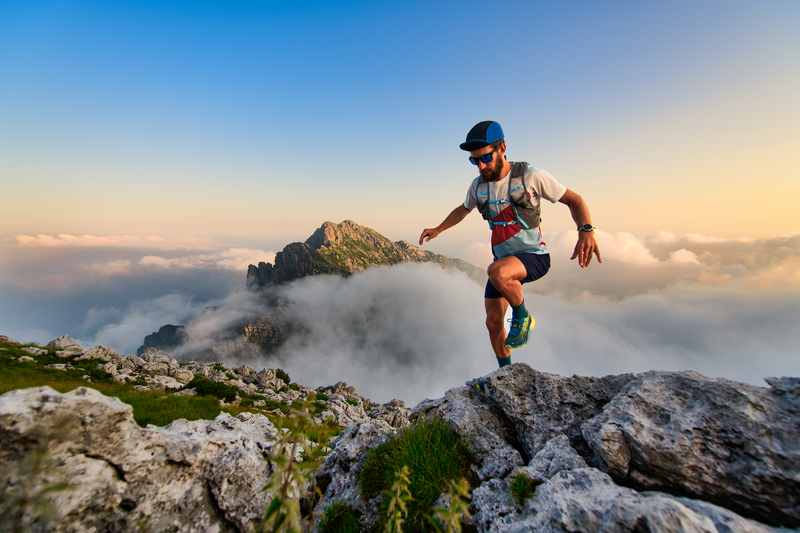
95% of researchers rate our articles as excellent or good
Learn more about the work of our research integrity team to safeguard the quality of each article we publish.
Find out more
ORIGINAL RESEARCH article
Front. Energy Res. , 02 June 2022
Sec. Sustainable Energy Systems
Volume 10 - 2022 | https://doi.org/10.3389/fenrg.2022.873800
Large-scale electrochemical energy storage (EES) can contribute to renewable energy adoption and ensure the stability of electricity systems under high penetration of renewable energy. However, the commercialization of the EES industry is largely encumbered by its cost; therefore, this study studied the technical characteristics and economic analysis of EES and presents a detailed analysis of the levelized cost of storage (LCOS) for different EES technologies. The results show that in the application of energy storage peak shaving, the LCOS of lead-carbon (12 MW power and 24 MWh capacity) is 0.84 CNY/kWh, that of lithium iron phosphate (60 MW power and 240 MWh capacity) is 0.94 CNY/kWh, and that of the vanadium redox flow (200 MW power and 800 MWh capacity) is 1.21 CNY/kWh. A detailed analysis of the cost breakdown shows that the proportion of the Capex and charging costs of EES projects are relatively high, while the Opex and tax costs are comparatively low. The difference between EES projects lies in the proportion of replacement costs. Finally, a sensitivity analysis considering four factors is presented, with this study considering the impact of round-trip efficiency, storage duration, unit initial investment, and the storage application scenario on the LCOS of EES. Among them, the LCOS varies with different application scenarios. For transmission and distribution (T&D) application, the LCOS of lithium iron phosphate is the lowest, due to its long-life advantage compared to lead-carbon.
The contradiction between human activities and the ecological environment has become increasingly prominent since the 20th century (Yu et al., 2020). Driven by the national strategic goals of carbon peaking and carbon neutrality, the power industry in China is implementing energy transition response policies, increasing the proportion of renewable energy connected to the grid, and building a new power system with renewable energy as the mainstay. Nevertheless, the power generation capacity of renewable energy such as wind and solar is variable and intermittent, which makes it difficult to provide a stable power supply for the system. Electricity cannot be simply stored, and wind and solar curtailment is costly, which leads to insufficient renewable energy adoption. The combination of energy storage and renewable energy in power operation has significant advantages in improving the flexibility of a power system, promoting renewable energy adoption, and ensuring the security of the power grid (Shafiullah et al., 2013; Kyriakopoulos and Arabatzis, 2016; Sakti et al., 2018; Gaudard and Madani, 2019). In the future, new power systems dominated by renewable energy will have an increasingly urgent demand for flexible power supplies, and the energy storage industry is ready to launch.
In order to achieve carbon neutrality in China by 2060, the establishment of a “near-zero emission” energy system based primarily on renewable energy will be indispensable. Energy storage is the overriding technology that can accelerate energy transition and buffer the mismatch between power demand and supply (Brandt et al., 2021; Murphy et al., 2021). The large-scale development of energy storage is a crucial technological approach to the development of low-carbon energy, the aims of which are to improve the adoption and storage capacity of renewable energy. The development of energy storage is mediated by the maturity of technology (Kousksou et al., 2014; Pierpoint, 2016), capacity allocation optimization (Ma et al., 2019; Hou et al., 2020; Jin et al., 2020; Li et al., 2020), system optimization scheduling of energy storage (Geem and Yoon, 2017; Hou et al., 2019), and energy storage policy (Ming et al., 2013; Winfield et al., 2018; McPherson et al., 2020; Varghese and Sioshansi, 2020). However, in the initial development stage, economic analysis is pivotal in the process of the technological development of energy storage (Ye et al., 2016). A reduction in the cost of energy storage technology will shorten the payback period of investment. The levelized cost of storage (LCOS) based on energy storage life cycle modeling is considered to be one of the international general energy storage cost evaluation indexes.
There has been a prolific number of research works employing the LCOS to analyze the economics of different storage systems. The International Renewable Energy Agency (IRENA) released the cost-of-service tool version 1.0 in 2017 to estimate the cost of energy storage in different application scenarios (Ralon et al., 2017). Lazard (2018) released a report to analyze the observed costs and revenue streams associated with commercially available energy storage technologies. Zakeri and Syri (2015) adopted an LCOS model to comprehensively analyze the economy of five types of energy storage technologies (physical energy storage, EES, superconducting magnetic energy storage, supercapacitors, and hydrogen energy storage) in three main applications (large-capacity energy storage, transmission and distribution support services, and frequency regulation). Schmidt et al. (2017) constructed an empirical curve to predict the levelized cost of 11 electricity storage technologies using the LCOS. Schmidt et al. (2019) employed an LCOS model to determine the life costs of nine energy storage technologies in 12 power system applications from 2015 to 2050. Obi et al. (2017) discussed the variables that affect the LCOS of energy storage systems and calculated the energy storage costs of physical energy storage (pumped storage systems and compressed air systems) and chemical batteries. Pawel (2014) exhaustively examined the costs of different power storage technologies, inter alia, the costs of long-term storage systems (100 MW power and 70 GWh capacity), and short-term storage systems (100 MW power and 400 MWh capacity) were analyzed. Jülch (2016) conceived a new framework for calculating the cost of energy storage, which was used to calculate the LCOE of combined photovoltaic and energy storage power plants.
