Academies Press. 9780309102476. doi:10.17226/11780
Concawe and JRC (2007). Well-to-Wheels Analysis of Future Automotive Fuels and Power Trains in the European Context, Well-To-Wheels Report. Luxembourg: Publications Office of the European Union, 44.
Concept Consulting Group (2021). Hydrogen in New Zealand Report 2—Analysis. Availableat: http://www.concept.co.nz/uploads/2/5/5/4/25542442/h2_report2_analysis_v4.pdf (Accessed on July 28, 2021).
Cormos, C.-C. (2012). Hydrogen and Power Co-generation Based on Coal and Biomass/solid Wastes Co-gasification with Carbon Capture and Storage. Int. J. Hydrogen Energy 37 (7), 5637–5648. doi:10.1016/j.ijhydene.2011.12.132
Dale Reed, R., and Lister, D. (2011). Wingless Flight: The Lifting Body storyNASA History Series SP-4220. Washington, DC: Books Express Publishing.
Dawood, F., Anda, M., and Shafiullah, G. M. (2020). Hydrogen Production for Energy: An Overview. Int. J. Hydrogen Energy 45 (7), 3847–3869. doi:10.1016/j.ijhydene.2019.12.059
Dawson, V. P. (2004). Taming Liquid Hydrogen: The Centaur Upper Stage Rocket1958-2002. NASA-SP-2004-4230.
de Miranda, P. E. V., Carreira, E. S., Icardi, U. A., and Nunes, G. S. (2017). Brazilian Hybrid Electric-Hydrogen Fuel Cell Bus: Improved On-Board Energy Management System. Int. J. Hydrogen Energy 42 (19), 13949–13959. doi:10.1016/j.ijhydene.2016.12.155
de-Troya, J. J., Álvarez, C., Fernández-Garrido, C., and Carral, L. (2016). Analysing the Possibilities of Using Fuel Cells in Ships. Int. J. Hydrogen Energy 41 (4), 2853–2866. doi:10.1016/j.ijhydene.2015.11.145
Demirci, U. B., and Miele, P. (2009). Sodium Borohydride versus Ammonia Borane, in Hydrogen Storage and Direct Fuel Cell Applications. Energy Environ. Sci.Energy Environ. Sci. 2, 627. doi:10.1039/b900595a
Dickel, R. (2020). Blue Hydrogen As an Enabler Of Green Hydrogen: The Case Of Germany; OIES Paper. Oxford, UK: The Oxford Institute for Energy Studies.
Dincer, I. (2020). Covid‐19 Coronavirus: Closing Carbon Age, but Opening Hydrogen Age. Int. J. Energy Res. 44 (8), 6093–6097. doi:10.1002/er.5569
Ding, Z., Jiang, X., Liu, Z., Long, R., Xu, Z., and Cao, Q. (2018). Factors Affecting Low-Carbon Consumption Behavior of Urban Residents: A Comprehensive Review. Resour. Conservation Recycl. 132, 3–15. doi:10.1016/j.resconrec.2018.01.013
Diva-Portal (2022). Techno-economic Study of Hydrogen as a Heavy-Duty Truck Fuel A Case Study on the Transport, Corridor Oslo – Trondheim. Available at: https://kth.diva-portal.org/smash/get/diva2:1372698/FULLTEXT01.pdf (Accessed on 04 03, 2022).
Domashenko, A. (2002). Production, Storage and Transportation of Liquid Hydrogen. Experience of Infrastructure Development and Operation. Int. J. Hydrogen Energy 27 (7–8), 753–755. doi:10.1016/s0360-3199(01)00152-5
Dudek, M., Lis, B., Raźniak, A., Krauz, M., and Kawalec, M. (2021). Selected Aspects of Designing Modular PEMFC Stacks as Power Sources for Unmanned Aerial Vehicles. Appl. Sci. 11 (2), 675. doi:10.3390/app11020675
Eberle, U., von Helmolt, R., Stolten, P. D., Samsun, D. R. C., and Garland, D. N. (2016). “GM HydroGen4 - A Fuel Cell Electric Vehicle Based on the Chevrolet Equinox,” in Fuel Cells : Data, Facts and Figures, 75–86. doi:10.1002/9783527693924.ch08
Edwards, P. P., Kuznetsov, V. L., David, W. I. F., and Brandon, N. P. (2008). Hydrogen and Fuel Cells: Towards a Sustainable Energy Future. Energy Policy 36 (12), 4356–4362. doi:10.1016/j.enpol.2008.09.036
El-Taweel, N. A., Khani, H., and Farag, H. E. Z. (2019). Hydrogen Storage Optimal Scheduling for Fuel Supply and Capacity-Based Demand Response Program under Dynamic Hydrogen Pricing. IEEE Trans. Smart Grid 10 (4), 4531–4542. doi:10.1109/tsg.2018.2863247
Energy.Gov (2021). Energy.Gov. Available at: https://afdc.energy.gov/vehicles/fuel_cell.html (Accessed on August 29, 2021).
Engel, R. (2012). Tomorrow's Energy: Hydrogen, Fuel Cells, and the Prospects for a Cleaner Planet. Int. J. Hydrogen Energy 37 (21), 16264. doi:10.1016/j.ijhydene.2012.08.018
Environment Hydrogen-Fuel-Cell (2021). Environment Hydrogen-Fuel-Cell. Available at: https://www.hyundai.co.uk/about-us/environment/hydrogen-fuel-cell (Accessed on August 27, 2021).
European Commission (2020). European Commission on Hydrogen Energy Strategy. Paris: European Commission.
