- 1Department of Materials and Life Sciences, Sophia University, Tokyo, Japan
- 2Basic Research Center, Nippon Chemi-Con Corporation, Kanagawa, Japan
Recently, ionic plastic crystals (IPCs) have been actively investigated to develop all-solid-state rechargeable batteries, such as lithium-ion batteries. Herein, we report supercapacitors assembled with mesoporous carbon electrodes and an IPC electrolyte, N-ethyl-N-methylpyrrolidinium bis(fluorosulfonyl)amide ([C2mpyr][FSA]). [C2mpyr][FSA] with a 10 mol% lithium bis(fluorosulfonyl)amide (LiFSA) dopant was used as the solid electrolyte in the supercapacitors. The charge–discharge tests of the supercapacitors were performed at various C-rates in the voltage range of 0–2.5 V at 25°C. The capacitance of the cells was 12.3 Fg−1 at a lower C rate (1 C = 8.9 mA g−1). The capacitance retention of the supercapacitors was maintained at approximately 100% up to 20 C, which was comparable to that of the cells containing organic electrolyte solutions. The advantage of using solid electrolytes was the fabrication of bipolar cells using two pairs of mesoporous carbon electrodes and a [C2mpyr][FSA]/LiFSA composite. The charge–discharge tests of the bipolar cells were also performed in the voltage range of 0–5.0 V at 25°C. The capacitance of the bipolar cells was 6.4 Fg−1 at a lower C rate. The bipolar cells exhibited a typical charge–discharge profile for 1,000 cycles, confirming their stable cyclic performance. Thus, IPC electrolytes are interesting materials for developing all-solid-state high-voltage supercapacitors.
Introduction
An electrochemical capacitor, also known as a supercapacitor, is a device that stores electric energy by forming an electric double layer at the electrode/electrolyte interface (Pandolfo and Hollenkamp, 2006;Simon and Gogotsi, 2008). Supercapacitors utilize the non-Faradaic process of charging and discharging the electric double layer and are generally characterized by high power density and a long cycle life, which are highly desirable for many applications, such as hybrid electric vehicles (Miller and Burke, 2008). Therefore, supercapacitors are considered one of the most important electrochemical storage devices in addition to other storage devices, such as Li-ion batteries, fuel cells, and solar cells. However, problems associated with the usage of liquid electrolytes, such as flammability and electrolyte leakage, require improvements. Nevertheless, these issues can be addressed by replacing liquid electrolytes with solid electrolytes. Furthermore, solid electrolytes make the devices thin and flexible. Although gel polymer electrolytes have been actively studied as solid electrolytes for supercapacitors (Naoi and Morita, 2008;Cheng et al., 2018), they are composed of a polymer matrix, solvent, and salt, and the leakage of liquid electrolytes may occur.
Plastic crystals (PCs) are solid materials with regular long-range crystal lattices, possessing local orientational or rotational disorders with respect to molecular or ionic species. Molecules in their PC phase have a higher degree of freedom than those in the normal crystalline state, exhibiting high plasticity and diffusivity of constituents. Ionic plastic crystals (ICPs) are soft solids with plasticity. These materials have characteristics different from those of conventional solid electrolytes; hence, research in this field has become more active. Remarkably, Cooper et al. and MacFarlane et al. previously reported that IPCs containing ammonium and pyrrolidinium salts acted as Li-ion conductors (Cooper and Angell, 1986;MacFarlane et al., 1999). In addition to lithium-ion conductors, IPCs have recently attracted attention as sodium-ion (Chimdi et al., 2015;Makhlooghiazad et al., 2018), proton (Yoshizawa-Fujita et al., 2007;Yoshizawa-Fujita et al., 2022), and iodide ion conductors (Armel et al., 2011). Furthermore, IPCs are attractive materials because the chemical and physical properties of a compound can be altered by changing the combination of cations and anions that comprise IPCs, enabling the development of IPC-based solid electrolytes suitable for various electrochemical devices. The potential use of IPCs in electrochemical devices has also been demonstrated for rechargeable batteries, fuel cells, and solar cells (Pringle, 2013;Zhu et al., 2019).