At present, EES is developing rapidly in China; however, the economics of EES technologies are ambiguous, which restricts further development of EES. He et al. (2019) calculated the cost per kilowatt-hour and cost per mileage of energy storage technologies and analyzed the full life cycle of energy storage in terms of the typical application scenarios of capacity and power energy storage. Xue et al. (2016) framed a general life cycle cost model to holistically calculate various costs of consumer-side energy storage, the results of which showed the average annual cost of battery energy storage on the consumer side of each category from low to high, namely, lead-acid battery < sodium sulfur battery (NaS) = lithium iron battery < vanadium redox flow battery. In summary, there is limited research analyzing the economics of large-scale EES deployment in China. Hence, it is of practical value to employ the LCOS to evaluate domestic EES projects. This study took large-scale EES projects as the research object to evaluate the average annual cost of different technologies by applying an LCOS model on lead-carbon, lithium iron phosphate, and vanadium redox flow projects.
The aims and contributions of the presented research are as follows: 1) to present the energy storage development policies over time in China and to summarize the technical characteristics of EES in China, that is, technical maturity, energy density, power density, charge/discharge cycle, roundtrip efficiency, etc.; 2) to develop an LCOS method for evaluating the levelized cost of storage by considering the technical and economic parameters and the costs, including Capex, Opex, charging cost, tax cost, replacement cost, and end-of-life cost; 3) to calculate the LCOS of three typical EES projects with different EES technologies, including lead-carbon, lithium iron phosphate, and vanadium redox flow batteries—by comparing the LCOS, the cost breakdown and its causes were analyzed; and 4) to analyze the sensitivity of four factors to the LCOS, including the round-trip efficiency, storage duration, unit initial investment, and storage application scenario, to better promote the development of EES.
The remainder of the article is organized as follows: Section 2 presents the energy storage development status across the world and in China. Section 3 provides the technical characteristics of EES in China. The methodology for assessing the LCOS of EES is established in Section 4. Section 5 shows the results and discussion. Finally, conclusions are given in Section 6.
The cumulative installed capacity of global energy storage in 2014–2020 is shown in Figure 1. According to the statistics reported by the China Energy Storage Alliance (CNESA), by the end of 2020, a total of 191.1 GW of energy storage projects had been put into operation worldwide. The breakdown of global energy storage projects in 2020 by technology distribution is shown in Figure 2. The proportion of EES was 7.5%, exceeding 10 GW for the first time. Among the different types of EES, the cumulative installed capacity of lithium iron batteries was the largest, accounting for 92% of the total installed capacity of EES.
FIGURE 2. Breakdown of global energy storage projects by technology distribution in 2020 (source: CNESA).
After pumped hydro, EES is the most widely used energy storage technology with great developmental potential. By the end of 2020, the cumulative installed capacity of EES had reached 14.2 GW. The cumulative installed capacity and growth rate of the global EES in 2014–2020 are shown in Figure 3.
FIGURE 3. Cumulative installed capacity and growth rate of the global EES in 2014–2020 (source: CNESA).
From 2016 to 2020, the energy storage industry in China steadily expanded, with the installed capacity rising from 24.3 GW in 2016 to 35.6 GW in 2020. Figure 4 shows the cumulative installed capacity of energy storage for China in 2016–2020. In 2020, the cumulative installed capacity in China reached 35.6 GW, a year-on-year increase of 9.8%, accounting for 18.6% of the global total installed capacity. Pumped hydro accounted for 89.30%, followed by EES with a cumulative installed capacity of 3.27 GW, accounting for 9.2%. Among all types of EES, the cumulative installed capacity of lithium iron batteries was the largest, accounting for 88.8%, with an installed capacity of 2.90 GW. The installed structure distribution of energy storage projects for China in 2020 is shown in Figure 5.
FIGURE 5. Installed structure distribution of energy storage projects for China in 2020 (source: CNESA).
By the end of 2020, the cumulative installed capacity of EES in China was 3269.2 MW, with a year-on-year growth of 91.2%. The cumulative installed capacity of newly added EES reached 1.56 GW, transcending 1 GW for the first time. In 2018, with the explosive growth in EES, the newly installed capacity of EES was as high as 612.8 MW compared to the newly added 147.3 MW in 2017, a year-on-year increase of 316%. Figure 6 shows the cumulative installed capacity and growth rate of EES in China from 2011 to 2020.
In 2005, the “Guiding Catalogue for the Development of Renewable Energy Industry,” which was issued by the National Development and Reform Commission (NDRC), began to involve the strategic layout of the energy storage industry. With the continuous expansion of market demand for the renewable energy industry, energy storage development was written into the bill for the first time in 2010. In the “Renewable Energy Law Amendment,” it was stipulated that power grid enterprises should develop and apply smart grid and energy storage technology.
At the end of the 12th Five-Year Plan, policies on energy storage began to be issued frequently. The “Energy Development Strategy Action Plan (2014–2020)” mentioned that energy storage should be used to solve the problem of renewable energy adoption and should promote reformation of the power system. At the same time, in the “Several Opinions on Further Deepening the Reform of the Power System,” it was pointed out that the Chinese energy sector should actively develop microgrid and smart grid technology that integrates advanced energy storage technology and information technology to improve the system energy consumption capacity and energy utilization efficiency. The reform of the power system and a series of supporting documents created a more flexible and market-oriented environment and widened the application space of energy storage in the power system.
During the 13th Five-Year Plan period, the energy storage strategy occupied a more important position. In 2016, in the “Outline of the 13th Five-Year Plan for the National Economic and Social Development of the People’s Republic of China,” the development of energy storage and distributed energy was included in the 100 major projects that China plans to implement in the next 5 years, and energy storage officially entered the national development plan for the first time, laying a good foundation for the introduction of energy storage policies in the future. At the same time, at the end of the 13th Five-Year Plan, the NDRC made it clear that it was necessary to promote energy storage to participate in power market transactions, which provided a guarantee for further promoting the development of energy storage.
Entering the new stage of the 14th Five-Year Plan, the energy storage industry has matured, and the key position of energy storage in the construction of China’s energy system in the future is becoming more and more prominent. The energy storage industry has ushered in rapid development, and the speed of policy introduction has been significantly accelerated. Driven by the policies, energy storage is changing from “optional” in the past to “mandatory” in the future power system. Table 1 summarizes the policies of China’s energy storage industry.
Energy storage is the process of storing energy through media or equipment and releasing it when needed (Hua, 2019). Energy storage enables the temporal and spatial transfer of electric energy, which can effectively isolate the production and utilization of electric power. It also eliminates the peak valley difference of day and night power consumption, improves resource utilization efficiency, and ensures the large-scale grid connection of renewable energy (Shafiullah et al., 2013; Taylor et al., 2013). Meanwhile, energy storage devices can improve the peak shaving and frequency modulation capacity of the system; they reduce the investment in the construction of peak-shaving power stations, reserve capacity, and expansion of power transmission and distribution (CNESA, 2019; Giarola et al., 2021).