Falcone, P. M., Hiete, M., and Sapio, A. (2021). Hydrogen Economy and Sustainable Development Goals: Review and Policy Insights. Curr. Opin. Green Sustain. Chem. 31 (100506), 100506. doi:10.1016/j.cogsc.2021.100506
Ferrero, F., Perboli, G., Rosano, M., and Vesco, A. (2018). Car-sharing Services: An Annotated Review. Sustain. Cities Soc. 37, 501–518. doi:10.1016/j.scs.2017.09.020
Foorginezhad, .S. (2021). Sensing Advancement towards Safety Assessment of Hydrogen Fuel Cell Vehicles. J. Power Sources 489, 229450. doi:10.1016/j.jpowsour.2021.229450
Forsberg, C. W. (2005). Hydrogen Markets: Implications for Hydrogen Production Technologies International Journal of Hydrogen Energy (DE-AC05-00OR22725). Oak Ridge, TN, United States: Oak Ridge National Laboratory. Available at: http://www.intpowertechcorp.com/122902.pdf (Accessed on August 29, 2021).
Frenette, G., and Forthoffer, D. (2009). Economic & Commercial Viability of Hydrogen Fuel Cell Vehicles from an Automotive Manufacturer Perspective. Int. J. Hydrogen Energy 34 (9), 3578–3588. doi:10.1016/j.ijhydene.2009.02.072
Galich, A., and Marz, L. (2012). Alternative Energy Technologies as a Cultural Endeavor: a Case Study of Hydrogen and Fuel Cell Development in Germany. Energ Sustain Soc. 2 (1), 2. doi:10.1186/2192-0567-2-2
Gao, Y., Xiong, T., Li, Y., Huang, Y., Li, Y., and Balogun, M.-S. J. T. (2019). A Simple and Scalable Approach to Remarkably Boost the Overall Water Splitting Activity of Stainless Steel Electrocatalysts. ACS Omega 4 (14), 16130–16138. doi:10.1021/acsomega.9b02315
García-Gusano, D., Martín-Gamboa, M., Iribarren, D., and Dufour, J. (2016). Prospective Analysis of Life-Cycle Indicators through Endogenous Integration into a National Power Generation Model. Resources 5, 39. doi:10.3390/resources5040039
García-Melero, G., Sainz-González, R., Coto-Millán, P., and Valencia-Vásquez, A. (2021). Sustainable Mobility Policy Analysis Using Hybrid Choice Models: Is it the Right Choice? Sustainability 13 (5), 2993. doi:10.3390/su13052993
Gas World Portal (2018). Hydrogen Car-Sharing System. Available at: https://www.gasworld.com/linde-to-close-worlds-first-fuel-cell-car-sharingservice/2014327.article (Accessed on 04 04, 2021).
Giacoppo, G., Barbera, O., Briguglio, N., Cipitì, F., Ferraro, M., Brunaccini, G., et al. (2017). Thermal Study of a SOFC System Integration in a Fuselage of a Hybrid Electric Mini UAV. Int. J. Hydrogen Energy 42 (46), 28022–28033. doi:10.1016/j.ijhydene.2017.09.063
Global-Energy-Related-Co2-Emissions (2021). Global-Energy-Related-Co2-Emissions. Availableat: https://www.iea.org/data-and-statistics/charts/global-energy-related-co2-emissions-by-sector (Accessed on June 15, 2021).
Global-Greenhouse-Gas-Emissions-Data (2021). Global-Greenhouse-Gas-Emissions-Data. Availableat: https://www.epa.gov/ghgemissions/global-greenhouse-gas-emissions-data (accessed on May 25, 2021).
Global.Honda (2021). Global.Honda. Available at: https://global.honda/heritage/timeline/product-history/automobiles/2001FCX-V4.html (accessed on August 28, 2021).
Gonzalez Aregall, M., Bergqvist, R., and Monios, J. (2018). A Global Review of the Hinterland Dimension of Green Port Strategies. Transp. Res. Part D Transp. Environ. 59, 23–34. doi:10.1016/j.trd.2017.12.013
Graetz, J. (2012). Metastable Metal Hydrides for Hydrogen Storage. ISRN Mater. Sci. 8. doi:10.5402/2012/863025
Han, W. (2014). Demonstrations and Marketing Strategies of Hydrogen Fuel Cell Vehicles in China. Int. J. Hydrogen Energy 39, 13859–13872.
Health-Topics (2021). Health-Topics. Availableat: https://www.who.int/health-topics/air-pollution#tab=tab_1 (Accessed on June 4, 2021).
Holden, E., Gilpin, G., and Banister, D. (2019). Sustainable Mobility at Thirty. Sustainability 11 (7), 1965.
Hu, Y., Huang, D., Zhang, J., Huang, Y., Balogun, M. S. J. T., and Tong, Y. (2019). Dual Doping Induced Interfacial Engineering of Fe 2 N/Fe 3 N Hybrids with Favorable d‐Band towards Efficient Overall Water Splitting. ChemCatChem 11 (24), 6051–6060. doi:10.1002/cctc.201901224
Huang, Y., Hu, L., Liu, R., Hu, Y., Xiong, T., Qiu, W., et al. (2019). Nitrogen Treatment Generates Tunable Nanohybridization of Ni5P4 Nanosheets with Nickel Hydr(oxy)oxides for Efficient Hydrogen Production in Alkaline, Seawater and Acidic Media. Appl. Catal. B Environ. 251 (Aug), 181–194. doi:10.1016/j.apcatb.2019.03.037
Huang, Z., Shen, J., Chan, S. H., and Tu, Z. (2020). Transient Response of Performance in a Proton Exchange Membrane Fuel Cell under Dynamic Loading. Energy Convers. Manag. 226 (113492), 113492. doi:10.1016/j.enconman.2020.113492
Hui, J., Xiao, Z., Liejin, G., Chao, Z., Changqing, C., and Zhenqun, W. (2017). Experimental Investigation on Methanation Reaction Based on Coal Gasification in Supercritical Water. Int. J. Hydrogen Energy 42 (7), 4636–4641. doi:10.1016/j.ijhydene.2016.06.216
Hydrogen Storage (2021). Hydrogen and Fuel Cell Technologies Office. Availableat: https://www.energy.gov/e ere/fuecells/ hydro gen-storage (Accessed on August 20, 2021).