A previous study reported the development of a supercapacitor using N,N-dimethylpyrrolidnium fluorohydroganate ([C1mpyr][(FH)2F]), an IPC, as a solid electrolyte (Taniki et al., 2014). The ionic conductivity of [C1mpyr][(FH)2F] is 1.03 × 10–2 S cm−1 at 25°C, which is comparable to that of ionic liquids. In addition, the charge–discharge tests of a supercapacitor with combined [C1mpyr][(FH)2F] and activated carbon electrodes showed that the capacitances of the positive and negative electrodes after 300 cycles were 263 and 221 Fg−1, respectively. Furthermore, the alternating current (AC) impedance measurement results suggested the formation of an electric double layer between the electrolyte and the surface of the pores of the activated carbon, demonstrating that the supercapacitor using IPC operated stably by charging and discharging the supercapacitor. This study demonstrated the effective replacement of liquid electrolytes with solid electrolytes using IPCs as a solid electrolyte for electrochemical capacitors. However, the anion of [C1mpyr][(FH)2F] contains hydrogen fluoride and requires specific care during its usage.
In our previous study, we showed that IPCs based on pyrrolidinium cations and bis(fluorosulfonyl)amide (FSA) anions exhibited relatively high ionic conductivities above 10–5 S cm−1 at 25°C when a small amount of Li salt was added to the IPCs as the dopant (Yamada et al., 2019). Furthermore, we demonstrated that Li batteries using N-ethyl-N-methylpyrrolidinium bis(fluorosulfonyl)amide ([C2mpyr][FSA]) with a small amount of a LiFSA dopant as solid electrolytes successfully operated at 50°C (Wang et al., 2017;Zhou et al., 2017). These results impelled us to develop supercapacitors with [C2mpyr][FSA] as a solid electrolyte. It has been reported that [C2mpyr][FSA] exhibited solid–solid phase transitions at −70 and −22°C and a high melting point of 205°C (Yoshizawa-Fujita et al., 2014). Zhou et al. also investigated the phase transition behaviors of [C2mpyr][FSA] with various Li salt contents (Zhou et al., 2017). Minimal changes in the solid–solid phase transition temperatures were observed with the addition of 10 mol% LiFSA, while the melting point decreased to 140 °C. Although the solid–solid phase transitions were distinct at 20 mol% LiFSA, the melting point could not be distinguished in the differential scanning calorimetry curves, suggesting that the composite was a mixture of solid and liquid phases. In this study, [C2mpyr][FSA] doped with 10 mol% LiFSA was prepared as a solid electrolyte and further used with mesoporous carbon electrodes to fabricate all-solid-state supercapacitors. Additionally, we investigated the electrochemical properties and performance of the cells with the electrolytes and electrodes mentioned above.
Experimental
Materials
N-Methylpyrrolidine (98%) was procured from Tokyo Chemical Industry Co. Ltd., (Chuo-ku, Tokyo, Japan), while iodoethane (>98.0%) and acetonitrile (AN, 99.8%) were procured from FUJIFILM Wako Pure Chemical Corporation (Chuo-ku, Osaka, Japan). The reagents were purified via distillation before use. Lithium bis(fluorosulfonyl)amide (LiFSA, 99%) was procured from Kishida Chemical Co., Ltd. (Chuo-ku, Osaka, Japan). Dimethyl carbonate (DMC) (99.9%) and a triethylmethylammonium tetrafluoroborate (TEMABF4)/propylene carbonate (PC) solution (1.8 M) were procured from Tomiyama Pure Chemical Industries, Ltd. (Chuo-ku, Tokyo, Japan). Mesoporous carbon was procured from TOYO TANSO Co., Ltd. (Nishiyodogawa-ku, Osaka, Japan). The vapour-grown carbon fibre (VGCF®) was procured from Showa Denko K.K. (Minato-ku, Tokyo, Japan). Poly(vinylidene difluoride) (PVDF) was procured from Kureha Corporation (Chuo-ku, Tokyo, Japan). All solvents were purchased from Kanto Chemical Co., Inc. (Chuo-ku, Tokyo, Japan), or FUJIFILM Wako Pure Chemical Corporation (Chuo-ku, Osaka, Japan) and purified by appropriate means, if necessary.