EES technology has broad application prospects in the field of power systems due to its characteristics of high-energy density, high specific power, fast response, high reliability, long life, flexible installation, etc. EES is a popular research topic and key developmental direction in different countries around the world (Wang et al., 2020). At present, the main EES technologies in China include lead, lithium iron, flow batteries, NaS, and supercapacitors. Table 2 summarizes the main characteristics of the EES technology in China.
In order to evaluate the cost of energy storage technologies, it is necessary to establish a cost analysis model suitable for various energy storage technologies. The LCOS model is a tool for comparing the unit costs of different energy storage technologies. It can be described as the total lifetime cost of energy storage technology divided by its cumulative delivered electricity using the discount rate
Despite the increasing research on the LCOS, there is no unified understanding of the calculation method of energy storage costs. While some studies have ignored economic parameters such as replacement or charging costs, others have excluded technical parameters such as
In this study, the LCOS considers all technical and economic parameters affecting the lifetime cost. EES costs include Capex, Opex, charging cost, tax cost, replacement cost, and end-of-life cost. The LCOS quantifies the discounted cost per unit of discharged electricity for a specific storage technology. The calculation method of the LCOS is similar to the LCOE of power generation technology, so it is a comparable and suitable tool for comparing the cost of power storage technology (Kousksou et al., 2014; Zakeri and Syri, 2015; Jülch, 2016; Lai et al., 2017b, 2019; Lai and McCulloch, 2017b; Schmidt et al., 2017, 2019).
Stakeholders can use the LCOS model to calculate the cost of different energy storage technologies, compare the results, and analyze the competitiveness of each energy storage technology, so as to make better decisions and promote the development of the energy storage industry. For example, market participants can choose lower-cost energy storage technology to maximize their benefits. For system operators, they can select a more appropriate energy storage technology to solve the problems of limited power grid consumption capacity and insufficient peak shaving capacity. Additionally, by analyzing the costs of different energy storage technologies, policymakers can help guide the development policies of the energy storage industry, such as the subsidy policy, in order to provide reference for the development planning and sustainable development operation of the energy storage industry against the background of large-scale grid connection of renewable energy in China.
where
This study calculated the LCOS by evaluating the discharged electricity and various costs from construction and operations. The following variables were the principal inputs for calculating the LCOS.
Capital expenditure (Capex): The initial investment cost includes the energy storage system cost, the power conversion cost, and the civil construction cost. Among them, the system cost is mainly composed of equipment installation costs (including battery costs) and construction costs (excluding land costs). The energy storage equipment includes energy storage batteries, battery management systems, energy storage inverters, and power distribution systems. The construction costs mainly include construction engineering fees, installation engineering fees, and equipment and facility design and commissioning expenses.
Operation and maintenance costs (Opex): The operation and maintenance costs are those costs needed to maintain the energy storage power station in a good standby state. These costs include maintenance costs (Cm), labor costs (Cl), insurance costs (Ci), power station management costs (Cpsm), and other fixed costs (Coth).
Financial costs: These include the own capital ratio (P), loan interest rate (Rl) and load period (Pl), after-tax internal return rate (IRR), service lifetime (Ps), and deprecation rate (q).
Tax costs: These include income tax (Ti), value-added tax (VAT), land use tax (Tl), urban maintenance and construction tax (Tu), and education surcharges (Te).
Charging costs: Electricity price and
Replacement costs: These include the percentage of annual reduction in the battery installation costs, battery replacement times, battery lifetime, etc.
End-of-life cost: These include asset evaluation fees, clean-up fees, dismantling and transportation fees, recycling and regeneration treatment fees, and recycling value.
Figure 7 clearly shows the cost structure of the LCOS.
In the aforementioned EES technologies, lead acid, lithium iron, flow, and NaS batteries can be employed in large-scale EES projects. In China, the use of NaS batteries started late. The current demonstration project is a 100 kw/800 kwh NaS energy storage system developed by the Institute of Silicate, Chinese Academy of Sciences, in cooperation with Shanghai Electric Power Company; there is no megawatt-level application demonstration case yet. Therefore, this study selected typical large-scale EES projects in China (the Huzhou 10 kV Bingchen 12 MW/24 MWh lead-carbon energy storage project, the Gansu Jiuquan Zhongneng brunji 60 MW/240 MWh energy storage project, and the Dalian liquid flow battery 200 MW/800 MWh energy storage project) to study the LCOS of lead-carbon, lithium iron phosphate, and vanadium redox flow batteries. Their application scenario is energy storage peak shaving, which is designed to charge at night and discharge during the day. Thus, these systems are assumed to charge and discharge once during each 24-h period at full charge/discharge depth. The power capacity, energy capacity, total initial investment, etc., are set according to the design value of the actual project. The battery and energy storage system costs are determined in proportion to the initial investment. The battery life, recycling coefficient (
(1) Capital expenditure (Capex)
The whole life cycle of an EES project is divided into a construction period and an operation period. The Capex occurs in the construction period of the project, which refers to the fixed asset investment. It mainly includes the energy storage system cost
where
where
This study calculated the cost of three EES technologies in the energy-usage scenario.
where
where
(2) Operation and maintenance cost (Opex)
During the life cycle of an EES project, in order to ensure its normal operation, the power station needs to be maintained every year. The Opex mainly includes the insurance, transportation, maintenance, wages, and welfare. Insurances and repair charges are given in the form of rates, which are 0.5 and 2% of the plant’s fixed assets per year, respectively (Hahn et al., 2017). According to the actual survey data, it is estimated that the annual employee salary in the power station is 80,000 CNY. The welfare labor insurance coefficient is set at 60% of the total salary, and the employee wage growth rate is 6%.
where
(3) Financial cost
The construction cost of the project is relatively high, and most of the investment comes from bank loans. According to the “Notice of the State Council on Strengthening the Capital Management of Fixed Asset Investment Projects,” this study set the capital proportion to 20%, with the other funds financed by bank loans (State Council, 2019). Considering the time value of the funds, a discount rate of 8% is adopted to discount the funds in the whole life period of the project (Schmidt et al., 2019). Since October 2015, the People’s Bank of China has adjusted the long-term loan interest rate to 4.9% (Monetary Policy Department, 2015). The loan period is 15 years, the design lifetime of the power station is 20 years, and the depreciation period is 15 years (NEA, 2015a; Hahn et al., 2017). According to the standard of the power industry, the internal rate of return (IRR) of an EES project is set at 8%.
