- 1CSIRO Energy, Bentley, WA, Australia
- 2Department of Civil and Environmental Engineering, University of Strathclyde, Glasgow, Scotland, United Kingdom
- 3School of Engineering and Innovation, Open University, Milton Keynes, England, United Kingdom
- 4CSIRO Energy, Private Bag 10, Clayton South, VIC, Australia
Hydrogen is set to play a part in delivering a net zero emissions future globally. However, previous research finds that risk perception issues are particularly challenging for emerging and potentially unfamiliar technologies. Hydrogen as a fuel falls into this category. Thus, while the hydrogen value chain could offer a range of potential environmental, economic and social benefits, it is imperative that the roll-out of hydrogen fits with societal expectations of how risk ought to be managed—and by whom. Communication and engagement are critical to ensure 1) communities and stakeholders are able to come to informed decisions on hydrogen and 2) developers, operators and regulators are able to respond to societal concerns and adapt practices appropriately.Within the hydrogen value chain, geological storage may be an important step, but could present challenges in terms of perceived safety. Lessons can be learned from international research and practice of CO2 and natural gas storage in geological formations [for carbon capture and storage (CCS) and power respectively] which may be relevant to hydrogen storage in salt caverns or porous sandstones. We draw on these analogues to present potential societal risk perception issues which may arise for geological storage of hydrogen. We argue that site-specific communication and engagement strategies, underpinned by broad-based principles covering the entire span of the project and a clear rationale for how hydrogen benefits the climate and the most vulnerable members of society under an energy crisis, will be critical to fostering societal support for geological hydrogen storage.
1 Introduction
The use of hydrogen as a fuel substitute for electricity, heating and transport has received growing attention, as a key contributor to a low-emissions future for many countries. Within broader consideration of the place of hydrogen within an increasingly electrified and net-zero society [e.g., the UK’s Committee on Climate Change (Climate Change Committee, 2021) report on hydrogen in a low carbon economy], governments and regulators are turning attention to issues of storage and safety around hydrogen. This is happening around the globe, for example, The Council of Australian Government’s hydrogen strategy working group states “The primary consideration in delivering hydrogen is attention to safety and community awareness” (Common wealth of Australia, 2018). There is a large body of research which indicates that societal views of energy technologies such as new applications of hydrogen are not homogenous, and ought to be considered within a much wider picture of what constitutes a fair and just transition to a net-zero society (e.g., Upham, et al., 2019; Scott and Powells, 2020). Negative public views and responses can also challenge other emerging technologies, including utility-scale renewable projects such as solar farms. Siting solar farms have key technical requirements (e.g., solar resource, proximity to transmission infrastructure, slope, aspect/elevation) but may be impacted by lack of support, uncertainty, opposition, or outright resistance (Sward et al., 2021). This range of sentiment has also been seen in new nuclear and wind installations and resulting in projects being moved or terminated.
Societal responses to energy-related developments can operate at different scales. Host communities immediately adjacent to developments may have concerns relating to the physical characteristics of the development, potential risk, or perceived fairness in consultation and engagement processes. At a wider societal level (e.g., national or regional level), publics and opinion-shapers (e.g., non-governmental organisations, NGOs) may express broader concerns about the appropriateness of different energy technologies under a climate emergency, or the general direction of national energy policy. However, site-specific controversies can also become focal points for broader societal concerns about energy policy or climate responses. For instance, a small-scale exploratory shale gas study at Balcombe in the United Kingdom became the focus of protest for national NGOs, media and celebrities concerned over the potential for shale gas exploration in the United Kingdom more broadly (Hilson, 2015).
Nonetheless, a key issue driving societal support across these scales is that of trust in operators to have the competence to deploy technologies safely and securely, including—for new subsurface technologies which may enable a net-zero society—being able to understand geological complexity and work with uncertainty to avoid leakage (Gough and Boucher, 2013; Mabon and Shackley, 2015). These concerns are relevant both for communities adjacent to infrastructure, who may bear the greatest physical exposure to any risks; and also for societal actors operating at a national or regional level, who may use issues of safety to judge whether an operator and/or a technology can be trusted to operate safely and quickly enough to support net-zero targets. While acceptance of new technologies is challenging at both a community and wider societal level, it is possible to draw parallels from other relevant technologies and developments such as the implementation of carbon capture and storage (CCS) and underground (natural) gas storage (UGS). CCS and UGS industries demonstrate successes and failures that can provide lessons learned for future proponents of a hydrogen economy. As hydrogen is rarely found as a free gas in “reservoirs” like natural gas resources (Prinzhofer et al., 2018; Zgonnik, 2020; Stalker et al., 2022), other methods have been developed to isolate or generate hydrogen.
A range of countries are considering becoming hydrogen exporters, such as Australia, Norway, Brunei, and Saudi Arabia (Common wealth of Australia, 2018). Japan and South Korea have developed formal, government-led strategies for transition to hydrogen imports (from LNG and other fuels). The articulation of the Japanese strategy demonstrates large-scale enduring commitment to the uptake of hydrogen, so addressing all aspects of safety for the full value chain is becoming increasingly urgent, especially in the light of announcements by the Japanese government to not only become carbon neutral by 2050, but also commit to a 46% reduction in its greenhouse gas emissions by 2030. Similar targets have also been proposed by over 130 countries (UN, 2022). Therefore identifying, understanding and communicating the perceived risks of storage as part of hydrogen utilisation becomes a critical factor in the adoption of (or pushback against) the emergence of the use of this fuel. Here we look to technology analogues for insight into the potential societal attitudes around the development and adoption of storage as part of the hydrogen chain. Scovell (2022) in a recent review notes that a large focus of past research on the role of hydrogen as a low emissions energy technology, has mainly focused on the role of this energy vector for transport applications (e.g., passenger transport). Scovell points out the vast array of uses of hydrogen for electricity, domestic heating, manufacturing and feedstocks, and notes that understanding all the aspects of the hydrogen value chain and how they are perceived will be important for uptake and acceptance (Figure 1). For this paper we focus and draw on experience of public attitudes towards the safety of UGS and the geological disposal of CO2. The aim of this work is to highlight potential sensitivities for the hydrogen sector to consider going forward.
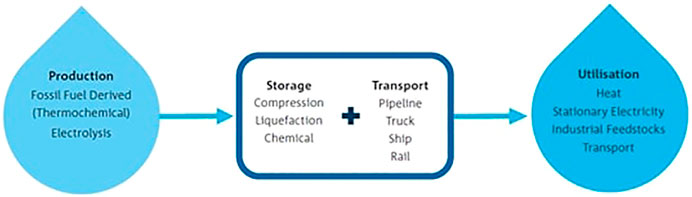
FIGURE 1. Simp2lified summary of the range of hydrogen technologies, including forms of hydrogen production, storage, transport and use (from Bruce et al., 2018). In this paper, we focus on underground hydrogen storage.
1.1 The hydrogen technology and value chain
The emerging hydrogen industry is anticipated to be a large-scale business. Goal setting for use as energy, fuel or feedstocks include 5 GW hydrogen production capacity by 2030 for the United Kingdom (Climate Change Committee, 2021). Japan’s Sixth Basic Energy Plan likewise aims to increase hydrogen supply from approximately 2 million tons per year in 2021, to up to 3 million tons/year in 2030, and 20 million tons/year by 2050 (METI, 2021).
There are a range of hydrogen technologies, including forms of hydrogen production, storage, transport, and use, each of which will have different associated risk perception issues (Figure 1). The production of hydrogen for feedstock is a long-established technology, however, the novelty of hydrogen for energy is its widespread application for greenhouse gas emissions reduction. There are different hydrogen feedstocks and processes to produce hydrogen. The most common production technology is steam reforming of natural gas, though hydrogen is also generated from coal gasification. Carbon dioxide (CO2) is a significant by-product of fossil fuel derived hydrogen but could be reduced or mitigated via CCS to reduce its carbon intensity (Volsund et al., 2016). There is potential for hydrogen generation via electrolysis using renewable energy (green hydrogen), offering hydrogen fuel with a low carbon footprint.
The temporary storage of hydrogen is likely to become a limiting factor for large scale hydrogen projects. Storage is particularly relevant where large volumes of hydrogen are being produced by electrolysis using excess renewable electricity during periods of low demand, thus requiring temporary storage to be later recovered for use or export. Small volumes of hydrogen can be stored in surface tanks, but their size is limited due to cost and safety. An overview by Barthélémy (2012) of industrial storage reinforces the limits of fabricated (e.g., large vessels) gas storage. Small volumes could also be stored in the domestic distribution pipeline networks (Panfilov, 2016). Underground geological formations, in contrast, can offer capacity to hold significant volumes of hydrogen. Two primary types of geological hydrogen stores are anticipated: salt caverns (whereby gas is injected into a series of natural or engineered small, but discrete cavities in thick salt formations), and larger-scale reservoir-caprock systems. In the latter, hydrogen is injected into a porous and permeable reservoir formation, such as a saline aquifer or a depleted hydrocarbon field, which is typically capped by an impermeable seal, not dissimilar to the characteristics of geological CO2 stores. Individual salt caverns may be small in capacity, but multiples can be used for commercial operations; four hydrogen storage projects are operational; three in the US and one plant in the United Kingdom (Zivar et al., 2021), though more are in planning. Some operations manage a large number of caverns which may have a range of different gases or materials present. Hydrogen storage in reservoir-caprock systems is more attractive owing to the larger size and scale of the potential store (Heinemann et al., 2018), but there are currently no commercial projects injecting hydrogen into porous media (Kruck, 2014; Heinemann et al., 2021).