N-Ethyl-N-methylpyrrolidinium bis(fluorosulfonyl)amide ([C2mpyr][FSA]) was prepared following a previously reported method (Yoshizawa-Fujita et al., 2014). The [C2mpyr][FSA]/LiFSA (10 mol%) composite was prepared by mixing [C2mpyr][FSA] and LiFSA at approximately 25°C in dichloromethane. After mixing for 1 h, the [C2mpyr][FSA]/LiFSA composite was dried in a vacuum at 40°C for 2 days.
Fabrication of Supercapacitor Cells
Figure 1A shows the fabrication process of the supercapacitor cells. Mesoporous carbon, VGCF®, and PVDF were mixed at 100:9:10 (by weight ratio) in N-methylpyrrolidone (NMP), and the composite solution was cast on an aluminium foil. The coated aluminium foils were dried at 80°C for 1 h and pressed at 3 kN cm−1. The carbon electrodes were cut into discs (area: 1.0 cm2). The loaded mass of the electrode active material was 0.9 mg cm−2. The [C2mpyr][FSA]/LiFSA composite was dissolved in DMC or AN, and a 10 wt% solution was prepared. Each solution was cast on the carbon electrodes to fill the pores of the electrodes, which were then dried in a vacuum at 80 °C for 12 h. Subsequently, the carbon electrodes and a separator filled with the [C2mpyr][FSA]/LiFSA composite were assembled and dried in a vacuum at 120°C for 12 h. Laminate cells were prepared in an Ar-filled glove box (H2O < 1 ppm, O2 < 1 ppm) to minimize moisture contamination. Furthermore, a bipolar cell was fabricated in this study to develop all-solid-state high-voltage supercapacitors. Figure 1B illustrates a schematic of the bipolar cell fabricated using two pairs of mesoporous carbon electrodes and [C2mpyr][FSA]/LiFSA.
Measurements
The cross-section milling of the electrodes was performed using an ion milling system (IM4000PLUS, Hitachi High-Tech (Minato-ku, Tokyo, Japan)). The cross section of the electrodes filled with the [C2mpyr][FSA]/LiFSA composite was observed using scanning electron microscopy (SEM; S-5500, Hitachi High-Tech (Minato-ku, Tokyo, Japan)).
Complex impedance measurements were performed between 10 and 1 MHz (applied voltage: 10 mV) using an impedance analyser (VMP3, BioLogic (France)) at 25°C. The temperature was controlled using a constant-temperature oven (MC-811T, Espec Corp. (Kita-ku, Osaka, Japan)). The charge–discharge tests were conducted using a battery charge–discharge system (TOYO SYSTEM Co., Ltd. (Fukushima, Japan)).
Results and Discussion
Observation of Electrodes
The pores of the electrodes must be filled with electrolytes to achieve high performance for the charge/discharge of supercapacitors. Hence, two kinds of solvents (AN and DMC) were used to investigate the effect of the solvent species on the pore filling of the electrodes. These solvents were selected because they can dissolve IPCs and have a relatively low boiling point. Figure 2 shows the SEM images of the cross section of the electrodes filled with the [C2mpyr][FSA]/LiFSA composite when AN was used as the solvent. The SEM images at any magnification in the range of 2000- (Figure 2A), 10000- (Figure 2B), and 50000-fold (Figure 2C) showed that the electrode pores were thoroughly filled with the electrolyte. However, when DMC was used as the solvent, many voids were observed in the pores of the electrode, even at low magnification in the SEM image (Supplementary Figure S1). A significant difference was observed between the AN and DMC solutions. The boiling points of AN and DMC were 82 and 90°C, respectively, and were almost similar. This difference is based on the solvent properties (for example, viscosity and the dielectric constant). Thus, the best result was obtained in the AN solution.