(4) Tax cost
The tax basis for tax purposes is different and needs to be determined according to the situation. Under the implementation of the policy of “Replacing Business Tax with Value-added Tax,” the operating tax of an EES project is not calculated. According to “Enterprise income tax law of the people’s Republic of China,” the enterprise income tax is levied at a tax rate of 15% on enterprises (STA, 2019a). According to the “Regulations on the Implementation of the Enterprise Income Tax Law of the People’s Republic of China,” from the tax year of the first production and the operation income of the project, the enterprise income tax is exempted from the first to the third year and is reduced by half from the fourth to the sixth year (STA, 2019b). Value-added tax has become one of the most important taxes in China. The NEA issued “The Notice on Reducing the Tax Burdened on Enterprises in the Field of Renewable Energy” and implemented a VAT rate of 6.5% (China Power Grid, 2017; STA, 2019c). Land use tax is levied on the land within the scope of cities, counties, organizational towns, and industrial and mining areas, and the actual occupied land area is the basis for tax calculation. The land use tax is levied at 2 CNY/m2 (Yuan et al., 2016). According to the relevant regulations on property tax, the tax rate is 1.2% and the property tax deduction ratio is 30% (TCPGPRC, 2005a). In addition, urban maintenance and construction tax and education surcharges are based on value-added tax, which are 4 and 3%, respectively (TCPGPRC, 2005b; TCPGPRC, 2020).
(5) Charging cost
According to Obi et al. (2017), this study adopted off-peak electricity prices. The charging cost can be expressed as follows:
where
(6) Replacement cost
In an EES system, the battery has capacity degradation, a decrease in performance, and a limited usage time. If the battery’s lifetime is shorter than the project’s, the replacement cost needs to be considered. As battery technology matures and expands, battery costs will drop year on year. Therefore, it is necessary to consider the proportion of battery cost reductions when determining battery replacement costs. During the project cycle, the replacement cost of a battery each time can be expressed as follows:
where
Therefore, the discounted value of replacement cost of the battery can be expressed as follows:
where
(7) End-of-life cost
After the end of the service life of the energy storage power station, the assets of the power station need to be disposed of, and the end-of-life costs mainly include asset evaluation fees, clean-up fees, dismantling and transportation fees, and recycling and regeneration treatment fees. The metal materials and some components in the power plant can be recovered. Therefore, the residual value of an energy storage power station is defined as the residual value at the end of the life of the power station, excluding the disposal cost. If the disposal fee is greater than the recycling value of the power station, it is the cost; otherwise, it is the income.
Therefore, the discounted value of the end-of-life cost of can be expressed as follows:
Where
(8) Discharged electricity
Energy capacity, self-discharge, and
where
In Figure 8, the results show that the LCOS of lead-carbon is 0.84 CNY/kWh, that of lithium iron phosphate is 0.94 CNY/kWh, and that of vanadium redox flow is 1.21 CNY/kWh.
Lead-carbon batteries are a new generation of lead acid batteries, in which carbon is added into the negative active material of traditional lead acid batteries. China has prolific lead resources, which can be simply processed with raw material of a lower cost. Additionally, the lead-carbon battery recycling system is relatively mature, as it is easier to recycle active materials from used batteries. This type of battery is easy to recycle because the residual value is quite high and the Capex is significantly lower than those of the other two types of battery. This is why the value of the LCOS of lead-carbon batteries is the smallest.
Lithium iron phosphate batteries have a long life cycle, with a 95% round-trip efficiency and a low charging cost. However, this type of energy storage project still faces many adversities. For instance, during the disposal of recycled batteries, the recycling cost is high, inter alia, the end-of-life cost of the lithium element is five times that of original lithium batteries. The positive and negative electrode materials of the batteries, the material side reactions of the electrolyte, the internal short circuit of the battery cores, and so on cause a high Ou of lithium iron phosphate batteries, as well as a power loss. Taking the above factors into consideration, the LCOS value of the lithium iron phosphate in EES projects is slightly higher than that of lead-carbon.
At present, lithium iron phosphate batteries are more widely used than lead-carbon batteries. However, regarding the repeated occurrence of safety incidents in lithium battery storage power stations around the world, lead-carbon batteries have become another development direction of EES projects due to the safety of lead-carbon batteries (Yang, 2021; CIEIN, 2022). Lead-carbon batteries currently have a good development momentum in China. Due to their low initial investment, high residual value, and easy recycling, the LCOS of lead-carbon batteries is the lowest.
Vanadium ions are the sole electrolyte ions of vanadium redox flow batteries. Changes in the valence state in vanadium ions occur during charging and discharging without the phase changes that other batteries commonly have. After long-term utilization, fast charge and discharge responses can still be maintained. When a battery’s life ends, the electrolyte solution can be recycled, the cost of which accounts for more than 50% of the total cost of the energy storage system, so the residual value is extremely high after the energy storage system is scrapped. The life cycle of vanadium redox flow batteries is much longer than those of lead-carbon and lithium iron phosphate batteries. The former batteries only need to be replaced once during the lifetime of the energy storage project, so the replacement cost is relatively low. The positive and negative electrolytes of the batteries are stored separately, and the Ou is very low when the batteries are in standby mode. However, the shortcomings are evident. First of all, such projects require independent storage tanks, reaction tanks, and facilities such as pumps and different types of pipelines, which take up more space and result in a higher Capex. Second, this type of EES project is still in its initial stage, with immature technology and a lower
The charging cost of an EES project is a significant part of the LCOS. It is in direct proportion to the electricity price and is inversely proportional to the
The cost breakdown of the three different types of battery technologies in EES projects is shown in Figure 9. The common point that the three projects have is that the Capex and charging cost account for a large proportion, while the Opex and tax cost account for the smallest proportion. The costs of lithium iron phosphate and vanadium redox flow in each category are basically the same; the Capex and charging cost account for the largest proportion, with the replacement cost being the second largest. The proportion of the Capex of lead-carbon drops significantly compared to the other two; instead, the proportion of replacement cost increases for lead-carbon batteries, which have a short life cycle and require three replacements during the lifetime of an energy storage project. The replacement cost is much higher than that for the other two types of batteries, but the raw material reserves for lead-carbon technology are prolific, the battery cost is low, and the recycling value is high; therefore, the Capex is less.
According to Schmidt et al. (2017), the experience rates (based on the production price) of lead-carbon, lithium ion, and vanadium redox flow are 4, 12, and 11%, respectively, and are based on the works of Obi et al. (2017) and Jülch (2016). This study selected four factors, namely, round-trip efficiency (±5%), storage duration (±50%), unit initial investment (±20%), and storage application scenario, to explore the impact of technology learning on the LCOS of EES. The results are shown in Figures 10–13.
In Figure 10, the LCOS of the three types of EES decreases with the increase in round-trip efficiency, and the decrease pattern is basically similar.
In Figure 11, the LCOS of the three types of EES increases with the increase in the unit initial investment cost. The rate of vanadium redox flow is the fastest, followed by lithium iron phosphate, while lead-carbon is the slowest. Therefore, vanadium redox flow is the most sensitive to unit initial investment cost, followed by lithium iron phosphate, while lead-carbon is the least sensitive. The experience rate (based on production price) of lead-carbon batteries is much lower than that of the other batteries (Schmidt et al., 2017). Therefore, compared to lead-carbon, the initial investment cost of lithium iron phosphate and vanadium redox flow has a higher declining slope, resulting in a faster decline of the LCOS.