Hydrogen-Production-By-Electrolysis-Ann-Cornell (2017). Hydrogen-Production-By-Electrolysis-Ann-Cornell. Availableat: https://energiforskmedia.blob.core.windows.net/media/23562/5-hydrogen-production-by-electrolysis-ann-cornell-kth.pdf.
Hydrogen-Production-Through-Electrolysis (1927). Hydrogen-Production-Through-Electrolysis. Availableat: https://www.h2bulletin.com/knowledge/hydrogen-production-through-electrolysis/.
Hydrogen-Production-Through-Electrolysis (2017). Progress and Prospects of Hydrogen Production: Opportunities and Challenges. Availableat: https://www.h2bulletin.com/knowledge/hydrogen-production-through-electrolysis/.
Hydrogencarsnow (2021). Hydrogencarsnow. Available at: https://www.hydrogencarsnow.com/index.php/ford-focus-fcv/ (Accessed on August 28, 2021).
Hype (2019). Hydrogen Taxi Operator. available at: https://hype.taxi/ (accessed on 04 04, 2022).
Hyundai (2021). Hyundai. Availableat: https://www.hyundai.com/worldwide/en/eco/nexo/technology (Accessed on August 25, 2021).
Ijaodola, O. S., El- Hassan, Z., Ogungbemi, E., Khatib, F. N., Wilberforce, T., Thompson, J., et al. (2019). Energy Efficiency Improvements by Investigating the Water Flooding Management on Proton Exchange Membrane Fuel Cell (PEMFC). Energy 179, 246–267. doi:10.1016/j.energy.2019.04.074
IMO (2012b). Guidelines for the Development of a Ship Energy Efficiency Management Plan (SEEMP); Resolution MEPC.213. London, UK: IMO.
IMO (2012a). Guidelines on the Method of Calculation of the Attained Energy Efficiency Design Index (EEDI) for New Ships; Resolution MEPC.212. London, UK: IMO.
Ivanenko, A. (2020). A Look at the Colors of Hydrogen that Could Power Our Future. Forbes. Availableat: https://www.forbes.com/sites/forbestechcouncil/2020/08/31/a-look-at-the-colors-of-hydrogen-that-could-power-our-future/?sh=3edf9d6e5e91 (accessed on December 30, 2020).
Jhunjhunwala, A., Kaur, P., and Mutagekar, S. (2018). Electric Vehicles in India: A Novel Approach to Scale Electrification. IEEE Electrific. Mag. 6 (4), 40–47. doi:10.1109/mele.2018.2871278
Jiang, W., Jiao, X., and Chen, D. (2013). Photocatalytic Water Splitting of Surfactant-free Fabricated High Surface Area NaTaO3 Nanocrystals. Int. J. Hydrogen Energy 38 (29), 12739–12746. doi:10.1016/j.ijhydene.2013.07.072
Jovan, D. J., and Dolanc, G. (2020). Can Green Hydrogen Production Be Economically Viable under Current Market Conditions. Energies 13, 6599. doi:10.3390/en13246599
Kandidayeni, M., Macias, A., Khalatbarisoltani, A., Boulon, L., and Kelouwani, S. (2019). Benchmark of Proton Exchange Membrane Fuel Cell Parameters Extraction with Metaheuristic Optimization Algorithms. Energy 183, 912–925. doi:10.1016/j.energy.2019.06.152
Kaur, A., Gangacharyulu, D., and Bajpai, P. K. (2016). Catalytic Hydrogen Generation from NaBH4/H2O System: Effects of Catalyst and Promoters. Braz. J. Chem. Eng. 35, 131.
Kaur, A., Gangacharyulu, d., and Bajpai, p. K. (2019). Kinetic Studies of Hydrolysis Reaction of Nabh4 with Γ-Al2o3 Nanoparticles as Catalyst Promoter and Cocl2 as Catalyst. Braz. J. Chem. Eng. 36, 929–939. doi:10.1590/0104-6632.20190362s20180290
Klanchar, M., Hughes, T. G., and Gruber, P. (2004). “Attaining DOE Hydrogen Storage Goals with Chemical Hydrides,” in 15th Hydrogen Annual Conference (Washington D. C: National Hydrogen Association).
Klecha, L., and Gianni, F. (2018). “Designing for Sustainable Urban Mobility Behaviour: A Systematic Review of the Literature,” in Citizen, Territory and Technologies: Smart Learning Contexts and Practices (Cham: Springer International Publishing), 137–149. doi:10.1007/978-3-319-61322-2_14
Korpas, M., and Gjengedal, T. (2006). “Opportunities for Hydrogen Storage in Connection with Stochastic Distributed Generation,” in 2006 International Conference on Probabilistic Methods Applied to Power Systems. doi:10.1109/pmaps.2006.360255
Kumar, R. R., and Alok, K. (2020). Adoption of Electric Vehicle: A Literature Review and Prospects for Sustainability. J. Clean. Prod. 253, 119911.