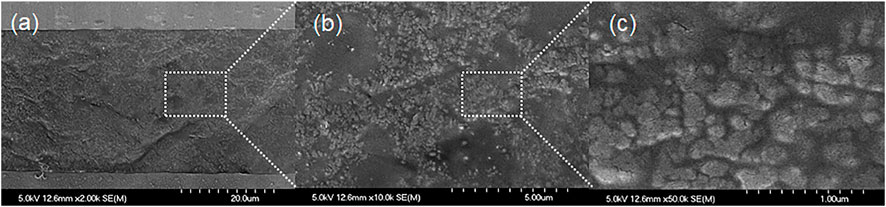
FIGURE 2. SEM images of the cross-section of the electrodes filled with the [C2mpyr][FSA]/LiFSA composite when acetonitrile was used as a solvent. (A) 2000-, (B) 10000-, and (C) 50000-fold.
Charge–Discharge Tests
The charge–discharge tests of the supercapacitors assembled with [C2mpyr][FSA]/LiFSA were performed at various C-rates (1 C = 8.9 mA g−1), where the supercapacitors were charged up to 2.5 V at 25°C. A TEMABF4/PC solution (1.8 M) was used as the electrolyte for comparison. Conventional supercapacitors employ solvent-based electrolytes to achieve high ionic conductivity, even at sub-zero temperatures. A combination of onium salts and organic solvents has been mainly utilised in supercapacitors (Ue, 1994b;Ue et al., 1997;Xu et al., 2001). In this study, the capacitance of the supercapacitors was 12.3 Fg−1 at a lower C rate. Figure 3A shows the C-rate dependence of capacitance for the supercapacitors assembled with TEMABF4/PC and [C2mpyr][FSA]/LiFSA at 25°C. The capacitance retention of the TEMABF4/PC system was maintained at 100% up to approximately 20 C, after which capacitance gradually decreased with an increasing C rate. In contrast, the [C2mpyr][FSA]/LiFSA system exhibited almost similar C-rate dependency as that of the TEMABF4/PC system up to 500 C. The capacitance of the [C2mpyr][FSA]/LiFSA system was above 10 Fg−1, even at 500 C. This C-rate dependency is linked to the difference in ionic conductivity between the two electrolytes. The ionic conductivity of the [C2mpyr][FSA]/LiFSA composite is 7 × 10–6 S cm−1 at 20°C (Zhou et al., 2017), which is much lower than that of the TEMABF4/PC solution (>10–2 S cm−1 at 25°C) (Ue, 1994a).
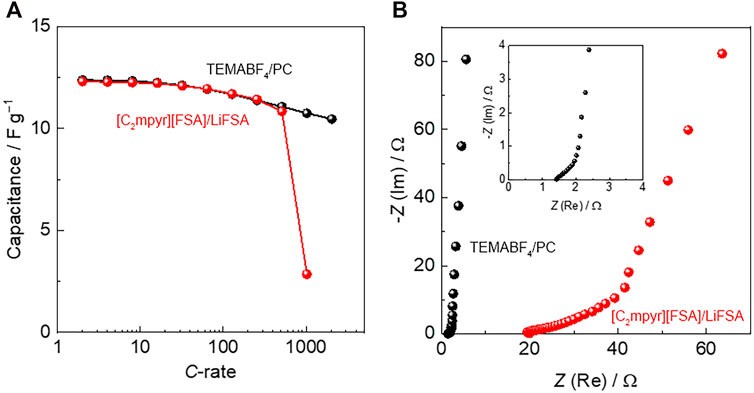
FIGURE 3. (A) C-rate dependence of capacitance for supercapacitors assembled with TEMABF4/PC and [C2mpyr][FSA]/LiFSA, and (B) Nyquist plots of AC impedance spectra for supercapacitors assembled with TEMABF4/PC and [C2mpyr][FSA]/LiFSA. The inset image shows the high-frequency region for TEMABF4/PC.