In Figure 12, the LCOS of the three types of EES decreases with the increase in storage duration. The decreasing rate of vanadium redox flow is the fastest, followed by lithium iron phosphate, while lead-carbon is the slowest. This means that vanadium redox flow is the most sensitive to storage duration, followed by lithium iron phosphate, while lead-carbon is the least sensitive. The technical advantage of vanadium redox flow is the medium- to long-term energy storage range of 4–10 h. When the vanadium redox flow increases by 50% (6 h), the LCOS is 0.94 CNY/kWh, which decreases by 29%. With the decrease in the initial investment cost of the battery and the extension of storage duration (which is its unique technical advantage), the vanadium redox flow is expected to compete with other technologies.
For transmission and distribution (T&D) application, storage systems charge/discharge twice during each 24-h period. In Figure 13, the results show that the LCOS of lead-carbon is 0.89 CNY/kWh, that of lithium iron phosphate is 0.79 CNY/kWh, and that of vanadium redox-flow is 1.13 CNY/kWh in T&D application.
In this scenario, the battery capacity is not fully utilized and the battery life increases. Lithium iron phosphate takes advantage of its long life. It only needs to be replaced once during the lifetime of the EES project, and the amortized value of the replacement cost over the whole lifecycle is 0.05 CNY/kWh, while that of lead-carbon battery is 0.21 CNY/kWh. This is the main reason why the LCOS of lithium iron phosphate is the smallest. Therefore, investors should give full play to the advantages of different technology types to maximize the income when arranging EES.
In China, the cumulative installed capacity of lithium-ion batteries accounted for 88.8% of the EES in 2020. Lithium iron phosphate batteries are the predominant EES technology, as they are the most widely used and commercialized at present in China. Lead-carbon batteries have the highest safety due to their lack of combustibles during operation. They have also become an option for large-scale EES technology. The cost of vanadium redox flow is high in the initial stage of development, but with the development of technology and industry, the cost will be greatly lowered. Moreover, the scale design of this type of battery is very flexible. The energy storage capacity depends on the electrolyte capacity and concentration. As long as the volume of the electrolyte is increased, the energy storage capacity can be enhanced. Therefore, vanadium redox flow will be the most promising large-scale EES technology in the future.
As a high-quality flexible resource, energy storage can play an important regulatory role in a high proportion of renewable energy power systems. With the increasing proportion of renewable energy in power generation, energy storage technology has great potential in energy systems. In recent years, the demand for EES in China has greatly increased, the installed capacity of EES on the power supply and grid sides continues to rise, and large-scale energy storage presents a rapid developmental trend.
This study combed the development process of energy storage policy and summarized the characteristics of EES technology in China and then analyzed the levelized cost of storage of three different types of EES power stations based on the LCOS model. The costs consisted of Capex, Opex, charging cost, tax cost, replacement cost, and end-of-life cost. This study calculated the LCOS by evaluating the discharged electricity and various costs related to construction and operation. Finally, the round-trip efficiency, storage duration, unit initial investment, and storage application scenario were selected as sensitivity factors, and sensitivity analysis of the four factors to the LCOS of EES was employed.
From the results, in the application scenario of energy storage peak shaving, due to the abundant lead resources and mature lead-carbon battery recycling system, the initial investment cost of lead-carbon batteries is significantly lower than that of the other two; the LCOS of lead-carbon is 0.84 CNY/kWh, which is the smallest. Due to the long life cycle but high end-of-life cost, the LCOS of lithium iron phosphate batteries is 0.94 CNY/kWh, which is in the middle. Due to the high recycling value and high investment cost, vanadium redox flow technology is still immature, with an LCOS of 1.21 CNY/kWh, which is the highest.
From the cost breakdown, it is indicated that the proportion of Capex and charging cost is the largest, while the proportion of tax cost and Opex is the smallest. The difference among the three projects lies in the fact that lead-carbon has a short life cycle with many replacements, which results in a large cost. The distinction of costs in each part was presented in this article.
From the sensitivity analysis, with an increase in the round-trip efficiency factor, the LCOS of the three types of EES decreases linearly with similar trends. With a decrease in the unit initial investment cost factor, the LCOS values of the three types of EES all show a downward trend, with that of vanadium redox flow being the most sensitive. With an increase in the storage duration factor, the LCOS of vanadium redox flow decreases the fastest, followed by that of lithium iron phosphate, while that of lead-carbon is the slowest. The LCOS varies with different application scenarios. For T&D application, LCOS is the lowest because the lithium iron phosphate batteries only need to be replaced once, taking advantage of their long life. In summary, stakeholders should minimize the cost of EES by improving round-trip efficiency and storage duration, reducing the unit initial investment cost, and giving full play to the advantages of different technology types when laying out EES.
The limitations of the presented research can be seen in the fact that the results are based on the economic evaluation of three types of EES projects. In cases of other types of EES, the technical characteristics will be different, especially in different application scenarios. Further research will be more focused on the comprehensive value of multiple applications of energy storage in power systems, especially in the ancillary services market and other services.
The original contributions presented in the study are included in the article/Supplementary Material; further inquiries can be directed to the corresponding author.
YX: conceptualization, methodology, and writing—original draft. JP and LC: data curation, visualization, and software. PL: investigation and validation. TM: resources and writing—reviewing and editing.
This study is supported by the National Natural Science Foundation of China (41901251), the Key Projects in Soft Science Research of Shanghai Science and Technology Commission (21692109400), the Program for the Innovative Talents of Higher Education Institutions of Shanxi (PTIT), and the Program for the Philosophy and Social Sciences Research of Higher Learning Institutions of Shanxi (2020W061).
The authors declare that the research was conducted in the absence of any commercial or financial relationships that could be construed as a potential conflict of interest.
All claims expressed in this article are solely those of the authors and do not necessarily represent those of their affiliated organizations, or those of the publisher, the editors, and the reviewers. Any product that may be evaluated in this article, or claim that may be made by its manufacturer, is not guaranteed or endorsed by the publisher.
The authors would also like to acknowledge the reviewers for their valuable comments which largely improved the academic quality of this article. The usual caveats apply.
Berrada, A., and Loudiyi, K. (2016). Operation, Sizing, and Economic Evaluation of Storage for Solar and Wind Power Plants. Renew. Sustain. Energy Rev. 59, 1117–1129. doi:10.1016/j.rser.2016.01.048
Brandt, A. R., Teichgraeber, H., Kang, C. A., Barnhart, C. J., Carbajales-Dale, M. A., and Sgouridis, S. (2021). Blow Wind Blow: Capital Deployment in Variable Energy Systems. Energy 224, 120198. doi:10.1016/j.energy.2021.120198
China Industrial Economic Information Network (2022). From Electrode Champion to Energy Storage Rookie Kungong Technology Pioneered the Technology of Large Capacity Lead Carbon Long-Term Battery. Available at: http://www.cinic.org.cn/zgzz/cx/1269196.html (Accessed April 12, 2022).