Le Quéré, C., Jackson, R. B., Jones, M. W., Smith, A. J. P., Abernethy, S., Andrew, R. M., et al. (2020). Temporary Reduction in Daily Global CO2 Emissions during the COVID-19 Forced Confinement. Nat. Clim. Chang. 10 (7), 647–653. doi:10.1038/s41558-020-0797-x
Lee, D.-Y., Elgowainy, A., Kotz, A., Vijayagopal, R., and Marcinkoski, J. (2018). Life-cycle Implications of Hydrogen Fuel Cell Electric Vehicle Technology for Medium- and Heavy-Duty Trucks. J. Power Sources 393, 217–229. doi:10.1016/j.jpowsour.2018.05.012
Lee, D.-Y., Elgowainy, A., and Vijayagopal, R. (2019). Well-to-wheel Environmental Implications of Fuel Economy Targets for Hydrogen Fuel Cell Electric Buses in the United States. Energy Policy 128, 565–583. doi:10.1016/j.enpol.2019.01.021
Lele, A. (2006). GSLV-D5 Success: A Major Booster to India’s Space Program. The space review. Available at: https://www.thespacereview.com/article/2428/1
Leo, T. J., Durango, J. A., and Navarro, E. (2010). Exergy Analysis of PEM Fuel Cells for Marine Applications. Energy 35 (2), 1164–1171. doi:10.1016/j.energy.2009.06.010
Letnik, T., Marksel, M., Luppino, G., Bardi, A., and Božičnik, S. (2018). Review of Policies and Measures for Sustainable and Energy Efficient Urban Transport. Energy 163, 245–257. doi:10.1016/j.energy.2018.08.096
Li, Q., Yang, H., Han, Y., Li, M., and Chen, W. (2016). A State Machine Strategy Based on Droop Control for an Energy Management System of PEMFC-Battery-Supercapacitor Hybrid Tramway. Int. J. Hydrogen Energy 41 (36), 16148–16159. doi:10.1016/j.ijhydene.2016.04.254
Li, Y., Guo, L., Zhang, X., Jin, H., and Lu, Y. (2010). Hydrogen Production from Coal Gasification in Supercritical Water with a Continuous Flowing System. Int. J. Hydrogen Energy 35 (7), 3036–3045. doi:10.1016/j.ijhydene.2009.07.023
Li, Y., Pei, P., Ma, Z., Ren, P., and Huang, H. (2020). Analysis of Air Compression, Progress of Compressor and Control for Optimal Energy Efficiency in Proton Exchange Membrane Fuel Cell. Renew. Sustain. Energy Rev. 133 (110304), 110304. doi:10.1016/j.rser.2020.110304
Lin, Z., Dong, J., and Greene, D. L. (2013). Hydrogen Vehicles: Impacts of DOE Technical Targets on Market Acceptance and Societal Benefits. Int. J. Hydrogen Energy 38 (19), 7973–7985. doi:10.1016/j.ijhydene.2013.04.120
Liu, J., and Zhong, F. (2019). Current Situation and Prospect of Hydrogen Energy Development in China. China Energy 41 (02), 32–36.
Liu, Y., Liu, R., and Jiang, X. (2019). What Drives Low-Carbon Consumption Behavior of Chinese College Students? the Regulation of Situational Factors. Nat. Hazards (Dordr.) 95 (1–2), 173–191. doi:10.1007/s11069-018-3497-3
López, C., Ruíz-Benítez, R., and Vargas-Machuca, C. (2019). On the Environmental and Social Sustainability of Technological Innovations in Urban Bus Transport: The EU Case. Sustainability 11 (5), 1413. doi:10.3390/su11051413
Lototskyy, M. V., Tolj, I., Pickering, L., Sita, C., Barbir, F., and Yartys, V. (2017). The Use of Metal Hydrides in Fuel Cell Applications. Prog. Nat. Sci. Mater. Int. 27 (1), 3–20. doi:10.1016/j.pnsc.2017.01.008
Low, J., Haszeldine, R. S., and Mouli-Castillo, J. (2020). Comparative Evaluation of Battery Electric and Hydrogen Fuel Cell Electric Vehicles for Zero Carbon Emissions Road Vehicle Fuel in Scotland. engrXiv.
Luo, Z., Hu, Y., Xu, H., Gao, D., and Li, W. (2020). Cost-Economic Analysis of Hydrogen for China's Fuel Cell Transportation Field. Energies 13, 6522. doi:10.3390/en13246522
Macher, J., Hausberger, A., Macher, A. E., Morak, M., and Schrittesser, B. (2021). Critical Review of Models for H2-Permeation through Polymers with Focus on the Differential Pressure Method. Int. J. Hydrogen Energy 46 (43), 22574–22590. doi:10.1016/j.ijhydene.2021.04.095
Manish Shakdwipee, R. B. (2006). Techno-economic Assessment of Fuel Cell Vehicles for India WHEC 16 – Lyon France June, 13–16.
Manna, J., Prakash, J., Sarkhel, R., Banerjee, C., Tripathi, A. K., and Nouni, M. R. (2021). Opportunities for Green Hydrogen Production in Petroleum Refining and Ammonia Synthesis Industries in India. Int. J. Hydrogen Energy. doi:10.1016/j.ijhydene.2021.09.064
Mari, V., Kristin, J., and Rahul, A. (2016). Hydrogen Production with CO2 Capture. Int. J. Hydrog. Energy 41, 4969–4992.
Marrero-Alfonso, E. Y., Beaird, A. M., Davis, T. A., and Matthews, M. A. (2009). Hydrogen Generation from Chemical Hydrides. Ind. Eng. Chem. Res. 48, 3703–3712. doi:10.1021/ie8016225
Martínez-Díaz, M., Soriguera, F., and Pérez, I. (2019). Autonomous Driving: a Bird’s Eye View. IET Intell. Transp. Syst. 13 (4), 563–579.
Matsunaga, M., Fukushima, T., and Ojima, K. (2009). Powertrain System of Honda FCX Clarity Fuel Cell Vehicle. Wevj 3 (4), 820–829. doi:10.3390/wevj3040820
Matulić, N., Radica, G., Barbir, F., and Nižetić, S. (2019). Commercial Vehicle Auxiliary Loads Powered by PEM Fuel Cell. Int. J. Hydrogen Energy 44 (20), 10082–10090.