There are similar challenges relating to surface operations of gas-filled pipelines. Transport of CO2 requires the additional management of high-pressure gas as a dense or supercritical phase. Irrespective of the type of gas, hazards relating to mechanisms, consequences and probabilities of damage or failure of pipelines can result in similar responses to risk perceptions and risk acceptability around the development of other new technologies, for example, managing large volumes of (unfamiliar) gas. Understanding these different risks can advise the appropriate development of a hydrogen economy. To this end, we consider the similarities and differences between hydrogen storage and other geoenergy technologies such as CO2 and natural gas storage to clarify where the similarities and differences between these technologies may lie (Section 2). We then review findings relevant to hydrogen storage from analogues such as advances in leakage risk perception, and communication and risk management (Section 3), and then consider what these could mean for societal and community acceptability of hydrogen deployment including storage and make recommendations for hydrogen development going forward (Section 4). However first we outline why perceived safety is such an important issue to consider at this early stage of technology development (Section 1.2) and what we know about public perceptions of hydrogen already (Section 1.3).
1.2 Why is the perceived safety of hydrogen so important?
The transition towards a zero-carbon future requires rapid and widespread technology innovation, scale-up and roll-out (Figure 2). The uptake of hydrogen depends on public acceptance of and engagement with the changes that are being implemented, which must be designed to be in line with what the publics will tolerate, accept, or support. At a community level, resistance to planning decisions has delayed or terminated a range of different energy developments and has long been recognized as a risk to deploying energy infrastructure which may form part of the low-carbon energy transition (Strachan and Lal, 2004; Van Os et al., 2014; Fast and Mabee, 2015; Temper et al., 2020). At a wider societal level, energy projects which could otherwise have been argued to support the net-zero transition have been delayed or cancelled due to national-level campaigning on broader issues such as biodiversity protection or questions over what constitutes appropriate climate action. Examples include RSPB Scotland’s opposition to offshore wind in Scotland on grounds of potential impacts on bird populations (RSPB, 2022); and the John Muir Trust’s opposition to the Beauly-Denny power line upgrade in Scotland on grounds of landscape and biodiversity impact despite the renewable energy potential the line could unlock (Tobiasson et al., 2016).
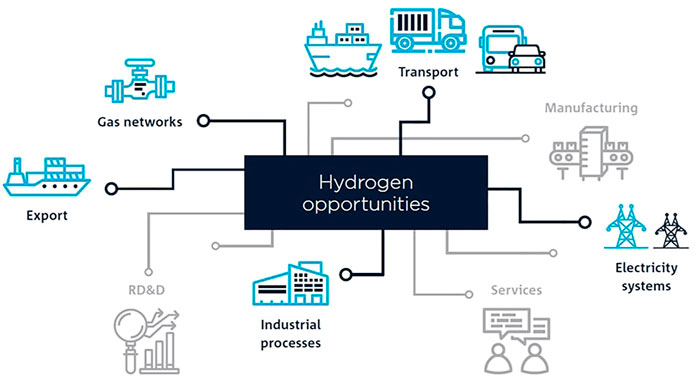
FIGURE 2. The range of role and use of hydrogen across the energy and transport sector (from Srinivasan et al., 2019).
Within this landscape, the perceived safety of the technology chain is known to be a strong component shaping public attitudes. Societal acceptability of new technologies interweaves issues of technical complexity, procedural, recogition and distributive justice, risk perception and governance of developments (Huijts et al., 2012), and depends on several factors, including perceived risk and benefits, and trust in risk management (Breakwell, 2014). As well as psychometric approaches to risk perception which emphasise perceived uncontrollability and affect from “dread factors” as explanatory factors (e.g., Alhakami and Slovic, 1994); cultural approaches to risk argue that trust in decision-makers, fit with world views and perceived fairness in decision-making processes can all shape people’s risk perceptions (Kasperson, 2014). Common across these approaches, however, is the idea that a broad range of social and cultural factors can influence what people consider to be an acceptable level of risk. Accordingly, providing more or better “evidence” alone will not necessarily lead people to believe that a risk is acceptable for a range of reasons, including perceptions of controllability (risks that are perceived to be uncontrollable are much less tolerable) and trust in the evidence-provider or other actors (Flynn et al., 2006).
Societal acceptance is particularly relevant to technologies that need public funding to support pre-commercial development (Lambert and Ashworth, 2018). In such cases, publics’ views can influence market preference and political will (in terms of the level of support for the technology). This is in addition to decision-making at the project-level, where good understanding of public attitudes can guide decisions about technology development, siting, and monitoring, and can shape community engagement processes to be fair and effective and increase the likelihood of getting support from stakeholders including local communities. Therefore, with anticipated growth of a global hydrogen market, there is a growing need to map and understand public attitudes towards hydrogen technologies at this nascent stage of technology development. This is especially important when it comes to hydrogen storage, as relatively few studies exist on public attitudes towards risks of hydrogen storage (Scovell, 2022). Much work that has been done on the societal dimensions of hydrogen has focused on hydrogen as a fuel for transportation (e.g., Itaoka et al., 2017); or on the place of hydrogen in the energy system in broader terms (e.g., Lambert and Ashworth, 2018). However, studying public perception is particularly challenging for emerging, highly technical, sensitive, uncertain or unfamiliar technologies; and exploring lay perceptions of geological hydrogen storage could be additionally confounded by the unfamiliarity of the subsurface (Ashworth et al., 2015).
1.3 What do we know about public perception of hydrogen safety?
A breadth of studies on new and unfamiliar energy technologies such as CCS have illustrated that public opinions are driven by a much broader range of factors than simple cost-benefit analyses or narrow perceptions of “safety,” both for host communities close to developments and also the wider public (e.g., Terwel et al., 2012; Mabon and Shackley, 2015). In their review of 14 studies of public perception of hydrogen, Ricci et al. (2008) noted that findings from free word association methods (used in six out of ten quantitative studies) found that hydrogen safety is not a major concern, though safety is found to be a primary concern in three of these studies and is raised as a key issue in all four qualitative studies included in their review (Ricci et al., 2008). Qualitative research found that participants also held largely positive beliefs about and attitudes towards hydrogen technology, but this positive viewpoint might be skewed by the large proportion of respondents that were undecided on this matter, and the generally very low knowledge of hydrogen as a fuel (Ricci et al., 2006). Sherry-Brennan et al. (2010) investigated host community attitudes to a hydrogen-wind project on Shetland (Scotland) using free-association methods integrated into a survey. They found hydrogen energy was generally positively evaluated despite participants being aware of and acknowledging the potential risks posed by the properties of hydrogen such as its explosiveness and flammability. In Japan, a series of more recent surveys (Itaoka et al., 2017) indicated that there was a much larger increase in awareness of hydrogen for energy use, but perception of risks and benefits remained from the previous observations discussed earlier.
A study in Australia which used mixed methods approach combining a literature review, ten focus groups (N = 92) and a nationally representative online survey (N = 2,785) Lambert and Ashworth (2018) found that the public attitudes towards hydrogen were generally neutral. The level of perceived or actual knowledge positively correlated with participants’ overall attitude to hydrogen technology, and the main perceived benefits related to the environment and include reduced greenhouse gas emissions and other pollutant emissions. Most people preferred hydrogen production from renewable sources, and there was some concern around using coal (a fossil fuel) or water (a scarce resource in Australia) as the fuel with which to generate hydrogen. A more recent review of 43 studies by Emodi et al. (2021) found that prior knowledge, perceived cost, risk and benefits were critical factors that contributed towards increased acceptance of hydrogen technologies.
Framing is known to be important in shaping public attitudes to emerging technologies (Hilson, 2015). The early generally positive attitude towards hydrogen noted in Ricci et al. (2008) was thought to be due to largely positive framing of the technology, or, conversely, an absence of negative framing. Providing negative information about the safety of hydrogen was found to significantly reduce acceptance, whereas the effect of positive information was marginal (see Ricci et al., 2008; Bögel et al., 2018). A study of how attitudes influence technology acceptance by Bögel and co-authors (2018) noted that this was limited by lack of knowledge and awareness of the technology in question. Their study on the use of hydrogen fuel cells in Europe (they surveyed nationally-representative samples of approximately 1,000 adults from 7 countries in Europe) found that information campaigns would have maximum effect at the early stage of new technology introduction in the sphere of hydrogen. The attitude of individuals with respect to technology, climate change, fuel cells and their specific applications were compared, and it was noted that prior attitudes had a strong influence on how new information on hydrogen was perceived (Bögel et al., 2018). In cases where there were positive attitudes, these were easily eroded and lacked intensity.
Trust—or more correctly, distrust—was also identified as a key driver of public beliefs, attitudes and expectations (Ricci et al., 2008). In Australia, where public attitudes are largely positive, the majority (77%) of Australian publics trusted that adequate safety precautions would keep any risks under control. As noted previously, few studies have explored public attitudes to hydrogen storage. Lambert and Ashworth (2018) report that in focus group discussions some participants expressed concerns about the use of carbon capture and storage as part of the hydrogen chain, largely owing to the perceived environmental risks posed by geological CO2 storage. The study does not report whether these participants expressed similar concern for hydrogen geological storage, but there are some concerns with hydrogen being stored underground, with only 42% supporting this approach (Lambert and Ashworth, 2018). While publics may have a neutral or even positive attitude towards hydrogen, given the limited—and mixed—empirical literature on public views on hydrogen storage, we turn to analogous technologies for insight.