China Power Grid (2017). Reducing the VAT Tax Burden of Hydropower Enterprises: Notice of the National Energy Administration on Reducing the Tax Burden of Enterprises in the Field of Renewable Energy. Available at: http://news.bjx.com.cn/html/20170912/849523.shtml (Accessed January 8, 2022).
Gan, Su. (2020). Lithium Iron Phosphate Battery Project. Available at: https://www.163.com/dy/article/FL4RFQLV0534697F.html (Accessed January 8, 2022).
Gaudard, L., and Madani, K. (2019). Energy Storage Race: Has the Monopoly of Pumped-Storage in Europe Come to an End? Energy Policy 126, 22–29. doi:10.1016/j.enpol.2018.11.003
Geem, Z. W., and Yoon, Y. (2017). Harmony Search Optimization of Renewable Energy Charging with Energy Storage System. Int. J. Electr. Power & Energy Syst. 86, 120–126. doi:10.1016/j.ijepes.2016.04.028
Giarola, S., Molar-Cruz, A., Vaillancourt, K., Bahn, O., Sarmiento, L., Hawkes, A., et al. (2021). The Role of Energy Storage in the Uptake of Renewable Energy: A Model Comparison Approach. Energy Policy 151, 112159. doi:10.1016/j.enpol.2021.112159
Hahn, H., Hau, D., Dick, C., and Puchta, M. (2017). Techno-economic Assessment of a Subsea Energy Storage Technology for Power Balancing Services. Energy 133, 121–127. doi:10.1016/j.energy.2017.05.116
He, Y., Chen, Y., Liu, Y., Liu, H., and Liu, H. (2019). Analysis of Cost Per Kilowatt-Hour and Cost Per Mileage for Energy Storage Technologies. Adv. Technol. Electr. Eng. Energy 38 (9), 1–10. doi:10.12067/ATEEE1907045
Hou, H., Xu, T., Wu, X., Wang, H., Tang, A., and Chen, Y. (2020). Optimal Capacity Configuration of the Wind-Photovoltaic-Storage Hybrid Power System Based on Gravity Energy Storage System. Appl. Energy 271, 115052. doi:10.1016/j.apenergy.2020.115052
Hou, P., Hu, J., and Yang, G. (2019). Convex Optimization of Virtual Storage System Scheduling in Market Environment. J. Mod. Power Syst. Clean. Energy 7, 1744–1748. doi:10.1007/s40565-019-0548-z
Hua, Z. (2019). Key Technologies and Business Operation Modes of Energy Storage. Beijing: China Electric Power Press.
Huang, B., Pan, Z., Su, X., and An, L. (2018). Recycling of Lithium-Ion Batteries: Recent Advances and Perspectives. J. Power Sources 399, 274–286. doi:10.1016/j.jpowsour.2018.07.116
Jin, R., Song, J., Liu, J., Li, W., and Lu, C. (2020). Location and Capacity Optimization of Distributed Energy Storage System in Peak-Shaving. Energies 13, 513. doi:10.3390/en13030513
Jülch, V. (2016). Comparison of Electricity Storage Options Using Levelized Cost of Storage (LCOS) Method. Appl. Energy 183, 1594–1606. doi:10.1016/j.apenergy.2016.08.165
Kousksou, T., Bruel, P., Jamil, A., El Rhafiki, T., and Zeraouli, Y. (2014). Energy Storage: Applications and Challenges. Sol. Energy Mater. Sol. Cells 120, 59–80. doi:10.1016/j.solmat.2013.08.015
Kyriakopoulos, G. L., and Arabatzis, G. (2016). Electrical Energy Storage Systems in Electricity Generation: Energy Policies, Innovative Technologies, and Regulatory Regimes. Renew. Sustain. Energy Rev. 56, 1044–1067. doi:10.1016/j.rser.2015.12.046
Lai, C. S., Jia, Y., Lai, L. L., Xu, Z., McCulloch, M. D., and Wong, K. P. (2017a). A Comprehensive Review on Large-Scale Photovoltaic System with Applications of Electrical Energy Storage. Renew. Sustain. Energy Rev. 78, 439–451. doi:10.1016/j.rser.2017.04.078
Lai, C. S., Jia, Y., Xu, Z., Lai, L. L., Li, X., Cao, J., et al. (2017b). Levelized Cost of Electricity for Photovoltaic/biogas Power Plant Hybrid System with Electrical Energy Storage Degradation Costs. Energy Convers. Manag. 153, 34–47. doi:10.1016/j.enconman.2017.09.076
Lai, C. S., Locatelli, G., Pimm, A., Li, X., and Lai, L. L. (2019). Levelized Cost of Electricity Considering Electrochemical Energy Storage Cycle-Life Degradations. Energy Procedia 158, 3308–3313. doi:10.1016/j.egypro.2019.01.975
Lai, C. S., and McCulloch, M. D. (2017a). Levelized Cost of Electricity for Solar Photovoltaic and Electrical Energy Storage. Appl. Energy 190, 191–203. doi:10.1016/j.apenergy.2016.12.153
Lai, C. S., and McCulloch, M. D. (2017b). Sizing of Stand-Alone Solar PV and Storage System with Anaerobic Digestion Biogas Power Plants. IEEE Trans. Ind. Electron. 64, 2112–2121. doi:10.1109/TIE.2016.2625781
Lazard (2015). Lazard’s Levelized Cost of Storage Analysis V1.0. Available at: https://www.lazard.com/media/2391/lazards-levelized-cost-of-storage.analysis-10.pdf (Accessed January 8, 2022).
Lazard (2018). Lazard’s Levelized Cost of Storage Analysis V4.0. Available at: https://www.lazard.com/media/450774/lazards-levelized-cost-of-storage-version-40-vfinal.pdf (Accessed January 8, 2022).
Li, J., Zhang, Z., Shen, B., Gao, Z., Ma, D., Yue, P., et al. (2020). The Capacity Allocation Method of Photovoltaic and Energy Storage Hybrid System Considering the Whole Life Cycle. J. Clean. Prod. 275, 122902. doi:10.1016/j.jclepro.2020.122902
Ma, W., Wang, W., Wu, X., Hu, R., Tang, F., Zhang, W., et al. (2019). Optimal Allocation of Hybrid Energy Storage Systems for Smoothing Photovoltaic Power Fluctuations Considering the Active Power Curtailment of Photovoltaic. IEEE Access 7, 74787–74799. doi:10.1109/ACCESS.2019.2921316
McPherson, M., Mehos, M., and Denholm, P. (2020). Leveraging Concentrating Solar Power Plant Dispatchability: A Review of the Impacts of Global Market Structures and Policy. Energy Policy 139, 111335. doi:10.1016/j.enpol.2020.111335
Ming, Z., Junjie, F., Song, X., Zhijie, W., Xiaoli, Z., and Yuejin, W. (2013). Development of China's Pumped Storage Plant and Related Policy Analysis. Energy Policy 61, 104–113. doi:10.1016/j.enpol.2013.06.061
Monetary Policy Department (2015). RMB Current Interest Rate Table. (October 24, 2015) Available at: http://www.pbc.gov.cn/zhengcehuobisi/125207/125213/125440/125838/125885/125896/2968988/index.html (Accessed January 8, 2022).