Figure 3B shows the Nyquist plots of the AC impedance spectra for the supercapacitors assembled with TEMABF4/PC and [C2mpyr][FSA]/LiFSA at 25°C. The measurements were conducted before the charge–discharge tests, and the inset shows the high-frequency region of TEMABF4/PC. The Nyquist plots were different for both systems. The resistances of the supercapacitors at a high frequency were 1.4 and 20 Ω for the TEMABF4/PC and [C2mpyr][FSA]/LiFSA systems, respectively. These values roughly reflect the ionic conductivities of the two electrolytes. The supercapacitors assembled with TEMABF4/PC and [C2mpyr][FSA]/LiFSA behaved as pure capacitance at low frequencies, characterised by a vertical line parallel to the imaginary axis. However, the vertical line was shifted largely along the real axis toward more resistive values at medium frequencies for the supercapacitor assembled with [C2mpyr][FSA]/LiFSA. This tendency is associated with a difference in the electrolyte penetration inside the micropores of the electrode (Taberna et al., 2003;Itagaki et al., 2007).
The charge–discharge tests evaluated the cyclic performance of the supercapacitors at a constant rate of 16 C, where the supercapacitors were charged up to 2.5 V at 25°C, as shown in Figure 4. During 1,200 charge–discharge tests, capacitance retention was maintained at approximately 100% for both electrolytes, and no decrease in capacitance was observed. The stable cyclic performance of the supercapacitors was confirmed during the test. The results indicate that [C2mpyr][FSA]/LiFSA performs as a stable solid-state electrolyte in the supercapacitors with mesoporous carbon electrodes in the voltage range of 0–2.5 V.
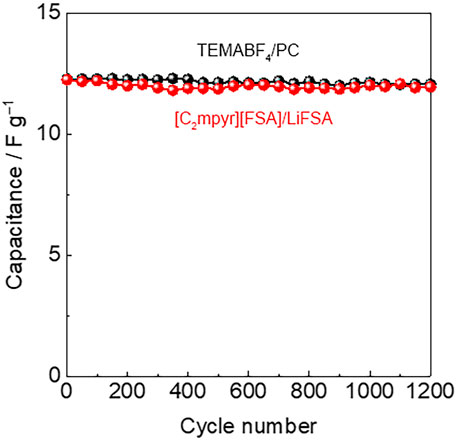
FIGURE 4. Cyclic performance of supercapacitors evaluated using charge–discharge tests at a constant rate of 16 C in the voltage range of 0–2.5 V.
Bipolar Cell
The advantage of solid-state electrolytes is the fabrication of bipolar cells. In addition, it has been reported that N,N-diethylpyrrolidinium bis(fluorosulfonyl)amide ([C2epyr][FSA]) doped with 5 mol% LiFSA, a similar composite of [C2mpyr][FSA]/LiFSA, exhibits high electrochemical stability with an electrochemical window of 5.4 V vs. Li/Li+ (Yamada et al., 2019). These results are related to the development of all-solid-state high-voltage supercapacitors. A bipolar cell was fabricated using two pairs of mesoporous carbon electrodes and [C2mpyr][FSA]/LiFSA, as shown in Figure 1B. Figure 5A illustrates the charge–discharge curves of the bipolar cell assembled with [C2mpyr][FSA]/LiFSA at a constant rate of 4 C. The bipolar cells were charged up to 5.0 V at 25°C. The bipolar cell with [C2mpyr][FSA]/LiFSA demonstrated a typical curved charge–discharge profile with a negligible IR drop at the potential switching point. The capacitance of the bipolar cell was 6.4 Fg−1 at 4 C, which is the half to that of a single cell. The bipolar cell was galvanostatically cycled at 16 C between 0 and 5.0 V for 1,000 cycles. Figure 5B shows the cyclic performance of the bipolar cell by charge–discharge tests at a constant rate of 16 C at 25°C. The stable cyclic performance of the bipolar cell was confirmed during 1,000 charge–discharge tests.

FIGURE 5. (A) Charge–discharge curves of the bipolar cell assembled with [C2mpyr][FSA]/LiFSA at a constant rate of 4 C, and (B) cyclic performance of the bipolar cell evaluated using charge–discharge tests at a constant rate of 16 C.