Mayrhofer, M., Koller, M., Seemann, P., Prieler, R., and Hochenauer, C. (2021). Assessment of Natural Gas/hydrogen Blends as an Alternative Fuel for Industrial Heat Treatment Furnaces. Int. J. Hydrogen Energy 46 (41), 21672–21686. doi:10.1016/j.ijhydene.2021.03.228
Mayyas, A. R., Ramani, D., Kannan, A. M., Hsu, K., Mayyas, A., and Schwenn, T. (2014). Cooling Strategy for Effective Automotive Power Trains: 3D Thermal Modeling and Multi-Faceted Approach for Integrating Thermoelectric Modules into Proton Exchange Membrane Fuel Cell Stack. Int. J. Hydrogen Energy 39 (30), 17327–17335. doi:10.1016/j.ijhydene.2014.08.034
Mbie, A. (2019). Vision for Hydrogen in New Zealand. Energy Strategies for New Zealand. Availableat: https://www.mbie.govt.nz/building-and-energy/energy-and-natural-resources/energy-strategies-for-new-zealand/ (Accessed on August 19, 2021).
Meng, X., Gu, A., Wu, X., Zhou, L., Zhou, J., Liu, B., et al. (2021). Status Quo of China Hydrogen Strategy in the Field of Transportation and International Comparisons. Int. J. Hydrogen Energy 46 (57), 28887–28899. doi:10.1016/j.ijhydene.2020.11.049
Meraj, S. T., Yahaya, N. Z., Singh, B. S. M., and Kannan, R. (2020). Implementation of A Robust Hydrogen-Based Grid System to Enhance Power Quality. Int. Conf. Power Energy Conf., 153–158. doi:10.1109/pecon48942.2020.9314536
Mercedes-Benz (2021). Mercedes-benz. Availableat: https://www.mercedes-benz.com/en/vehicles/passenger-cars/glc/the-new-glc-f-cell/ (Accessed on August 28, 2021).
Mercedes-Benz-Cars (2021). Mercedes-Benz-Cars. Availableat: https://www.mercedes-benz.co.in/passengercars/mercedes-benz-cars/models/a-class/sedan-v177/explore.html (Accessed on August 28, 2021).
Morel, J., Obara, S., Sato, K., Mikawa, D., Watanabe, H., and Tanaka, T. (2015). “Contribution of a Hydrogen Storage-Transportation System to the Frequency Regulation of a Microgrid,” in 2015 International Conference on Renewable Energy Research and Applications (Palermo: ICRERA). doi:10.1109/icrera.2015.7418465
Muir, S. S., and Yao, X. (2011). Progress in Sodium Borohydride as a Hydrogen Storage Material: Development of Hydrolysis Catalysts and Reaction Systems. Int. J. Hydrogen Energy 36, 5983–5997. doi:10.1016/j.ijhydene.2011.02.032
Navas-Anguita, Z., García-Gusano, D., and Dufour, J. (2020). Diego Iribarren, Prospective Techno-Economic and Environmental Assessment of a National Hydrogen Production Mix for Road Transport. Appl. Energy 259, 114121. doi:10.1016/j.apenergy.2019.114121
Negoro, N. (2007). “Next Booster Engine LE-X in japan,” in 43rd AIAA/ASME/SAE/ASEE Joint Propulsion (Conference & Exhibit), Cincinnati, OH. doi:10.2514/6.2007-5490
Nissan-Global (2021). Nissan-Global. Availableat: https://www.nissan-global.com/EN/TECHNOLOGY/OVERVIEW/fcv.html (Accessed on August 28, 2021).
Noussan, M., Raimondi, P. P., Scita, R., and Hafner, M. (2021). The Role of Green and Blue Hydrogen in the Energy Transition—A Technological and Geopolitical Perspective. Sustainability 13, 298. doi:10.3390/su13010298
Ogungbemi, E., Wilberforce, T., Ijaodola, O., Thompson, J., and Olabi, A. G. (2021). Selection of Proton Exchange Membrane Fuel Cell for Transportation. Int. J. Hydrogen Energy 46 (59), 30625–30640. doi:10.1016/j.ijhydene.2020.06.147
Okolie, J. A., Patra, B. R., Mukherjee, A., Nanda, S., Dalai, A. K., and Kozinski, J. A. (2021). Futuristic Applications of Hydrogen in Energy, Biorefining, Aerospace, Pharmaceuticals and Metallurgy. Int. J. Hydrogen Energy 46 (13), 8885–8905. doi:10.1016/j.ijhydene.2021.01.014
Olabi, A. G., Wilberforce, T., and Abdelkareem, M. A. (2021). Fuel Cell Application in the Automotive Industry and Future Perspective. Energy 214, 118955.
Oliveira, G. D., and Dias, L. C. (2020). The Potential Learning Effect of a MCDA Approach on Consumer Preferences for Alternative Fuel Vehicles. Ann. Oper. Res. 293 (2), 767–787. doi:10.1007/s10479-020-03584-x
Özcan, A., and Garip, M. T. (2020). Development of a Simple and Efficient Method to Prepare a Platinum-Loaded Carbon Electrode for Methanol Electrooxidation. Int. J. Hydrogen Energy 45 (35), 17858–17868. doi:10.1016/j.ijhydene.2020.04.230
Perdikaris, N., Panopoulos, K. D., Hofmann, P., Spyrakis, S., and Kakaras, E. (2010). Design and Exergetic Analysis of a Novel Carbon Free Tri-generation System for Hydrogen, Power and Heat Production from Natural Gas, Based on Combined Solid Oxide Fuel and Electrolyser Cells. Int. J. Hydrogen Energy 35 (6), 2446–2456. doi:10.1016/j.ijhydene.2009.07.084
Perez, R. J., Brent, A. C., and Hinkley, J. (2021). Assessment of the Potential for Green Hydrogen Fuelling of Very Heavy Vehicles in New Zealand. Energies 14 (9), 2636. doi:10.3390/en14092636
Pojani, D., and Stead, D. (2018). Policy Design for Sustainable Urban Transport in the Global South. Policy Des. Pract. 1 (2), 90–102. doi:10.1080/25741292.2018.1454291
Polish Electromobility Act (2018). Polish Electromobility Act. Available at: http://prawo.sejm.gov.pl/isap.nsf/download.xsp/WDU20180000317/T/D20180317L.pdf (Accessed on 04 04, 2022).