2 Analogues for the geological storage of hydrogen
The geological storage of CO2 and natural gas (UGS) and compressed air energy storage (CAES) have all been argued to be suitable analogues (Itaoka et al., 2017) (Table 1). However, we do not consider CAES in this work because the technology is in very early development, like hydrogen storage. Demonstration projects that do exist for CAES at present are small-scale and within the grounds of research facilities, and hence are not close to communities or in the general public domain. As such, the kinds of CAES facilities that currently exist are unlikely to be understood, let alone a source of concern, for publics; and may offer limited insight to managing risks associated with storage from a technical perspective. This is different to CO2 storage and natural gas, where there have been medium-to large-scale demonstrators (e.g., Tomakomai in Japan, Ketzin in Germany), commercial-scale CCS operations of millions of tonnes per annum (e.g., Gorgon in Australia, Boundary Dam in Canada or Sleipner in Norway) and a long history of storage (natural gas) in the lived environment, and hence examples of deployment that lessons for hydrogen can be drawn from.
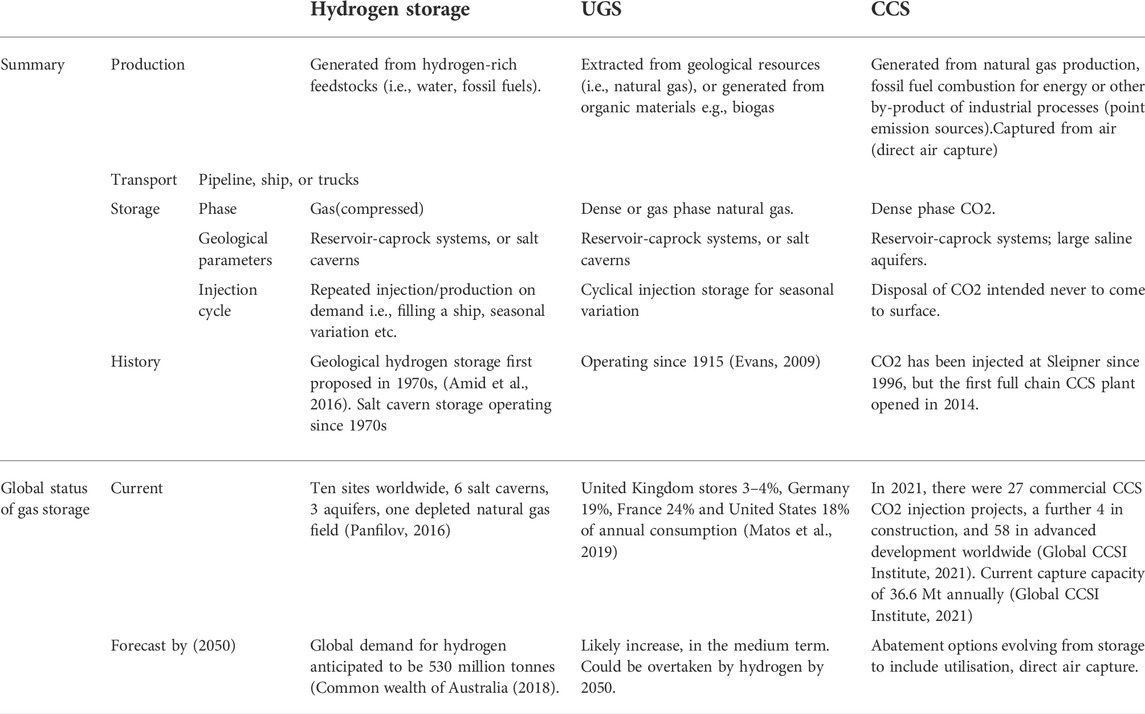
TABLE 1. A comparison of the CO2, hydrogen and natural gas geological storage process and the current and projected development of these technologies.
2.1 Carbon capture and storage and natural gas storage analogues
CCS includes geological storage of CO2 that is intended to be permanent. Globally, there are currently 27 active commercial-scale CCS projects (Global CCSI Institute, 2021; Table 1). While gas processing and handling (i.e., akin to capture), pipeline transport of gases and geological assessment of the subsurface for volumes of hydrocarbons in place (i.e., carbon geological storage) are all well understood, joining these pieces up together for CCS is still regarded as somewhat of an emerging technology. However, all the components of the CCS value chain have been technically feasible for decades, and there is a reasonably lengthy history of activity. The first CO2 injection project (unconnected to enhanced oil recovery (EOR) processes) is Sleipner. The Sleipner CCS Project is located in the Norwegian North Sea and started injecting CO2 for storage in 1996. However, it was 18 more years until the first fully integrated CCS project Boundary Dam in Canada commenced CO2 injection (Preston et al., 2018). Geological storage of CO2 is fundamental to the delivery of net zero emissions by 2050 (Alcalde et al., 2018), but many challenges have hindered CCS development. These have tended to be economic and financial rather than technical or procedural and had up until recently related to a lack of economic viability (Alcalde et al., 2018). The design and development of hub and cluster models such as Northern Lights, Acorn, or Net Zero Teeside have reframed the technoeconomics (e.g., Alcade et al., 2019).
UGS refers to temporary, cyclic, storage of methane. UGS is a well-established technology that has been used as an economical method for managing gas delivery for over 90 years. In total 630 facilities were in operation in 2009 (Evans, 2009) with 317 active commercial projects in the US in 2017 (Michanowicz et al., 2017). UGS presently occurs in salt or rock caverns, depleted hydrocarbon reservoirs or abandoned mines, and saline aquifers. UGS is deemed to have excellent health, safety and environmental record (Evans, 2009), however in recent years there have been some incidences of gas leakage in the US, including the well failure at the Aliso Canyon gas storage facility in Los Angeles in 2015 (Pan et al., 2018). The paper by Pan et al. (2018) notes that the researchers had previously developed coupled well-reservoir simulators for modelling behaviour of CO2 leakage in wells, which was then adapted for methane leakage. The modelling software was then able to predict possible outcomes from remediation and kill procedures to mitigate the leakage of large amounts of methane from the UGS facility, demonstrating the benefits of considering analogues.
Key properties and behaviours of each of these gas storage types, summarized in Table 1, illustrate how comparable these industries are.
2.2 Comparing analogues
Reservoirs for hydrogen and UGS tend to be shallower than CO2 storage [>800 m to maintain supercritical CO2 phase (Chadwick et al., 2008)]. Hydrogen storage depth is shallower (∼200 m below surface at typical geothermal gradient; Heinemann et al., 2018). For all gas storage technologies, the surface footprint of the geological store is small, and most visual impacts, if any, would be related to the well head or monitoring of the store.
A primary concern for all forms of geological storage is leakage. While the most likely potential leakage pathways of CO2, natural gas and hydrogen may be similar (poorly sealed wells, un-imaged faults etc., (Chadwick et al., 2008), the impacts of leakage will be different owing to the properties of the three gases. While hydrogen might be more mobile in the subsurface relative to natural gas and CO2, other processes, such as increasingly tortuous pathways for smaller molecules (Carrigan et al., 1996) could have counterintuitive influences. At surface, light gases like hydrogen readily disperse, whereas CO2 ponds in depressions due to its greater density. Understanding the fate of hydrogen that might leak to surface especially given its larger range of flammability introduces different and potentially greater risks to that of methane and CO2 (Table 2). Further points of similarity and difference between hydrogen and other gases can be found in Schmidtchen (2009).
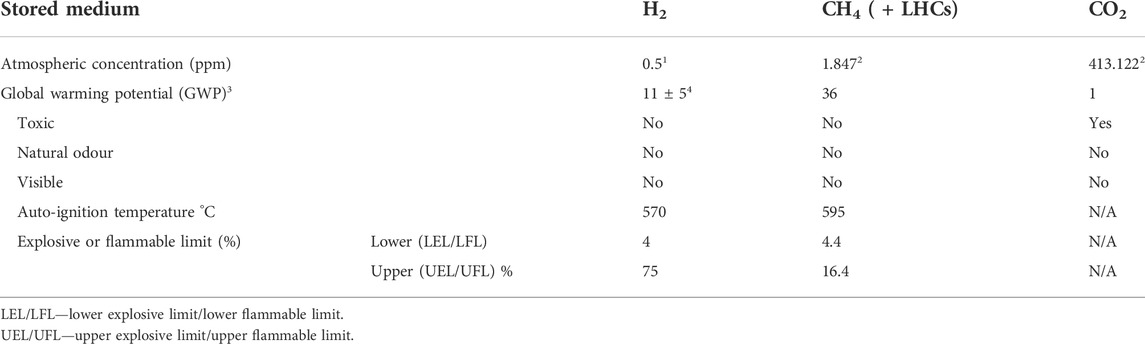
Table 2. Summary of the different properties of H2, CH4, and CO2. Natural gas is normally a blend of light hydrocarbons (LHCs) but is predominantly CH4.1Pidwirny (2012), 2Cape Grim Data (2022); 3IPCC (2013); 4Warwick et al. (2022).