Conclusion
In conclusion, supercapacitors were fabricated using mesoporous carbon electrodes and an IPC, [C2mpyr][FSA]. The two solvents, AN and DMC, were used to investigate the effect of the solvent species on the pore filling of the electrodes. Remarkably, the two solvents showed significant differences. The electrode pores are thoroughly filled with the electrolyte when using AN, while a large number of voids are observed in the pores of the electrode when using DMC. Hence, the choice of solvent species is essential for preparing mesoporous carbon electrodes filled with IPCs. The charge–discharge tests of the supercapacitors assembled with [C2mpyr][FSA]/LiFSA were performed at various C-rates, where the supercapacitors were charged up to 2.5 V at 25°C. The supercapacitors with [C2mpyr][FSA]/LiFSA exhibited almost similar C-rate dependence of capacitance to that of the TEMABF4/PC system up to 500 C. Subsequently, the capacitance of the [C2mpyr][FSA]/LiFSA system rapidly decreased compared to that of the TEMABF4/PC system. Therefore, further improvements in the ionic conductivity of IPC electrolytes are required to achieve improved capacitance at higher C-rates. An all-solid-state high-voltage supercapacitor with [C2mpyr][FSA] was also developed. Bipolar cells were fabricated using two pairs of mesoporous carbon electrodes and a [C2mpyr][FSA]/LiFSA composite. The bipolar cell with [C2mpyr][FSA]/LiFSA exhibited a typical curved charge–discharge profile in the voltage range of 0–5.0 V. Furthermore, the stable cyclic performance of the bipolar cell was confirmed during 1,000 charge–discharge tests. Thus, this study demonstrates that [C2mpyr][FSA], a pyrrolidinium salt-based IPC, can be utilised in supercapacitors. As a next step, the development of lithium-ion capacitors (Naoi and Simon, 2008;Simon and Gogotsi, 2020) will be an interesting topic because [C2mpyr][FSA]/LiFSA functions as a lithium-ion conductor.
Data Availability Statement
The original contributions presented in the study are included in the article/Supplementary Material, further inquiries can be directed to the corresponding author.
Author Contributions
MY-F, SK, and SI designed the study. MY-F and SK prepared the samples and measured their properties. MY-F, SK, and SI participated in data analysis. MY-F wrote the manuscript. All authors contributed to the manuscript and approved the submitted version.
Funding
This work was supported by JSPS KAKENHI (Grant Number 19K05604), JSPS Bilateral Program (Grant Number JPJSBP120199977), and a Sophia University Special Grant for Academic Research.
Conflict of Interest
SK and SI were employed by the company Nippon Chemi-Con Corporation.
The remaining authors declare that the research was conducted in the absence of any commercial or financial relationships that could be construed as a potential conflict of interest.
Publisher’s Note
All claims expressed in this article are solely those of the authors and do not necessarily represent those of their affiliated organizations, or those of the publisher, the editors and the reviewers. Any product that may be evaluated in this article, or claim that may be made by its manufacturer, is not guaranteed or endorsed by the publisher.
Acknowledgments
We would like to thank Editage (www.editage.com) for English language editing.