Murphy, C. A., Schleifer, A., and Eurek, K. (2021). A Taxonomy of Systems that Combine Utility-Scale Renewable Energy and Energy Storage Technologies. Renew. Sustain. Energy Rev. 139, 110711. doi:10.1016/j.rser.2021.110711
Nan, Du. (2015). Lead Carbon Battery Project. Available at: http://www.itdcw.com/news/qiye/122K53942015.html (Accessed January 8, 2022).
NDRC (2005). Guiding Catalogue for the Development of Renewable Energy Industry. Available at: http://www.nea.gov.cn/2011-11/21/c_131260306.htm (Accessed January 8, 2022).
NDRC (2016). 13th Five-Year Plan for Renewable Energy Development. Available at: http://www.nea.gov.cn/2016-12/19/c_135916140.htm (Accessed January 8, 2022).
NDRC (2017). Guiding Opinions on Promoting the Development of Energy Storage Technology and Industry. Available at: https://www.ndrc.gov.cn/xxgk/zcfb/tz/201907/t20190701_962470.html?code=&state=123 (Accessed January 8, 2022).
NDRC (2018). Opinions on Innovating and Improving the Price Mechanism for Promoting Green Development. Available at: https://www.ndrc.gov.cn/xxgk/zcfb/ghxwj/201806/t20180629_960951.html?code=&state=123 (Accessed January 8, 2022).
NDRC (2019). Implement the ‘Guiding Opinions on Promoting the Development of Energy Storage Technology and Industry’ Action Plan for 2019-2020. Available at: http://www.gov.cn/xinwen/2019-07/02/content_5405225.htm (Accessed January 8, 2022).
NDRCNEA (2021). Guiding Opinions on Accelerating the Development of Renewable Energy Storage (Draft for Solicitation of Comments). Available at: http://www.gov.cn/xinwen/2021-04/22/content_5601283.htm (Accessed January 8, 2022).
NEA (2015a). Notice on Organizing the Construction of Solar Thermal Power Demonstration Project. Available at: http://zfxxgk.nea.gov.cn/auto87/201509/t20150930_1968.htm (Accessed January 8, 2022).
NEA (2015b). Guiding Opinions on Promoting the Construction of Renewable Energy Microgrid Demonstration Projects. Available at: http://www.nea.gov.cn/2015-07/23/c_134440478.htm (Accessed January 8, 2022).
NPC (2010). Renewable Energy Law Amendment. Available at: http://www.npc.gov.cn/zgrdw/huiyi/cwh/1112/2009-12/26/content_1533216.htm (Accessed January 8, 2022).
NPC (2016). Outline of the 13th Five-Year Plan for the National Economic and Social Development of the People's Republic of China. Available at: https://www.12371.cn/special/sswgh/wen/ (Accessed January 8, 2022).
NPC (2021). The 14th Five-Year Plan for the National Economic and Social Development of the People's Republic of China and the Long-Term Target Plan for 2035. Available at: http://www.gov.cn/xinwen/2021-03/13/content_5592681.htm (Accessed January 8, 2022).
Obi, M., Jensen, S. M., Ferris, J. B., and Bass, R. B. (2017). Calculation of Levelized Costs of Electricity for Various Electrical Energy Storage Systems. Renew. Sustain. Energy Rev. 67, 908–920. doi:10.1016/j.rser.2016.09.043
Pai, P. (2016). Vanadium Redox-Flow Battery Project. Available at: https://www.thepaper.cn/newsDetail_forward_1549936 (Accessed January 8, 2022).
Pawel, I. (2014). The Cost of Storage - How to Calculate the Levelized Cost of Stored Energy (LCOE) and Applications to Renewable Energy Generation. Energy Procedia 46, 68–77. doi:10.1016/j.egypro.2014.01.159
Pierpoint, L. M. (2016). Harnessing Electricity Storage for Systems with Intermittent Sources of Power: Policy and R&D Needs. Energy Policy 96, 751–757. doi:10.1016/j.enpol.2016.04.032
Ralon, P., Taylor, M., and Ilas, A. (2017). Electricity Storage and Renewables: Costs and Markets to 2030. Available at: https://www.irena.org/publications/2017/Oct/Electricity-storage-and-renewables-costs-and-markets (Accessed January 8, 2022).
Sakti, A., Botterud, A., and O’Sullivan, F. (2018). Review of Wholesale Markets and Regulations for Advanced Energy Storage Services in the United States: Current Status and Path Forward. Energy Policy 120, 569–579. doi:10.1016/j.enpol.2018.06.001
Schmidt, O., Hawkes, A., Gambhir, A., and Staffell, I. (2017). The Future Cost of Electrical Energy Storage Based on Experience Rates. Nat. Energy 2, 17110. doi:10.1038/nenergy.2017.110
Schmidt, O., Melchior, S., Hawkes, A., and Staffell, I. (2019). Projecting the Future Levelized Cost of Electricity Storage Technologies. Joule 3, 81–100. doi:10.1016/j.joule.2018.12.008
Sepulveda, N. A., Jenkins, J. D., Edington, A., Mallapragada, D. S., and Lester, R. K. (2021). The Design Space for Long-Duration Energy Storage in Decarbonized Power Systems. Nat. Energy. 6, 506–516. doi:10.1038/s41560-021-00796-8
Shafiullah, G. M., Amanullah, M. T. O., Shawkat Ali, A. B. M., and Wolfs, P. (2013). Potential Challenges of Integrating Large-Scale Wind Energy into the Power Grid-A Review. Renew. Sustain. Energy Rev. 20, 306–321. doi:10.1016/j.rser.2012.11.057
State Council (2014). Energy Development Strategy Action Plan (2014-2020). Available at: http://www.nea.gov.cn/2014-12/03/c_133830458.htm (Accessed January 8, 2022).
State Council (2015). Several Opinions on Further Deepening the Reform of the Power System. Available at: http://www.china-nengyuan.com/news/91900.html (Accessed January 8, 2022).
State Council (2019). Notice on Strengthening Capital Management of Fixed Assets Investment Projects. Available at: http://www.gov.cn/zhengce/content/2019-11/27/content_5456170.htm (Accessed January 8, 2022).
State Taxation Administration (2019a). Enterprise Income Tax Law of the People's Republic of China. Available at: http://www.chinatax.gov.cn/chinatax/n810341/n810825/c101434/c28479830/content.html (Accessed January 8, 2022).