3 The perceived risks of underground storage
As illustrated in Section 2, UGS offers insights into the management of risks related to gas storage from a technical perspective, which can help to identify potential risk perception and management issues that could arise for hydrogen. However, there are very few modern academic studies specific to the social perception of risks associated with UGS. This likely reflects that UGS is a widespread and widely accepted energy technology, and therefore has been in little need of research around public perceptions, even following a series of events in the US where several thousand people were displaced from their homes over several months following the Aliso Canyon underground gas storage well blowout and subsequent kill operations (Pan et al., 2018). As such, much of this section focuses on public perception of leakage from CO2 stores. We return to UGS in Section 4, where we draw out potential risk issues for hydrogen storage identified in our analogues and reflect on with this means for societal risk perception and engagement on risk for hydrogen storage.
3.1 Perceived risk of gas leakage
Whilst it is true that a wide range of factors shape public perceptions of new subsurface energy technologies, L׳Orange Seigo et al. (2014) report that the most commonly held concern about CCS is that the injected CO2 might leak, how this leakage might occur, and the resultant environmental impact. As CO2 is regarded as a pollutant, it also has associations with toxicity. Publics can express fear of over-pressurization and may believe there might be sudden blowouts or explosions at the surface or deep underground, or that CO2 injection might cause earthquakes, which might compromise storage integrity (L’Orange Seigo et al., 2014). Shackley and Gough (2006) found that leakage in the style of the well-known Lake Nyos disaster from 1986 was commonly articulated or raised as a potential impact of CO2 leakage. However, the perceived risk of CCS is also negatively related to the trust in stakeholders (Terwel and Daamen, 2012) and in acceptability of the technology (Wallquist et al., 2012). Public perception of risk is reduced if monitoring approaches are trusted (Terwel and Daamen, 2012), and detailed monitoring is deemed to be beneficial in terms of public acceptability of the technology and trust in the regulation (Feitz et al., 2014; Waarum et al., 2017). Conversely, in the case of Tomakomai, Japan, Mabon et al. (2017) found that fishers’ initial apprehensions towards sub-seabed CO2 storage were driven in part by previous negative experiences with poorly managed industrial activities in the bay. Both at the community and wider societal levels, therefore, although safety and storage integrity concerns are often discussed by publics and stakeholders, these discussions are heavily influenced by issues of trust and perceived competence of operators and regulators.
Public support for CCS is dependent upon the acknowledgement that climate change is real and must be mitigated (Mabon and Shackley, 2015). However, publics and stakeholders may also view CCS as an unsustainable or undesirable mitigation strategy, even if they acknowledge the reality of climate change and trust in the safety of the technology. CO2 storage space is finite, and the technology is associated with power stations and heavy industry. CCS has been critiqued for its potential to allow fossil fuel energy operators to continue environmentally harmful practices such as oil recovery and hydrogen production from methane without fully deploying CCS to capture associated emissions. This may become especially problematic if CCS is perceived as diverting attention and research and development effort away from renewable energy sources (Mabon and Shackley, 2015); or comes to be viewed as “subsidising” fossil fuel industries through the use of public funds to support operators to implement CCS projects (Stephens, 2013). In short, public and opinion-shaper support for CCS—which is critical to sustain support from policy makers and legislators—may depend not only on the perceived safety of the technology, but on a much wider suite of factors relating to the political economy of who ought to benefit from the deployment of net-zero technologies (Markusson et al., 2020).
Scepticism towards CCS may hence be strong at the national scale. Yet at a local level if storage sites are located close to current or recent subsurface activity and/or onshore energy infrastructure, then communities may be more familiar with subsurface processes and less concerned by the technical or political-economic risks outlined above. Operators involved in CCS activities are likely to have prior experience of working in a locality through, for example, extracting oil or gas from reservoirs that are now considered storage sites, or running power stations/refineries that are suitable for CO2 capture (Alcade et al., 2019). It may thus be the case that operators come to be viewed as good and trusted employers with a long history of managing their operations safely and bringing employment and economic benefit to host communities. Whilst it should not be assumed that this means a social licence to operate can be transferred to CCS activities, good relations established during prior activity may well help to maintain community support for new developments such as CCS (Mabon et al., 2017; Gough et al., 2018).
3.2 Conceptualizing leakage
For many, geoenergy technologies such as CCS can appear largely “imaginary” (Reiner, 2015). This may be because the scale is difficult to envisage, the projects are far from centres of population, the surface footprint of storage activities is comparatively small, or the technology is still at the conceptual stage. Similarly, research into the subsurface more broadly has found that publics without in-depth or specialised knowledge can find the nature of the subsurface hard to conceptualise (Gibson et al., 2016). In turn, this difficulty in conceptualising the subsurface can lead to concerns that because CO2 is a gas it must inevitably rise to and leak out at the surface. Indeed, there is much room for misconceptions around leakage, and leak impact, particularly where there are misinterpretations of the technicalities of the process. For example, if it is perceived that CO2 is stored in the form of “a large bubble” of gas in the rocks, it could be understood that the bubble could burst at any time, and so the perceived risk is deemed to be high (Shackley et al., 2004). Further, given that the most publicised forms of leakage from UGS are uncontrolled blowouts, it is reasonable for publics to assume that leakage of hydrogen or CO2 from geological stores might occur in a similar dramatic manner.
3.3 Communicating leakage
The need for effective engagement and communication around CCS, and the role of such communication in risk perception and management, is well recognised and can offer insights for hydrogen storage. CCS communication and engagement has been the subject of extensive study, especially as some projects have been terminated due to societal concerns (e.g., Ashworth et al., 2010; Van Os et al., 2014; Xenias and Whitmarsh, 2018). These, and other studies provide a gateway into planning and consideration for the emergence of hydrogen technology use and deployment. Reflecting our points above about the breadth of factors that can shape societal attitudes to CCS and other novel uses of the subsurface, it is vital that engagement and communication does not tend towards ‘information deficit’ models where the end goal is to lead host communities and wider society to “accept” new energy or emissions-related technologies. It is also important that engagement and communication respects the breadth of issues that may be of concern to publics, rather than closing down engagement to narrow discussions on risk and safety. Nonetheless, for technologies like CCS, UGS, and hydrogen where public understanding is low and real-world examples may (in the case of CCS and hydrogen) be limited (Reiner, 2015; Perdan et al., 2017), it is true that some level of communication and engagement may be required to enable communities, less-informed stakeholders and publics to come to an informed decision on whether or not they deem these technologies to be appropriate.
Several studies have found that as public participants gained more information about CCS, group discussions became increasingly complicated, and the opinions of participants negatively affected (e.g., Upham and Roberts, 2011). However, more recent work from Dowd et al. (2014) suggests that such problems could arise because of flaws in the assumed public knowledge of CO2. They found that providing information on the scientific characteristics of CO2 reduces the potential for misunderstanding of CCS and prevents the degree of negative opinion change. This may directly relate to the lack of flammability or explosive behaviour of CO2 relative to other gases such as hydrogen (Table 2).
Significant efforts have been undertaken to develop materials that have helped to inform a range of stakeholders about CCS concepts (Ashworth et al., 2015). Community engagement has been conducted—and evaluated—for CO2 storage-related activities across a range of scales. One example of this is the QICS (Quantifying and Monitoring Potential Ecosystem Impacts of CO2 Storage) project, which released CO2 from below the seabed into a bay in the west of Scotland under experimental conditions, to assess potential ecosystem impacts of leakage from storage site (Mabon et al., 2015). Another is the Tomakomai CCS Demonstration project in Japan, which injected 300,000 tonnes of CO2 into subsea geological formations via a subsea pipeline from an onshore capture plant to demonstrate the viability of CCS technologies in Hokkaido, northern Japan (Mabon et al., 2017). The peer-reviewed research into both of these examples illustrates the importance of early and prolonged engagement and of projects being able to adapt their communication and engagement approaches in response to what communities and stakeholders say they want to know. While the emerging CCS industry can provide case studies and potential roadmaps for community engagement, there are mixed results reported in the literature regarding the effect that information and tools used by these communication programmes has had on risk perceptions (L’Orange Seigo et al., 2014).
Perceived risk could be informed by imagery. It is a well-known proverb that “a picture speaks a thousand words,” however, with problems of scale, conventional visualisations of the CCS process generally present overly simplified and highly schematic depictions of the geological subsurface (Feenstra, 2012). The role of the subsurface geology, and its depiction, is a key part in not only CCS but also in underground hydrogen (and gas) storage. In 2010, Wallquist et al., 2010 and co-authors noted that “CCS communication should focus on information and images that quickly help nonexperts improve their understanding and avoid information and images that might only increase risk perception without resulting in a better understanding of CCS.” Some of these visuals will include imagery and communication materials from pilot and commercial scale CCS projects, and also from research, which may be employed to illustrate impact and risk (or lack thereof). Indeed, natural analogues for CO2 leakage are widespread, and have been studied around the globe to understand the surface expression of leakage. There is hence a case for making these images and data more widely available to enhance communication of leakage risk (Roberts and Stalker, 2017; Stalker et al., 2018). At the same time, however, the ability of publics to quickly grasp complex issues and to engage in-depth with questions of risk and uncertainty should not be underestimated. Graphics that appear over-simplified, or indeed engagement approaches that are viewed as patronising or overly confident in the capabilities of CCS, may have the effect of reducing public support for CCS if they create the impression the presenter is trying to convince the public of the technology (Howell et al., 2014). These are important lessons to be considered as part of the development of a hydrogen industry.
4 Translating these learnings to hydrogen storage
We have presented learnings about the perceived risks around gas leakage from CO2 storage and from UGS and experiences of communicating these risks, to identify potential risk perception issues that may arise in relation to the geological storage of hydrogen. We now consider the differences between CO2, natural gas, and hydrogen storage which could limit how translatable or applicable these learnings are, and then we suggest key messages for the hydrogen sector, as a first step towards managing the social acceptability of hydrogen geological storage.