Supplementary Material
The Supplementary Material for this article can be found online at: https://www.frontiersin.org/articles/10.3389/fenrg.2022.854090/full#supplementary-material
References
Armel, V., Forsyth, M., MacFarlane, D. R., and Pringle, J. M. (2011). Organic Ionic Plastic crystal Electrolytes; a New Class of Electrolyte for High Efficiency Solid State Dye-Sensitized Solar Cells. Energy Environ. Sci. 4, 2234–2239. doi:10.1039/c1ee01062j
Cheng, X., Pan, J., Zhao, Y., Liao, M., and Peng, H. (2018). Gel Polymer Electrolytes for Electrochemical Energy Storage. Adv. Energ. Mater. 8, 1702184. doi:10.1002/aenm.201702184
Chimdi, T., Gunzelmann, D., Vongsvivut, J., and Forsyth, M. (2015). A Study of Phase Behavior and Conductivity of Mixtures of the Organic Ionic Plastic crystal N-Methyl-N-Methyl-Pyrrolidinium Dicyanamide with Sodium Dicyanamide. Solid State Ionics 272, 74–83. doi:10.1016/j.ssi.2014.12.018
Cooper, E., and Angell, C. (1986). Ambient Temperature Plastic crystal Fast Ion Conductors (PLICFICS). Solid State Ionics 18-19, 570–576. doi:10.1016/0167-2738(86)90180-3
Itagaki, M., Suzuki, S., Shitanda, I., Watanabe, K., and Nakazawa, H. (2007). Impedance Analysis on Electric Double Layer Capacitor with Transmission Line Model. J. Power Sourc. 164, 415–424. doi:10.1016/j.jpowsour.2006.09.077
MacFarlane, D. R., Huang, J., and Forsyth, M. (1999). Lithium-doped Plastic crystal Electrolytes Exhibiting Fast Ion Conduction for Secondary Batteries. Nature 402, 792–794. doi:10.1038/45514
Makhlooghiazad, F., Guazzagaloppa, J., O’Dell, L. A., Yunis, R., Basile, A., Howlett, P. C., et al. (2018). The Influence of the Size and Symmetry of Cations and Anions on the Physicochemical Behavior of Organic Ionic Plastic crystal Electrolytes Mixed with Sodium Salts. Phys. Chem. Chem. Phys. 20, 4721–4731. doi:10.1039/c7cp06971e
Miller, J. R., and Burke, A. (2008). Electrochemical Capacitors: Challenges and Opportunities for Real-World Applications. Electrochem. Soc. Interf. 17, 53–57. doi:10.1149/2.f08081if
Naoi, K., and Morita, M. (2008). Advanced Polymers as Active Materials and Electrolytes for Electrochemical Capacitors and Hybrid Capacitor Systems. Electrochem. Soc. Interf. 17, 44–48. doi:10.1149/2.f06081if
Naoi, K., and Simon, P. (2008). New Materials and New Configurations for Advanced Electrochemical Capacitors. Electrochem. Soc. Interf. 17, 34–37. doi:10.1149/2.f04081if
Pandolfo, A. G., and Hollenkamp, A. F. (2006). Carbon Properties and Their Role in Supercapacitors. J. Power Sourc. 157, 11–27. doi:10.1016/j.jpowsour.2006.02.065
Pringle, J. M. (2013). Recent Progress in the Development and Use of Organic Ionic Plastic crystal Electrolytes. Phys. Chem. Chem. Phys. 15, 1339–1351. doi:10.1039/c2cp43267f
Simon, P., and Gogotsi, Y. (2008). Materials for Electrochemical Capacitors. Nat. Mater 7, 845–854. doi:10.1038/nmat2297
Simon, P., and Gogotsi, Y. (2020). Perspectives for Electrochemical Capacitors and Related Devices. Nat. Mater. 19, 1151–1163. doi:10.1038/s41563-020-0747-z
Taberna, P. L., Simon, P., and Fauvarque, J. F. (2003). Electrochemical Characteristics and Impedance Spectroscopy Studies of Carbon-Carbon Supercapacitors. J. Electrochem. Soc. 150, A292. doi:10.1149/1.1543948
Taniki, R., Matsumoto, K., Nohira, T., and Hagiwara, R. (2014). All Solid-State Electrochemical Capacitors Using N,N-dimethylpyrrolidinium Fluorohydrogenate as Ionic Plastic crystal Electrolyte. J. Power Sourc. 245, 758–763. doi:10.1016/j.jpowsour.2013.07.020