Power-Technology (2022). Power-Technology. Available at: https://www.power-technology.com/comment/green-hydrogen-india-energy-security/ (Accessed on 04 05, 2022).
Ren, P., Pei, P., Li, Y., Wu, Z., Chen, D., and Huang, S. (2020). Degradation Mechanisms of Proton Exchange Membrane Fuel Cell under Typical Automotive Operating Conditions, Prog. Energy Combust. Sci. 80, 100859.
Rabiee, A., Keane, A., and Soroudi, A. (2021). Green Hydrogen: A New Flexibility Source for Security Constrained Scheduling of Power Systems with Renewable Energies. Int. J. Hydrogen Energy 46. doi:10.1016/j.ijhydene.2021.03.080
Ranieri, L., Digiesi, S., Silvestri, B., and Roccotelli, M. (2018). A Review of Last Mile Logistics Innovations in an Externalities Cost Reduction Vision. Sustainability 10 (3), 782. doi:10.3390/su10030782
Ransformative-Mobility (2021). Ransformative-Mobility. Availableat: https://www.transformative-mobility.org/publications/benefits-of-sustainable-mobility (Accessed on August 15, 2021).
Rao, M. K., Sridhara Murthi, K. R., and Prasad, M. Y. S. (2019). The Decision for Indian Human Spaceflight Programme-Political Perspectives, National Relevance, and Technological Challenges. New Space 7 (2), 99–109. doi:10.1089/space.2018.0028
Rao, Y., Shao, Z., Ahangarnejad, A. H., Gholamalizadeh, E., and Sobhani, B. (2019). Shark Smell Optimizer Applied to Identify the Optimal Parameters of the Proton Exchange Membrane Fuel Cell Model. Energy Convers. Manag. 182, 1–8. doi:10.1016/j.enconman.2018.12.057
Reddy, S. N., Nanda, S., Vo, D.-V. N., Nguyen, T. D., Nguyen, V.-H., Abdullah, B., et al. (2020). “Hydrogen: Fuel of the Near Future,” in New Dimensions in Production and Utilization of Hydrogen (Elsevier), 1–20. doi:10.1016/b978-0-12-819553-6.00001-5
Ren, R., Hu, W., Dong, J., Sun, B., Chen, Y., and Chen, Z. (2019). A Systematic Literature Review of Green and Sustainable Logistics: Bibliometric Analysis, Research Trend and Knowledge Taxonomy. Ijerph 17 (1), 261. doi:10.3390/ijerph17010261
Roadmap to a Single European Transport Area-Towards a competitive and resource efficient transport system (2021). Roadmap to a Single European Transport Area-Towards a Competitive and Resource Efficient Transport System. Available online: http://eur-lex.europa.eu/legal-content/EN/TXT/?uri=URISERV:tr0054 (Accessed on June 20, 2021).
Rohith, P. K., Priolkar, J., and Kunkolienkar, G. R. (2016). “Hydrogen: An Innovative and Alternative Energy for the Future,” in 2016 World Conference on Futuristic Trends in Research and Innovation for Social Welfare(Coimbatore: Startup Conclave). doi:10.1109/startup.2016.7583905
Rolandgumpert (2021). Rolandgumpert. Available at: https://www.rolandgumpert.com/en/ (Accessed on August 28, 2021).
Sakintuna, B., Lamaridarkrim, F., and Hirscher, M. (2007). Metal Hydride Materials for Solid Hydrogen Storage: A Review☆. Int. J. Hydrogen Energy 32, 1121–1140. doi:10.1016/j.ijhydene.2006.11.022
Saleem, M. A., Eagle, L., and Low, D. (2021). Determinants of Eco-Socially Conscious Consumer Behavior toward Alternative Fuel Vehicles. Jcm 38 (2), 211–228. doi:10.1108/jcm-05-2019-3208
Santos, G. (2018). Sustainability and Shared Mobility Models. Sustainability 10 (9), 3194. doi:10.3390/su10093194
Sapru, K. (2002). “Development of a Small Scale Hydrogen Production-Storage System of Hydrogen Applications,” in IECEC-97 Proceedings of the Thirty-Second Intersociety Energy Conversion Engineering Conference (Cat (No.97CH6203), Honolulu, HI.
Sattler, G. (2000). Fuel Cells Going Onboard. J. Power Sources 86 (1–2), 61–67. doi:10.1016/s0378-7753(99)00414-0
Schiro, F., Stoppato, A., and Benato, A. (2020). Modelling and Analyzing the Impact of Hydrogen Enriched Natural Gas on Domestic Gas Boilers in a Decarbonization Perspective. Carbon Resour. Convers. 3, 122–129. doi:10.1016/j.crcon.2020.08.001
Sekigawa, E., and Mecham, M. (1996). Mitsubishi Advances LE-5 Design as H2 Goes Commercial. Aviat. Week Space Technol. 145, 3.
Sergeant., N., Boureima., F.-S., Matheys., J., Timmermans., J.-M., and Van Mierlo., J. (2009). An Environmental Analysis of FCEV and H2-ICE Vehicles Using the Ecoscore Methodology. World Electr. Veh. J. 3 (3), 635–646.