4.1 How similar might the perceived risks be?
CO2, natural gas and hydrogen storage follows the same principles—the storage of gas in geological formations. Hydrogen and natural gas are a valuable commodity, while CO2 has long been considered as a pollutant, a waste product and a cost. However, this perception is in transition as more countries declare their net zero targets and their related mechanisms for crediting emissions avoided. If hydrogen is used for domestic power, publics will be familiar with the gas and its use. The fact that underground hydrogen storage offers temporary storage of a useful resource, and not a form of waste disposal (i.e., intended to remain in the subsurface for geological timescales) may make hydrogen storage more acceptable to publics than CCS. However, there may be nuance in perception depending on the nature of the hydrogen produced. ‘Green’ hydrogen (i.e., hydrogen produced through renewable energy) will likely be viewed more favourably than hydrogen derived from fossil fuels, given that this will reduce the CO2 emissions associated with hydrogen production and remove the need for CCS, which is viewed by more sceptical voices as unproven or unfeasible (e.g., Toke, 2020). This reflects results of research conducted for CCS in the context of CO2-Enhanced Oil Recovery, which found that public and opinion-shapers perceived storage less positively when it was viewed as enabling an activity regarded as harmful to the climate (Mabon and Littlecott, 2016). Indeed, reflecting CCS research e.g., Stephens. (2013) and Mabon and Shackley. (2015), evidence from Scotland suggests that NGOs view hydrogen production as allowing fossil fuel operators to give the impression of taking positive climate action and hence perpetuating harmful activities elsewhere (Dixon, 2019). In 2020, Friends of the Earth Scotland branded hydrogen as a “false solution” to climate change (Friends of the Earth, 2020). Similarly, empirical research has questioned the necessity of hydrogen in the UK’s heating goals, and positioned the technology as being pushed by a coalition of incumbent actors including the gas industry (Lowes et al., 2020). At a national level, it may thus be the case that public and stakeholder perceptions of hydrogen storage are driven not only by views on safety concerns, but on the necessity and propriety of hydrogen in a net-zero energy system more broadly.
The gases we consider here for geological storage gases (CO2, H2, and CH4) are all odourless and not visible, and so are difficult to detect with the human senses, but only CO2 classifies as toxic (Table 2). Humans tend to be more fearful of unseen or undetectable compounds such as gases. Public awareness of the properties of these gases (i.e., whether or not you can smell or see them) is generally quite limited (Upham and Roberts, 2011). This low level of knowledge in itself means that it is difficult to predict perception of a hydrogen leak in comparison to CO2 or natural gas, since the lay public are likely not to know, for example, that hydrogen disperses more readily than CO2. Nonetheless, initial research (Mouli-Castillo et al., 2021) indicates that people can identify escaping odorised hydrogen at regulatory thresholds.
As for leakage from geological formations, hydrogen is a smaller and lighter molecule than natural gas and CO2 and could buoyantly leak more readily (Heinemann et al., 2018). But the small size of hydrogen molecules could lead to more tortuous pathways through the subsurface thus taking longer to travel. While CO2 is injected for permanent disposal; hydrogen will have a comparatively short residence time in the subsurface formation, reducing likelihood of significant leakage (Heinemann et al., 2018). That said, from a technical perspective, UGS and hydrogen storage could be regarded as more complex than CO2 storage. The repeated injection and production cycles associated with these technologies and the resulting variable subsurface pressure (i.e., dynamic geomechanical impacts) pose enhanced uncertainty regarding the rock behaviour, and therefore the sealing capability of the different rock formations to prevent leakage over time (Heinemann et al., 2021). Understanding deliverability (i.e., cycles of injection and withdrawal) and impacts from the interplay of water drainage and replacement by gas, types of cushion gas, buffering and repressurisation are complex and still subjects of research into performance and financial viability of UHS (Muhammed et al., 2022). However, these geological complexities are unlikely to be a major influence on public perspectives of leakage risk (Ricci et al., 2006). Rather, the same issues around how publics conceptualise the subsurface i.e., one of the present challenges for CCS, will likely be the same set of challenges for hydrogen and many other georesources.
Another learning to consider concerns monitoring and measurement. Since both methane and hydrogen are present at much lower concentrations in our atmosphere, they may be easier to detect above background, which has implications for ease of detection and monitoring. These two gases might therefore be easier to monitor for and measure than CO2, where variations in background concentration due to natural processes can be problematic (Blackford et al., 2015). Reflecting on the points raised in Section 3, however, trust in the institution providing the data to “tell the truth” and manage a storage site competently is likely to have a significant influence on whether communities and stakeholders engage with and trust the outputs of monitoring and measurement. The ease of monitorability may alter risk perception. There are other learnings beyond leakage risk to be translated between the technologies. For example, there may be parallels with regards to the political narrative or messaging regarding CO2 storage and hydrogen. In the United Kingdom, Turner et al. (2019) indicates that the political narrative of presenting CCS as part of a climate mitigation strategy (i.e., reducing CO2 emissions), rather than as part of an industrial or business strategy (i.e., creating jobs, stimulating the economy), has affected how CCS has been received by policymakers, businesses and publics. Swennenhuis et al. (2020) similarly suggest that presenting CCS within a narrative of a just transition for workers and communities reliant on carbon-intensive industries may build support, a strategy that could be explored for hydrogen too.
4.2 Messages for the hydrogen storage sector
A nebula of issues surrounds societal and community acceptability of new technologies. What is critical to note is that issues of perceived risk are often closely linked to questions of trust, efficacy, and fairness. Understanding these issues, and what hydrogen developers, regulators and policymakers can do to allay, adapt, or address concerns, will be fundamental to the roll out of hydrogen storage and the wider hydrogen economy. Applying learnings from geological storage of CO2, we propose the following recommendations:
• Early engagement: Successful projects have early and high-quality engagement programmes to open channels for dialogue with communities and stakeholders proximate to proposed developments; and also with key opinion-shapers (e.g., NGOs, media) at a national or regional level. Such dialogue builds trust and deepens understanding of both publics’ and developers’ understandings, concerns and proposed responses. This dialogue improves understanding of the project context, including familiarity with energy or resource industries. What is especially important, however, is that engagement is not “closed down” to a narrow focus on technical factors, and that industry and governments are able to demonstrate they are able to make tangible changes to projects (or at least to engagement strategies) in response to societal concerns;
• Address the unknown: New technologies or changes, such as using hydrogen for distributed power, generating hydrogen from renewable-powered electrolysis, or storing hydrogen in rocks underground are relatively new and unfamiliar. It is important that hydrogen stakeholders do not assume a level of knowledge from non-expert stakeholders, as misunderstandings can propagate, become problematic and difficult to reverse. Conversely, it should also be remembered that non-experts can quickly understand and engage with complex issues relating to energy and the subsurface. Engagement and communication that is perceived as overly simplified or patronising may be viewed with suspicion;
• Complex concepts: New, complex or unfamiliar concepts can affect perceived risks. For example, risk of gas leakage or conceptualising the subsurface is typically unfamiliar and therefore challenging for non-specialists to conceptualise. Such topics should be identified early so as to enable the design of effective communication tools and approaches. Again, however, the dangers of over-simplifying communication tools or of trying to “over-sell” a technology should be borne in mind. It may instead be more effective to aim to empower communities, stakeholders and wider publics with the knowledge they need to come to their own informed standpoint on the merits or otherwise of hydrogen;
• Material evidence: There is value in demonstrator projects: whether these are field trials, pilot scale projects or commercial scale operations. They generate evidence. And without evidence, beliefs may be built on own experience or on rumour (Flynn et al., 2006). Further, to quote Reiner (2015):710 “…it is difficult to engage in a serious public debate over risks or to develop an effective risk communications strategy if there is no actual project on which to present information.” Demonstrator projects can also help to overcome the scepticism that subsurface technologies are unviable or cannot reach deployment stage (Gonzalez et al., 2021);
• Trust in operators and regulators: As for any emerging technology or any new development, a project will be affected by trust in the governing institutions. Risks become more acceptable if there is the perception that regulation will protect, monitoring will be robust, and that operators will be genuinely operating to maximise safety. Experiences from analogous technologies suggest that these perceptions will vary with scale and context, for example having a demonstrable track record. Under a climate emergency and a cost-of-living crisis, the sincerity in the motivations of operators from the energy sector in particular is likely to come under intense scrutiny from NGOs, the media and pressure groups operating at a national or regional level. This is especially so if projects are supported in part by public funds;
• Blue, green or black: Public attitudes may be more positive or hostile depending on the hydrogen source. The underground storage of hydrogen generated from renewables (green hydrogen) or biofuels will likely be much more widely acceptable than the storage of hydrogen generated from fossil fuels (black hydrogen) or fossil fuels with CCS (blue hydrogen). Clarity on the hydrogen source involved in projects, and ideally a clear pathway towards the least carbon-intensive forms of hydrogen, may hence help to build support and demonstrate the climate benefits of a project;
• Impacts and Benefits: Societal and community perception will depend on the perceived negative and positive impacts, both to the host community and to the region or country more broadly. These might be in terms of economic or social gains, or environmental fit. For example, research on the public acceptance of CCS finds that support for the technology is dependent upon the acknowledgement that climate change is real and must be mitigated. It is very likely that this will also apply to the public acceptability of underground hydrogen storage, and the hydrogen economy more generally. More generally, lack of local benefit from investment in infrastructure such as CCS and hydrogen will challenge societal and community acceptability, especially for localities that have been heavily reliant on fossil fuel jobs and who will expect to see local employment and financial benefits as part of a just transition. Examining developments through a social justice lens will reduce risk of developments hindering a just transition to net zero.