Ue, M. (1994a). Conductivities and Ion Association of Quaternary Ammonium Tetrafluoroborates in Propylene Carbonate. Electrochimica Acta 39, 2083–2087. doi:10.1016/0013-4686(94)85092-5
Ue, M. (1994b). Mobility and Ionic Association of Lithium and Quaternary Ammonium Salts in Propylene Carbonate and γ‐Butyrolactone. J. Electrochem. Soc. 141, 3336–3342. doi:10.1149/1.2059336
Ue, M., Takeda, M., Takehara, M., and Mori, S. (1997). Electrochemical Properties of Quaternary Ammonium Salts for Electrochemical Capacitors. J. Electrochem. Soc. 144, 2684–2688. doi:10.1149/1.1837882
Wang, X., Zhu, H., Greene, G. W., Zhou, Y., Yoshizawa-Fujita, M., Miyachi, Y., et al. (2017). Organic Ionic Plastic crystal-based Composite Electrolyte with Surface Enhanced Ion Transport and its Use in All-Solid-State Lithium Batteries. Adv. Mater. Tech. 2. doi:10.1002/admt.201770029
Xu, K., Ding, M. S., and Jow, T. R. (2001). Quaternary Onium Salts as Nonaqueous Electrolytes for Electrochemical Capacitors. J. Electrochem. Soc. 148, A267. doi:10.1149/1.1350665
Yamada, H., Miyachi, Y., Takeoka, Y., Rikukawa, M., and Yoshizawa-Fujita, M. (2019). Pyrrolidinium-based Organic Ionic Plastic Crystals: Relationship between Side Chain Length and Properties. Electrochimica Acta 303, 293–298. doi:10.1016/j.electacta.2019.02.076
Yoshizawa-Fujita, M., Fujita, K., Forsyth, M., and Macfarlane, D. R. (2007). A New Class of Proton-Conducting Ionic Plastic Crystals Based on Organic Cations and Dihydrogen Phosphate. Electrochemistry Commun. 9, 1202–1205. doi:10.1016/j.elecom.2007.01.024
Yoshizawa-Fujita, M., Kishi, E., Suematsu, M., Takekawa, T., and Rikukawa, M. (2014). A Plastic Electrolyte Material in a Highly Desirable Temperature Range: N-Ethyl-N-Methylpyrrolidinium Bis(fluorosulfonyl)amide. Chem. Lett. 43, 1909–1911. doi:10.1246/cl.140833
Yoshizawa-Fujita, M., Nakazawa, M., Takeoka, Y., and Rikukawa, M. (2022). Phase Transitions and Ionic Conductivity of Ionic Plastic Crystals Based on Pyrrolidinium Cations and Dihydrogen Phosphate Anion. J. Non-Crystalline Sol. X 13, 100078. doi:10.1016/j.nocx.2021.100078
Zhou, Y., Wang, X., Zhu, H., Yoshizawa‐Fujita, M., Miyachi, Y., Armand, M., et al. (2017). Solid‐State Lithium Conductors for Lithium Metal Batteries Based on Electrospun Nanofiber/Plastic Crystal Composites. ChemSusChem 10, 3135–3145. doi:10.1002/cssc.201700691
Keywords: ionic plastic crystals, supercapacitors, electrical double-layer capacitors, bipolar cells, pyrrolidinium, solid electrolytes
Citation: Yoshizawa-Fujita M, Kubota S and Ishimoto S (2022) All-Solid-State High-Voltage Supercapacitors Using an Ionic Plastic Crystal-Based Electrolyte. Front. Energy Res. 10:854090. doi: 10.3389/fenrg.2022.854090
Received: 13 January 2022; Accepted: 21 February 2022;
Published: 08 March 2022.
Edited by:
Jun Mei, Queensland University of Technology, AustraliaReviewed by:
Lu Han, Oak Ridge National Laboratory (DOE), United StatesTianyi Liu, Virginia Tech, United States
Copyright © 2022 Yoshizawa-Fujita, Kubota and Ishimoto. This is an open-access article distributed under the terms of the Creative Commons Attribution License (CC BY). The use, distribution or reproduction in other forums is permitted, provided the original author(s) and the copyright owner(s) are credited and that the original publication in this journal is cited, in accordance with accepted academic practice. No use, distribution or reproduction is permitted which does not comply with these terms.
*Correspondence: Masahiro Yoshizawa-Fujita, bWFzYWhpLWZAc29waGlhLmFjLmpw