Sergienko, A. (1993). Liquid Rocket Engines for Large Thrust: Present and Future. Acta Astronaut. 29 (12), 905–909. doi:10.1016/0094-5765(93)90011-k
Shang, Y., and Chen, R. (2006). Semiempirical Hydrogen Generation Model Using Concentrated Sodium Borohydride Solution. Energy fuels. 20, 2149–2154. doi:10.1021/ef050380f
Shusheng, X., Qiujie, S., Baosheng, G., Encong, Z., and Zhankuan, W. (2020). Research and Development of On-Board Hydrogen-Producing Fuel Cell Vehicles. Int. J. Hydrogen Energy 45 (35), 17844–17857. doi:10.1016/j.ijhydene.2020.04.236
Solarimpulse (2022). Solarimpulse. Availableat: https://solarimpulse.com/hydrogen-mobility-solutions (Accessed on 04 05, 2022).
Staffell, I., Scamman, D., Velazquez Abad, A., Balcombe, P., Dodds, P. E., Ekins, P., et al. (2019). The Role of Hydrogen and Fuel Cells in the Global Energy System. Energy Environ. Sci. 12 (2), 463–491. doi:10.1039/c8ee01157e
Sum4all.org (2021). Sum4all.org. Availableat: https://www.sum4all.org/implementing-sdgs (Accessed on July 28, 2021).
Sun, J., Feng, H., Xu, J., Jin, H., and Guo, L. (2021). Investigation of the Conversion Mechanism for Hydrogen Production by Coal Gasification in Supercritical Water. Int. J. Hydrogen Energy 46 (17), 10205–10215. doi:10.1016/j.ijhydene.2020.12.130
Taiebat, M., Brown, A. L., Safford, H. R., Qu, S., and Xu, M. (2018). A Review on Energy, Environmental, and Sustainability Implications of Connected and Automated Vehicles. Environ. Sci. Technol. 52 (20), 11449–11465. doi:10.1021/acs.est.8b00127
Tan, Y. H. (2013). Research on Large Thrust Liquid Rocket Engine. Yuhang Xuebao/J Astronaut. 34, 1303–1308.
Tanç, B., Arat, H. T., Baltacıoğlu, E., and Aydın, K. (2018). Overview of the Next Quarter Century Vision of Hydrogen Fuel Cell Electric Vehicles. Int. J. Hydrogen Energy 44 (20), 10120.
Tanç, B., Arat, H. T., Conker, Ç., Baltacioğlu, E., and Aydin, K. (2020). Energy Distribution Analyses of an Additional Traction Battery on Hydrogen Fuel Cell Hybrid Electric Vehicle. Int. J. Hydrogen Energy 45 (49), 26344–26356. doi:10.1016/j.ijhydene.2019.09.241
Tasleem, S., and Tahir, M. (2020). Current Trends in Strategies to Improve Photocatalytic Performance of Perovskites Materials for Solar to Hydrogen Production. Renew. Sustain. Energy Rev. 132 (110073), 110073. doi:10.1016/j.rser.2020.110073
Tirachini, A. (2020). Ride-hailing, Travel Behaviour and Sustainable Mobility: an International Review. Transportation 47 (4), 2011–2047. doi:10.1007/s11116-019-10070-2
Topler, J. (2017). “Hydrogen Technology and Economy in Germany-History and Present State,” in Hydrogen in an International Context: Vulnerabilities of Hydrogen Energy in Emerging Markets. Editor I. Lordache (Gistrup, Denmark; Delft, Netherlands: River Publishers), 3–48.
Traction: India to trial fuel cell trainset (2021). Traction: India to Trial Fuel Cell Trainset. Available online: https://www.railwaygazette.com/in-depth/traction-india-to-trial-fuel-cell- trainset/57957.article#:∼:text=India's%20hydrogen%20prototype,by%20the%20end%20of%202021 (Accessed on July 2, 2021).
Trencher, G., and Edianto, A. (2021). Drivers and Barriers to the Adoption of Fuel Cell Passenger Vehicles and Buses in Germany. Energies 14 (4), 833. doi:10.3390/en14040833
Tronstad, T., Åstrand, H. H., Haugom, G. P., and Langfeldt, L. (2017). Study on the Use of Fuel Cells in Shipping. Oslo, Norway. Report to European Maritime Safety Agency by DNV GL; DNV GL.
Turoń, K. (2018). “Car-sharing Problems - Multi-Criteria Overview,” in International Conference on Traffic and Transport Engineering. Editor O. Cokorilo (Belgrade Serbia: City Net Scientific Research Center), 916–922. ICTTE, September 27-28th, 2018, Belgrade, Serbia.
Turon., K. (2020). Hydrogen-powered Vehicles in Urban Transport Systems – Current State and Development. Transp. Res. Procedia 45, 835–841. doi:10.1016/j.trpro.2020.02.086,
van Biert, L., Godjevac, M., Visser, K., and Aravind, P. V. (2016). A Review of Fuel Cell Systems for Maritime Applications. J. Power Sources 327, 345–364. doi:10.1016/j.jpowsour.2016.07.007
Van Mierlo, J., Timmermans, J.-M., Maggetto, G., Van den Bossche, P., Meyer, S., Hecq, W., et al. (2004). Environmental Rating of Vehicles with Different Alternative Fuels and Drive Trains: a Comparison of Two Approaches. Transp. Res. Part D Transp. Environ. 9 (5), 387–399. doi:10.1016/j.trd.2004.08.005
Vialetto, G., Noro, M., Colbertaldo, P., and Rokni, M. (2019). Enhancement of Energy Generation Efficiency in Industrial Facilities by SOFC - SOEC Systems with Additional Hydrogen Production. Int. J. Hydrogen Energy 44 (19), 9608–9620. doi:10.1016/j.ijhydene.2018.08.145
Vincent, I., and Bessarabov, D. (2018). Low Cost Hydrogen Production by Anion Exchange Membrane Electrolysis: a Review. Renew. Sustain. Energy Rev. 81 (Jan), 1690–1704. doi:10.1016/j.rser.2017.05.258
von Döllen, A., Hwang, Y., and Schlüter, S. (2021). The Future Is Colorful-An Analysis of the CO2 Bow Wave and Why Green Hydrogen Cannot Do it Alone. Energies 14, 5720. doi:10.3390/en14185720
Wang, H., Wang, Z., Sun, M., and Wu, H. (2013). Combustion Modes of Hydrogen Jet Combustion in a Cavity-Based Supersonic Combustor. Int. J. Hydrogen Energy 38 (27), 12078–12089.