4.3 Limitations of technocentric analogues
We have focused in this paper on technocentric analogues for the geological storage of hydrogen, especially CCS and UGS. There are, however, limitations to what can be understood from these analogues. Firstly, as noted by Esteves et al. (2012), the operational and closure phases are an equally important part of community engagement with developments. The lack of large-scale CCS projects (not related to fossil fuel production) that have reached operational yet alone completion stage, and the limited body of literature on the societal aspects of later stages of UGS, mean that there is only limited insight from these analogues as to the broader issues that geological storage of hydrogen projects may face once they are up and running. Indeed, one could argue that the CCS literature in particular has been skewed towards “acceptance” of new CCS projects, and not enough towards what happens once projects are in operation.
Second, although we have previously suggested that a history of an operator working on subsurface energy issues in a locality may make it more likely for communities to engage with new subsurface developments, it must not be assumed that this will always be the case. A social licence to operate cannot directly be “transferred” from one development to another, even if there may be links (Gough et al., 2018). This is especially so in the context of an energy crisis and a climate emergency. Oil, gas or coal operations will likely have commenced several decades previous, when community and civil society concerns over climate change were much less visible than they are today. Therefore, it should not be assumed that because an analogous fossil fuel development was able to proceed in the past, that there will be similar support for developments such as hydrogen storage that are happening against much more vocal calls for a just transition and rapid move away from fossil fuels (see e.g., Swennenhuis et al., 2020).
5 Conclusion
The review of existing scholarship around CCS and UGS illustrates the importance of early and thorough consideration of societal issues prior to deployment of hydrogen storage in support of a potential future hydrogen economy. Risk perception is of course just one factor among many driving societal views towards new and potentially unfamiliar technologies such as geological hydrogen storage. Moreover, risk perception has also been shown to be influenced by a wide range of contextual factors relating to society and culture. Experiences with CCS show the importance of considering new technologies in context, and of understanding how place, history and prior experience can drive risk perception. There is a concomitant need to develop communication resources for stakeholder, community, and general public engagement on hydrogen storage, underpinned by rigorous and context-specific research and an acknowledgement that communities and stakeholders will expect their own knowledges and concerns to be respected within the engagement and decision-making process. Moreover, experiences with CCS also illustrate there can be a difference between considering the technology in the abstract versus a “real-world” project in a specific locale. In this regard, pilot projects offer a valuable opportunity to understand what informs societal responses in practice, and to generate evidence of new technology. Once projects commence, it is also important to remember that stakeholders and communities will require ongoing engagement and monitoring data, hence there is value in considering now questions such as the relative ease of monitorability of hydrogen leakage. Whilst it is too early to pinpoint specific issues which may arise for societal support of underground hydrogen storage, it is fair to say that site-specific communication and engagement strategies, underpinned by broad-based principles covering the entire span of the project and a clear rationale for how hydrogen benefits the climate and the most vulnerable members of society under an energy crisis, will aid the likelihood of underground hydrogen storage gaining societal acceptance, as well as the broader expansion of the hydrogen economy.
Data availability statement
This work is a synthesis of research experience and from the intersection of geological experience and research into social license to operate. It has not used specific data sets or generated data.
Author contributions
All authors participated in the development and design of the paper. LS led the writing, JR and LM provided additional material and references on the social aspects. PH provided information and materials on the context of the hydrogen industry and access to the figures.
Funding
LS, PH are employees of CSIRO. LM is an employee of Open University JR is a Chancellors Fellow at U. Strathclyde
Acknowledgments
We thank the University of Strathclyde and the Sir David Anderson Bequest Award for supporting Roberts’ research visit to CSIRO to pursue this work. LS and PH thank CSIRO for the opportunity to conduct this research, while LJM acknowledges support from the EPSRC HyStorPor project (EP/S027815/1).
Conflict of interest
The authors declare that the research was conducted in the absence of any commercial or financial relationships that could be construed as a potential conflict of interest.
Publisher’s note
All claims expressed in this article are solely those of the authors and do not necessarily represent those of their affiliated organizations, or those of the publisher, the editors and the reviewers. Any product that may be evaluated in this article, or claim that may be made by its manufacturer, is not guaranteed or endorsed by the publisher.
References
Alcalde, J., Flude, S., Wilkinson, M., Johnson, G., Edlmann, K., Bond, C. E., et al. (2018). Estimating geological CO2 storage security to deliver on climate mitigation. Nat. Commun. 9, 2201. doi:10.1038/s41467-018-04423-1
Alcalde, J., Heinemann, N., Mabon, L., Worden, R. H., de Coninck, H., Robertson, H., et al. (2019). Acorn: Developing full-chain industrial carbon capture and storage in a resource- and infrastructure-rich hydrocarbon province. J. Clean. Prod. 233, 963–971. doi:10.1016/j.jclepro.2019.06.087
Alhakami, A. S., and Slovic, P. (1994). A psychological study of the inverse relationship between perceived risk and perceived benefit. Risk Anal. 14 (6), 1085–1096. doi:10.1111/j.1539-6924.1994.tb00080.x
Amid, A., Mignard, D., and Wilkinson, M. (2016). Seasonal storage of hydrogen in a depleted natural gas reservoir. Int. J. Hydrogen Energy 41, 5549–5558. doi:10.1016/j.ijhydene.2016.02.036
Ashworth, P., Boughen, N., Mayhew, M., and Millar, F. (2010). From research to action: Now we have to move on CCS communication. Int. J. Greenh. Gas Control 4 (2), 426–433. doi:10.1016/j.ijggc.2009.10.012
Ashworth, P., Wade, S., Reiner, D., and Liang, X. (2015). Developments in public communications on CCS. Int. J. Greenh. Gas Control 40, 449–458. doi:10.1016/j.ijggc.2015.06.002
Barthélémy, H. (2012). Hydrogen storage - industrial prospectives. Int. J. Hydrogen Energy 37, 17364–17372. doi:10.1016/j.ijhydene.2012.04.121
Blackford, J., Bull, J. M., Cevatoglu, M., Connelly, D., Hauton, C., James, R. H., et al. (2015). Marine baseline and monitoring strategies for carbon dioxide capture and storage (CCS). Int. J. Greenh. Gas Control 38, 221–229. doi:10.1016/j.ijggc.2014.10.004
Bögel, P., Oltra, C., Sala, R., Lores, M., Upham, P., DütschkeSchneider, U., et al. (2018). The role of attitudes in technology acceptance management: Reflections on the case of hydrogen fuel cells in Europe. J. Clean. Prod. 188, 125–135. doi:10.1016/j.jclepro.2018.03.266
Bruce, S., Temminghoff, M., Hayward, J., Schmidt, E., Munnings, C., Palfreyman, D., et al. (2018). National hydrogen roadmap. Australia: CSIRO.
Cape Grim Data (2022). Csiro. AvaliableAt: https://www.csiro.au/en/Research/OandA/Areas/Assessing-our-climate/Latest-greenhouse-gas-data Data from December 2021 (accessed 2 3, 2022).
Carrigan, C. R., Heinle, R. A., Hudson, G. B., Nitao, J. J., and Zucca, J. J. (1996). Trace gas emissions on geological faults as indicators of underground nuclear testing. Nature 382, 528–531. doi:10.1038/382528a0
Chadwick, A., Arts, R., Bernstone, C., May, F., and ThibeauZweigel, S. P. (2008). Best practice for the storage of CO2 in saline aquifers. Report. Keyworth: British Geological Survey Occasional Publication.
Climate Change Committee (2021). Independent assessment: The UK’s net zero strategy. AvaliableAt: https://www.theccc.org.uk/publication/independent-assessment-the-uks-net-zero-strategy/ (accessed 511, 2021).
Common wealth of Australia (2018). Hydrogen for Australia’s future A briefing paper for the coag energy Council prepared by the hydrogen strategy group August 2018. Available at: https://www.chiefscientist.gov.au/2018/08/briefing-paper-hydrogen-for-australias-future/ (accessed 11 5, 2021).
Dixon, T. (2019). Why hydrogen is NOT the fuel of the future’ the Scotsman Online Edition. Available at: https://www.scotsman.com/lifestyle/travel/why-hydrogen-not-fuel-future-dr-richard-dixon-1406371 (accessed 02 04, 2022).
Dowd, A. M., Itaoka, K., Ashworth, P., Saito, A., and de Best-Waldhober, M. (2014). Investigating the link between knowledge and perception of CO 2 and CCS: An international study. Int. J. Greenh. Gas Control 28, 79–87. doi:10.1016/j.ijggc.2014.06.009
Emodi, N. V., Lovell, H., Levitt, C., and Franklin, E. (2021). A systematic literature review of societal acceptance and stakeholders’ perception of hydrogen technologies. Int. J. Hydrogen Energy 46, 30669–30697. doi:10.1016/j.ijhydene.2021.06.212
Esteves, A. M., Franks, D., and Vanclay, F. (2012). Social impact assessment: the state of the art. Impact Assess. Proj. Apprais. 30 (1), 34–42. doi:10.1080/14615517.2012.660356
Evans, D. J. (2009). A review of underground fuel storage events and putting risk into perspective with other areas of the energy supply chain. Geol. Soc. Lond. Spec. Publ. 313 (1), 173–216. doi:10.1144/sp313.12
Fast, S., and Mabee, W. (2015). Place-making and trust-building: The influence of policy on host community responses to wind farms. Energy Policy 81, 27–37. doi:10.1016/j.enpol.2015.02.008
Feenstra, Y. (2012). Visuals? Better none than wrong. Available at: http://www.globalccsinstitute.com/insights/authors/feenstra/2012/03/15/visuals-betternone-Wrong.