State Taxation Administration (2019b). Regulations on the Implementation of the Enterprise Income Tax Law of the People's Republic of China. Available at: http://www.chinatax.gov.cn/chinatax/n810341/n810825/c101434/c28479831/content.html (Accessed January 8, 2022).
State Taxation Administration (2019c). Announcement on Deepening the Relevant Policies of Value-Added Tax Reformm. Available at: http://www.chinatax.gov.cn/n810341/n810755/c4160283/content.html (Accessed January 8, 2022).
Taylor, P. G., Bolton, R., Stone, D., and Upham, P. (2013). Developing Pathways for Energy Storage in the UK Using a Coevolutionary Framework. Energy Policy 63, 230–243. doi:10.1016/j.enpol.2013.08.070
The Central People's Government of the People's Republic of China (2005a). Provisional Regulations of the People's Republic of China on Real Estate Tax. Available at: http://www.gov.cn/banshi/2005-08/19/content_24823.htm (Accessed January 8, 2022).
The Central People's Government of the People's Republic of China (2005b). Decision of the State Council on Amending the Interim Provisions on the Collection of Educational Surcharges. Available at: http://www.gov.cn/zwgk/2005-09/27/content_70440.htm (Accessed January 8, 2022).
The Central People's Government of the People's Republic of China (2020). Tax Law of the People's Republic of China on Urban Maintenance and Construction. Available at: http://www.gov.cn/xinwen/2020-08/11/content_5534188.htm (Accessed January 8, 2022).
Varghese, S., and Sioshansi, R. (2020). The Price Is Right? How Pricing and Incentive Mechanisms in California Incentivize Building Distributed Hybrid Solar and Energy-Storage Systems. Energy Policy 138, 111242. doi:10.1016/j.enpol.2020.111242
Wang, W., Zhu, H., Gao, Z., Li, H., Li, B., and Li, B. (2020). Progress in Large-Scale Low-Cost Electrochemical Energy Storage Technology and its Application. Mod. Chem. Ind. 40 (10), 80–85. doi:10.16606/j.cnki.issn0253-4320.2020.10.017
Winfield, M., Shokrzadeh, S., and Jones, A. (2018). Energy Policy Regime Change and Advanced Energy Storage: A Comparative Analysis. Energy Policy 115, 572–583. doi:10.1016/j.enpol.2018.01.029
Xue, J., Ye, J., Tao, Q., Wang, D., and Sang, B. (2016). Economic Feasibility of User-Side Battery Energy Storage Based on Whole-Life-Cycle Cost Model. Power Syst. Technol. 40 (8), 2471–2476.
Yang, Y. (2021). Lead Carbon Battery Should Be the First Choice for Large-Scale Energy Storage. Available at: http://paper.people.com.cn/zgnyb/html/2021-09/13/content_25879508.htm (Accessed April 12, 2022).
Ye, J., Tao, Q., Xue, J., Huang, R., Wang, D., and Yang, B. (2016). Economic Progress Analysis of Energy Storage in the Application of Wind Power Integration. Chem. Industry Eng. Prog. 40 (8), 2471–2476. doi:10.16085/j.issn.1000-6613.2016.s2.023
Yu, X., Ma, S., Cheng, K., and Kyriakopoulos, G. L. (2020). An Evaluation System for Sustainable Urban Space Development Based in Green Urbanism Principles-A Case Study Based on the Qin-Ba Mountain Area in China. Sustainability 12, 5703. doi:10.3390/su12145703
Yuan, J., Na, C., Xu, Y., and Zhao, C. (2016). Feed-In Tariff for Onshore Wind Power in China. Emerg. Mark. Finance Trade 52, 1427–1437. doi:10.1080/1540496X.2016.1152797
Zakeri, B., and Syri, S. (2015). Electrical Energy Storage Systems: A Comparative Life Cycle Cost Analysis. Renew. Sustain. Energy Rev. 42, 569–596. doi:10.1016/j.rser.2014.10.011
α Percentage of annual reduction in battery installation costs
β The
γ Recycling coefficient
λP/E Ratio of the nominal power capacity of the energy storage system to the nominal energy capacity
ηbat Conversion efficiency
Capex Capital expenditure
Cbat Battery cells cost
Cc Unit energy cost of civil construction
Cciv Civil construction cost
CE Unit energy cost of battery
Ce Charging electricity price
Chargingn Annual value of charging cost
Ci Insurance cost
Cl Labor cost
Cm Maintenance cost
Com Unit energy cost of the Opex
Coth Other fixed costs
Cp Unit power cost of PCS
Cpcs Power conversion cost
Cpcs-e Unit power conversion cost in the energy-usage scenario
Cpcs-p Unit power conversion cost in the power-usage scenario
Cpsm Power station management cost
Crec Discounted residual value of a power station
Crep Discounted value of the replacement cost of batteries
Csys Energy storage system cost
D Annual operating days
EDischarge Discharge of the energy storage system
Enom Nominal energy capacity
IRR Internal return rate
k Battery replacement times
l Battery lifetime
LCOS Levelized cost of storage
N Service lifetime of the plant
Opexn Operation and maintenance costs
P Own capital ratio
Pl Loan period
Pnom Nominal power capacity
Ps Service lifetime
q Deprecation rate
Rl Loan interest rate
t Nominal discharge time
Taxn Annual tax amount of a power plant
Te Education surcharges
Ti Income tax
Tl Land use tax
Tu Urban maintenance and construction tax
VAT Value-added tax
Keywords: electrochemical energy storage, levelized cost of storage, economy, sensitivity analysis, China
Citation: Xu Y, Pei J, Cui L, Liu P and Ma T (2022) The Levelized Cost of Storage of Electrochemical Energy Storage Technologies in China. Front. Energy Res. 10:873800. doi: 10.3389/fenrg.2022.873800
Received: 11 February 2022; Accepted: 26 April 2022;
Published: 02 June 2022.
Edited by:
Sgouris Sgouridis, Dubai Electricity and Water Authority, United Arab EmiratesReviewed by:
Nikolaos Koltsaklis, Czech Technical University in Prague, CzechiaCopyright © 2022 Xu, Pei, Cui, Liu and Ma. This is an open-access article distributed under the terms of the Creative Commons Attribution License (CC BY). The use, distribution or reproduction in other forums is permitted, provided the original author(s) and the copyright owner(s) are credited and that the original publication in this journal is cited, in accordance with accepted academic practice. No use, distribution or reproduction is permitted which does not comply with these terms.
*Correspondence: Liang Cui, ZGN4eXR5QDE2My5jb20=
Disclaimer: All claims expressed in this article are solely those of the authors and do not necessarily represent those of their affiliated organizations, or those of the publisher, the editors and the reviewers. Any product that may be evaluated in this article or claim that may be made by its manufacturer is not guaranteed or endorsed by the publisher.
Research integrity at Frontiers
Learn more about the work of our research integrity team to safeguard the quality of each article we publish.