Wang, Q., Xue, M., Lin, B.-L., Lei, Z., and Zhang, Z. (2020). Well-to-wheel Analysis of Energy Consumption, Greenhouse Gas and Air Pollutants Emissions of Hydrogen Fuel Cell Vehicle in China. J. Clean. Prod. 275 (123061), 123061. doi:10.1016/j.jclepro.2020.123061
Wang, Y., Gao, L., and Liu, Y. (2015). America's National Hydrogen Energy Program and its Enlightenment. U.S. Department of Energy, Office of Science, Office of Basic Energy Sciences, 22–29.
Wasserstoffautos (2021). Wasserstoffautos. Available at: https://h2.live/en/wasserstoffautos/ (Accessed on August 29, 2021).
Wei, W., and Chen, W. (2020). Development Strategy and Enlightenment of Hydrogen Energy in Japan. Globalization, 60–71+135.
Weinmann, O. (1999). Hydrogen - the Flexible Storage for Electrical Energy. Power Eng. J. 13 (3), 164–170. doi:10.1049/pe:19990311
World Commission on Environment and Development (1987). Our Common Future. Oxford, UK: Oxford University Press.
World's First Hydrogen Train Runs Route in Germany (2021). World's First Hydrogen Train Runs Route in Germany-Report 2020. Availableat: https://www.industryweek.com/technology-and-iiot/emerging-technologies/article/22026353/worlds-first-hydrogen-train-runs-route-in-germany (Accessed on August 5, 2021).
Yan, L., Zhang, J., Zhou, X., Wu, X., Lan, J., Wang, Y., et al. (2013). Crystalline Phase-dependent Photocatalytic Water Splitting for Hydrogen Generation on KNbO3 Submicro-Crystals. Int. J. Hydrogen Energy 38 (9), 3554–3561. doi:10.1016/j.ijhydene.2013.01.028
Yang, C., and Ogden, J. (2007). Determining the Lowest-Cost Hydrogen Delivery Mode. Int. J. Hydrogen Energy 32 (2), 268–286. doi:10.1016/j.ijhydene.2006.05.009
Zhang, G., Xie, X., Xuan, J., Jiao, K., and Wang, Y. (2019). “Three-dimensional Multi-Scale Simulation for Large-Scale Proton Exchange Membrane Fuel Cell,” in SAE Technical Paper Series. doi:10.4271/2019-01-0381
Zhang, G., Yuan, H., Wang, Y., and Jiao, K. (2019). Three-dimensional Simulation of a New Cooling Strategy for Proton Exchange Membrane Fuel Cell Stack Using a Non-isothermal Multiphase Model. Appl. Energy 255 (113865), 113865. doi:10.1016/j.apenergy.2019.113865
Zhang, T., Zhao, K., Yu, J., Jin, J., Qi, Y., Li, H., et al. (2013). Photocatalytic Water Splitting for Hydrogen Generation on Cubic, Orthorhombic, and Tetragonal KNbO3 Microcubes. Nanoscale 5 (18), 8375–8383. doi:10.1039/c3nr02356g
Zhao, F., Mu, Z., Hao, H., Liu, Z., He, X., Victor Przesmitzki, S., et al. (2020). Hydrogen Fuel Cell Vehicle Development in China: An Industry Chain Perspective. Energy Technol. 8 (11), 2000179. doi:10.1002/ente.202000179
Keywords: climate change, sustainable mobility, hydrogen mobility, hydrogen fuel, GHG
Citation: Chakraborty S, Dash SK, Elavarasan RM, Kaur A, Elangovan D, Meraj ST, Kasinathan P and Said Z (2022) Hydrogen Energy as Future of Sustainable Mobility. Front. Energy Res. 10:893475. doi: 10.3389/fenrg.2022.893475
Received: 10 March 2022; Accepted: 19 April 2022;
Published: 31 May 2022.
Edited by:
Subrata Hait, Indian Institute of Technology Patna, IndiaReviewed by:
Muhammad Aziz, The University of Tokyo, JapanSushant Kumar, Indian Institute of Technology Patna, India
Copyright © 2022 Chakraborty, Dash, Elavarasan, Kaur, Elangovan, Meraj, Kasinathan and Said. This is an open-access article distributed under the terms of the Creative Commons Attribution License (CC BY). The use, distribution or reproduction in other forums is permitted, provided the original author(s) and the copyright owner(s) are credited and that the original publication in this journal is cited, in accordance with accepted academic practice. No use, distribution or reproduction is permitted which does not comply with these terms.
*Correspondence: Suprava Chakraborty, c3VwcmF2YUBlZS5pc20uYWMuaW4=; Rajvikram Madurai Elavarasan, cmFqdmlrcmFtNzg3QGdtYWlsLmNvbQ==; Devaraj Elangovan, ZWxhbmdvdmFuLmRldmFyYWpAdml0LmFjLmlu