Feitz, A. J., Leamon, G., Jenkins, C., Jones, D. G., Moreira, A., Bressan, L., et al. (2014). Looking for leakage or monitoring for public assurance? Energy Procedia 63, 3881–3890. doi:10.1016/j.egypro.2014.11.418
Flynn, R., Bellaby, P., and Ricci, M. (2006). Risk perception of an emergent technology: The case of hydrogen energy. Forum Qual. Sozialforschung / Forum Qual. Soc. Res. 7 (1), 19. doi:10.17169/fqs-7.1.58
Friends of the Earth (2020). Twitter. Available at: https://twitter.com/FoEScot/status/1319564597238616065?s=20 last (accessed 06 27, 2022).
Gibson, H., Stewart, I. S., Pahl, S., and Stokes, A. (2016). A "mental models" approach to the communication of subsurface hydrology and hazards. Hydrol. Earth Syst. Sci. 20 (5), 1737–1749. doi:10.5194/hess-20-1737-2016
Global CCSI Institute (2021). The global status of CCS 2021; CCS accelerating to net zero. Melbourne, Australia: Global CCS Institute.
Gonzalez, A., Mabon, L., and Agarwal, A. (2021). Who wants North Sea CCS, and why? Assessing differences in opinion between oil and gas industry respondents and wider energy and environmental stakeholders. Int. J. Greenh. Gas Control 106, 103288. doi:10.1016/j.ijggc.2021.103288
Gough, C., and Boucher, P. (2013). Ethical attitudes to underground CO2 storage: Points of convergence and potential faultlines. Int. J. Greenh. Gas Control 13, 156–167. doi:10.1016/j.ijggc.2012.12.005
Gough, C., Cunningham, R., and Mander, S. (2018). Understanding key elements in establishing a social license for CCS: An empirical approach. Int. J. Greenh. Gas Control 68, 16–25. doi:10.1016/j.ijggc.2017.11.003
Heinemann, N., Alcalde, J., Miocic, J. M., Hangx, S. J. T., Kallmeyer, J., Ostertag-Henning, C., et al. (2021). Enabling large-scale hydrogen storage in porous media - the scientific challenges. Energy Environ. Sci. 14, 853–864. doi:10.1039/d0ee03536j
Heinemann, N., Booth, M. G., Haszeldine, R. S., Wilkinson, M., Scafidi, J., Edlmann, K., et al. (2018). Hydrogen storage in porous geological formations - onshore play opportunities in the midland valley (Scotland, UK). Int. J. Hydrogen Energy 43, 20861–20874. doi:10.1016/j.ijhydene.2018.09.149
Hilson, C. (2015). Framing fracking: Which frames are heard in English planning and environmental policy and practice? J. Environ. Law 27 (2), 177–202. doi:10.1093/jel/equ036
Howell, R., Shackley, S., Mabon, L., Ashworth, P., and Jeanneret, T. (2014). Engaging the public with low-carbon energy technologies: Results from a scottish large group process. Energy Policy 66, 496–506. doi:10.1016/j.enpol.2013.11.041
Huijts, N. M. A., Molin, E. J. E., and Steg, L. (2012). Psychological factors influencing sustainable energy technology acceptance: A review-based comprehensive framework. Renew. Sustain. Energy Rev. 16, 525–531. doi:10.1016/j.rser.2011.08.018
IPCC (2013). in Climate change 2013: The physical science basis. Contribution of working group I to the fifth assessment report of the intergovernmental panel on climate change. Editors T. F. Stocker, D. Qin, G.-K. Plattner, M. Tignor, S. K. Allen, J. Boschunget al. (Cambridge, United Kingdom and New York, NY, USA: Cambridge University Press), 1535.
Itaoka, K., Saito, A., and Sasaki, K. (2017). Public perception on hydrogen infrastructure in Japan: Influence of rollout of commercial fuel cell vehicles. Int. J. Hydrogen Energy 42, 7290–7296. doi:10.1016/j.ijhydene.2016.10.123
Kasperson, R. (2014). Four questions for risk communication. J. Risk Res. 17 (10), 1233–1239. doi:10.1080/13669877.2014.900207
Kruck, O. (2014). Assessment of the potential, the actors and relevant business cases for large scale and seasonal storage of renewable electricity by hydrogen underground storage in Europe. AvaliableAt: http://hyunder.eu/wp-content/uploads/2016/01/D3.1_Overview-of-all-known-underground-storage-technologies.pdf.
Lambert, V., and Ashworth, P. (2018). The Australian public’s perception of hydrogen for energy. Report. Canberra: Australian Renewable Energy Agency.
Lowes, R., Woodman, B., and Speirs, J. (2020). Heating in Great Britain: An incumbent discourse coalition resists an electrifying future. Environ. Innovation Soc. Transitions 37, 1–17. doi:10.1016/j.eist.2020.07.007
L׳Orange Seigo, S., Dohle, S., and Siegrist, M. (2014). Public perception of carbon capture and storage (CCS): A review. Renew. Sustain. Energy Rev. 38, 848–863. doi:10.1016/j.rser.2014.07.017
Mabon, L., Kita, J., and Xue, Z. (2017). Challenges for social impact assessment in coastal regions: A case study of the Tomakomai CCS demonstration project. Mar. Policy 83, 243–251. doi:10.1016/j.marpol.2017.06.015
Mabon, L., and Shackley, S. (2015). Meeting the targets or Re-imagining society? An empirical study into the ethical landscape of carbon dioxide capture and storage in Scotland. Environ. values 24, 465–482. doi:10.3197/096327115x14345368709907
Mabon, L., and Littlecott, C. (2016). Stakeholder and public perceptions of CO 2 -EOR in the context of CCS - results from UK focus groups and implications for policy. Int. J. Greenh. Gas Control 49, 128–137. doi:10.1016/j.ijggc.2016.02.031
Mabon, L., Shackley, S., Blackford, J. C., Stahl, H., and Miller, A. (2015). Local perceptions of the QICS experimental offshore CO 2 release: Results from social science research. Int. J. Greenh. Gas Control 38, 18–25. doi:10.1016/j.ijggc.2014.10.022
Markusson, N., Balta-Ozkan, N., Chilvers, J., Healey, P., Reiner, D., McLaren, D., et al. (2020). Social science sequestered. Front. Clim. 2, 2. doi:10.3389/fclim.2020.00002
Matos, C. R., Carneiro, J. F., and Silva, P. P. (2019). Overview of large-scale underground energy storage technologies for integration of renewable energies and criteria for reservoir identification. J. Energy Storage 21, 241–258. doi:10.1016/j.est.2018.11.023
Michanowicz, D. R., Buonocore, J. J., Rowland, S. T., Konschnik, K. E., Goho, S. A., Bernstein, A. S., et al. (2017). A national assessment of underground natural gas storage: Identifying wells with designs likely vulnerable to a single-point-of-failure. Environ. Res. Lett. 12, 064004. doi:10.1088/1748-9326/aa7030
Mouli-Castillo, J., Orr, G., Thomas, J., Hardy, N., Crowther, M., Haszeldine, R. S., et al. (2021). A comparative study of odorants for gas escape detection of natural gas and hydrogen. Int. J. Hydrogen Energy 46 (27), 14881–14893. doi:10.1016/j.ijhydene.2021.01.211
Muhammed, N. S., Haq, B., Al Shehri, D., Al-Ahmed, A., Rahman, M. M., Zaman, E., et al. (2022). A review on underground hydrogen storage: Insight into geological sites, influencing factors and future outlook. Energy Rep. 8, 461–499. doi:10.1016/j.egyr.2021.12.002
Pan, L., Oldenburg, C. M., Freifeld, B. M., and Jordan, P. D. (2018). Modeling the Aliso Canyon underground gas storage well blowout and kill operations using the coupled well-reservoir simulator T2Well. J. Petroleum Sci. Eng. 161, 158–174. doi:10.1016/j.petrol.2017.11.066
Panfilov, M. (2016). “Underground and pipeline hydrogen storage,” in Hydrogen storage, transportation and infrastructure (Sawston: Woodhead Publishing), 91–115. doi:10.1016/b978-1-78242-362-1.00004-3
Perdan, S., Jones, C. R., and Azapagic, A. (2017). Public awareness and acceptance of carbon capture and utilisation in the UK. Sustain. Prod. Consum. 10, 74–84. doi:10.1016/j.spc.2017.01.001
Pidwirny, M. (2012). “Atmospheric Composition: Carbon Dioxide,” in Fundamentals of Physical Geography eBook. 2nd Edition. Kelowna: University of British Columbia. Available At: http://www.physicalgeography.net/fundamentals/7aCO2.html
Preston, C., Bruce, C., and Monea, M. (2018). “An update on the integrated CCS project at SaskPower’s boundary Dam power station,” in 14th Greenhouse Gas Control Technologies Conference, October 21-26 (Melbourne: GHGT-14).
Prinzhofer, A., Tahara Cissé, C. S. T., and Diallo, A. B. (2018). Discovery of a large accumulation of natural hydrogen in Bourakebougou (Mali). Int. J. Hydrogen Energy 43, 19315–19326. doi:10.1016/j.ijhydene.2018.08.193
Reiner, D. M. (2015). Where can I go to see one? Risk communications for an 'imaginary technology'. J. Risk Res. 18 (6), 710–713. doi:10.1080/13669877.2015.1040657
Ricci, M., Bellaby, P., and Flynn, R. (2008). What do we know about public perceptions and acceptance of hydrogen? A critical review and new case study evidence. Int. J. Hydrogen Energy 33 (21), 5868–5880. doi:10.1016/j.ijhydene.2008.07.106
Ricci, M., Newsholme, G., Bellaby, P., and Flynn, R. (2006). Hydrogen: Too dangerous to base our future upon? Institution Chem. Eng. Symposium Ser. 151, 42.
Roberts, J. J., and Stalker, L. (2017). What have we learned about CO2 leakage from field injection tests? Energy Procedia 114, 5711–5731. doi:10.1016/j.egypro.2017.03.1710
RSPB (2022). media-centre. AvaliableAt: https://www.rspb.org.uk/about-the-rspb/about-us/media-centre/press-releases/scotwind-response/ accessed14/06/2022.
Schmidtchen, U. (2009). “Fuels - SAFETY | hydrogen: Overview,” in Encyclopaedia of electrochemical power sources (Elsevier), 519–527. doi:10.1016/b978-044452745-5.00842-x
Scott, M., and Powells, G. (2020). Towards a new social science research agenda for hydrogen transitions: Social practices, energy justice, and place attachment. Energy Res. Soc. Sci. 61, 101346. doi:10.1016/j.erss.2019.101346
Scovell, M. D. (2022). Explaining hydrogen energy technology acceptance: A critical review. Int. J. Hydrogen Energy 47, 10441–10459. doi:10.1016/j.ijhydene.2022.01.099
Shackley, S., and Gough, C. (2006). Carbon capture and its storage: An integrated assessment. Aldershot: Ashgate.
Shackley, S., McLachlan, C., and Gough, C. (2004). The public perception of carbon dioxide capture and storage in the UK: Results from focus groups and a survey. Clim. Policy 4, 377–398. doi:10.3763/cpol.2004.042810.1080/14693062.2004.9685532
Sherry-Brennan, F., Devine-Wright, H., and Devine-Wright, P. (2010). Public understanding of hydrogen energy: A theoretical approach. Energy Policy 38 (10), 5311–5319. doi:10.1016/j.enpol.2009.03.037
Srinivasan, V., Temminghoff, M., Charnock, S., and Hartley, P. (2019). Hydrogen research, development and demonstration: Priorities and opportunities for Australia. CSIRO.
Stalker, L., Roberts, J. J., and Mabon, L. (2018). Bursting bubbles: Can experiments and analogues help stakeholders and the public visualise risks? APPEA J. 58 (2), 612. doi:10.1071/aj17091
Stalker, L., Talukder, A., Strand, J., Josh, M., and Faiz, M. (2022). Gold (hydrogen) rush: Risks and uncertainties in exploring for naturally occurring hydrogen. Appea J. 62 (1), 361–380. doi:10.1071/aj21130
Stephens, J. C. (2013). Time to stop investing in carbon capture and storage and reduce government subsidies of fossil‐fuels. WIREs Clim. Change 5, 169–173. doi:10.1002/wcc.266
Strachan, P. A., and Lal, D. (2004). Wind energy policy, planning and management practice in the UK: Hot air or a gathering storm? Reg. Stud. 38 (5), 549–569. doi:10.1080/0143116042000229311
Sward, J. A., Nilson, R. S., Katkar, V. V., Stedman, R. C., Kay, D. L., Ifft, J. E., et al. (2021). Integrating social considerations in multicriteria decision analysis for utility-scale solar photovoltaic siting. Appl. Energy 288, 116543. doi:10.1016/j.apenergy.2021.116543
Swennenhuis, F., Mabon, L., Flach, T. A., and de Coninck, H. (2020). What role for CCS in delivering just transitions? An evaluation in the North Sea region. Int. J. Greenh. Gas Control 94, 102903. doi:10.1016/j.ijggc.2019.102903
Temper, L., Avila, S., Bene, D., Gobby, J., Kosoy, N., Billon, P., et al. (2020). Movements shaping climate futures: A systematic mapping of protests against fossil fuel and low-carbon energy projects. Environ. Res. Lett. 15, 123004. doi:10.1088/1748-9326/abc197
Terwel, B. W., and Daamen, D. D. L. (2012). Initial public reactions to carbon capture and storage (CCS): Differentiating general and local views. Clim. Policy 12, 288–300. doi:10.1080/14693062.2011.637819
Terwel, B. W., ter Mors, E., and Daamen, D. D. L. (2012). It's not only about safety: Beliefs and attitudes of 811 local residents regarding a CCS project in Barendrecht. Int. J. Greenh. Gas Control 9, 41–51. doi:10.1016/j.ijggc.2012.02.017
Tobiasson, W., Beestermöller, C., and Jamasb, T. (2016). Public engagement in electricity network development: The case of the beauly-denny project in Scotland. Econ. Polit. Ind. 43, 105–126. doi:10.1007/s40812-016-0030-0
Toke, D. (2020). The UK Government may be encouraging greenwash in its announcement on ‘hydrogen ready’ boilers. AvaliableAt: https://www.gshp.org.uk/Hydrogen_Greenwash.html (accessed 02 4, 2022).
Turner, K., Alabi, O., Low, R., and Race, J. (2019). Reframing the value case for CCUS: Evidence on the economic value case for CCUS in Scotland and the UK. (Technical Report). Glasgow: University of Strathclyde. doi:10.17868/67391
UN (2022). Net zero coalition. AvaliableAt: https://www.un.org/en/climatechange/net-zero-coalition (accessed 02 3, 2022).
Upham, P., Bögel, P., and Johansen, K. (2019). Energy transitions and social psychology: A sociotechnical perspective. London: Routledge.
Upham, P., and Roberts, T. (2011). Public perceptions of CCS in context: Results of NearCO2 focus groups in the UK, Belgium, The Netherlands, Germany, Spain and Poland. Energy Procedia 4, 6338–6344. doi:10.1016/j.egypro.2011.02.650
van Os, H. W. A., Herber, R., and Scholtens, B. (2014). Not under our back yards? A case study of social acceptance of the northern Netherlands CCS initiative. Renew. Sustain. Energy Rev. 30, 923–942. doi:10.1016/j.rser.2013.11.037
Voldsund, M., Jordal, K., and Anantharaman, R. (2016). Hydrogen production with CO2 capture. Int. J. hydrogen energy 41, 4969–4992. doi:10.1016/j.ijhydene.2016.01.009
Waarum, I.-K., Blomberg, A. E. A., Eek, E., Brown, J., Ulfsnes, A., Carpenter, M., et al. (2017). CCS leakage detection technology - industry needs, government regulations, and sensor performance. Energy Procedia 114, 3613–3627. doi:10.1016/j.egypro.2017.03.1493
Wallquist, L., Seigo, S. L. O., Visschers, V. H. M., and Siegrist, M. (2012). Public acceptance of CCS system elements: A conjoint measurement. Int. J. Greenh. Gas Control 6, 77–83. doi:10.1016/j.ijggc.2011.11.008
Wallquist, L., Visschers, V. H. M., and Siegrist, M. (2010). Impact of knowledge and misconceptions on benefit and risk perception of CCS. Environ. Sci. Technol. 44, 6557–6562. doi:10.1021/es1005412
Warwick, N., Griffiths, P., Keeble, J., Archibald, A., and Pyle, J. (2022). Atmospheric implications of increased hydrogen use. AvaliableAt: https://assets.publishing.service.gov.uk/government/uploads/system/uploads/attachment_data/file/1067144/atmospheric-implications-of-increased-hydrogen-use.pdf pp 75.
Xenias, D., and Whitmarsh, L. (2018). Carbon capture and storage (CCS) experts' attitudes to and experience with public engagement. Int. J. Greenh. Gas Control 78, 103–116. doi:10.1016/j.ijggc.2018.07.030
Zgonnik, V. (2020). The occurrence and geoscience of natural hydrogen: A comprehensive review. Earth-Science Rev. 203, 103140. doi:10.1016/j.earscirev.2020.103140
Keywords: geological carbon storage, energy transition, societal acceptance, underground storage, hydrogen safety
Citation: Stalker L, Roberts JJ, Mabon L and Hartley PG (2022) Communicating leakage risk in the hydrogen economy: Lessons already learned from geoenergy industries. Front. Energy Res. 10:869264. doi: 10.3389/fenrg.2022.869264
Received: 04 February 2022; Accepted: 07 July 2022;
Published: 10 August 2022.
Edited by:
Quan Xie, Curtin University, AustraliaReviewed by:
Emily Cox, Cardiff University, United KingdomFanhe Kong, Georgia Institute of Technology, United States
Copyright © 2022 Stalker, Roberts, Mabon and Hartley. This is an open-access article distributed under the terms of the Creative Commons Attribution License (CC BY). The use, distribution or reproduction in other forums is permitted, provided the original author(s) and the copyright owner(s) are credited and that the original publication in this journal is cited, in accordance with accepted academic practice. No use, distribution or reproduction is permitted which does not comply with these terms.
*Correspondence: Linda Stalker, bGluZGEuc3RhbGtlckBjc2lyby5hdQ==