- 1National Renewable Energy Laboratory, Catalytic Carbon Transformation and Scale-Up Center, Golden, CO, United States
- 2ICM Inc., Technology Development, St. Joseph, MO, United States
One billion tons of biomass feedstocks have been identified for the production of renewable biofuels and biochemicals. This is one of the key carbon feedstocks to supply energy to the transportation sector for light duty, heavy duty and aviation fuels. Utilization of lignocellulosic feedstocks supports an improved energy security by reducing demand of petroleum imports, agricultural development, job creation, and reducing greenhouse gas emissions. To date, however, operational challenges have stymied the industrial production of large volumes of lignocellulosic-based fuels and chemicals. As a result, significant research investment has been led by the United States Department of Energy to understand and improve operational reliability at pioneer cellulosic biorefineries. In this perspective article lignocellulosic conversion technologies are described that have been adopted from the starch ethanol process. The developed process culminated in successful demonstration of 1,000-h integrated runs using several feedstocks, including switchgrass, energy sorghum, and two types of corn kernel fiber. This article highlights process development that solved several of the issues that plagued—and continue to plague—many in the cellulosic sugars space such as biomass feeding into equipment, high ash content, diversified co-product value, and others.
Introduction
Process Integration Approaches to Cellulosic Processing Design
The road to commercialization of lignocellulosic biomass to production of biofuels and chemicals has not been as easy as it was sold to investors a decade ago. At that time, [bio]catalyst costs in pretreatment and hydrolysis were considered the critical path to overcome the hurdles to commercialization. Through significant efforts and good science, producers realized dramatic cost reductions in biocatalyst (enzymes and yeast) in the years that followed. Those milestones were followed by a handful of cellulosic plant groundbreakings, largely using engineering and technology originally developed for the pulp and paper industry, where the process design seemed intrinsically obvious, to fill out the rest of the process design around the key technologies.
Unfortunately, these pioneer plants struggled to realize their design capacity. Consequently, policy incentives remain inconsistent and the second round of cellulosic capacity buildout has not occurred. Many potential producers and investors, seeking to “be first to be second,” feel the correct first-of-its-kind cellulosic process is still not proven out, noting that significant risks remain in realizing design uptime at full scale.
Processes that break down the fiber structure to low-cost liquid fuels has proven dissimilar to processing pulp and paper where the goal instead is to preserve the fiber structure. These differences are reflected in the significant downtime due to retooling and engineering redesign at pioneer plants, many of whom by 2021 have shuttered operations (Slupska and Bushong, 2019). Producers realized they must cope with broad input variations in moisture, ash content, etc. that were not evaluated at relevant scale during development. These issues have been problematic, with many related to materials transport.
This Perspective article describes process development via incremental changes from the commercial starch ethanol process rather than the pulp and paper process. Herein process design concepts are described that culminated in successful demonstration of 1,000-h integrated runs using several feedstocks, including switchgrass, energy sorghum, and two types of corn kernel fiber. This article highlights a process design approach that solved the issues that plagued—and continue to plague—many processes in the pioneer cellulosic space such as biomass feeding of grasses and grain fibers, high ash content, diversified coproduct value, and others.
Managing Material Handling Challenges
Lignocellulosic Feedstocks Systems Are Not Agnostic
The design challenge for an industrial process with poor control of input feedstock quality attributes is exacerbated by the heterogeneous nature of different forms of biomass:
• Agricultural. Agricultural feedstock can be subdivided into several subclasses, including grasses, residues, and energy crops. All these agricultural solids have substantive differences in composition, physical characteristics, harvesting methods and storage conditions.
• Municipal Solid Waste. MSW can be as varied as the collection and sorting methods used to recover the material post-consumer. MSW cellulose content may include different types of extractives, binders, and inks as part of their previous application.
• Captive Fibers. These consist of post-processed food, and are subject to extraction method variations, microbial degradation, and food sources.
• Woody biomass. Woody biomass has been used industrially for centuries. Industrial woody feedstocks are almost always subdivided into hardwood and softwood, with variant processing methods applied to exploit each one. Experience has taught the pulp and paper industry that in many cases, blends of hardwood and softwood can improve their flowability and processability (Chauhan et al., 2011; Nugroho, 2012).
Non-Agnosticism: Switchgrass, Energy Sorghum, and Corn Kernel Fiber
In 2015, industry demonstrated ethanol production using lignocellulosic feestocks, including switchgrass, energy sorghum, and corn kernel fibers at a scale of 10 tons per day of continuous production (Javers, 2017). During those runs, process engineers experienced the inherent variation between feedstocks, even those within a lignocellulose sub-category. Special care had been taken to grow, harvest, store and process the feedstocks in such a way as to avoid storage variability from moisture and decomposition (Smith et al., 2013). However, upon grinding the feedstocks and pneumatically transferring to downstream processing, seemingly innocuous steps like tramp washing and wetting of feedstock led to material bridging, rotary airlock plugging, high wear areas, cooling and pumping issues, among others (Supplementary Figure S1). Those challenges proved difficult to overcome without equipment and process changes specific for each lignocellulose type.
Agricultural Residues Have Many Ash Sources
Ash content is a significant variant in feedstocks for bioprocessing. In addition to bound ash in the form of dirt and intrinsic ash within the feedstock itself, manufacturing plants must also manage the levels of tramp that comes in with biomass (Zhang et al., 2017). In one of the runs, almost two cubic meters of small rocks were removed from just 10–12 tons of feedstock (Javers, 2017), requiring improved storage design.
Feedstock Variation Impacts the Front End
Ash content, stalk thickness and moisture content can have pronounced impacts on the milling operation. When ash content (often as soil) is high, mechanical wear on milling equipment can significantly reduce equipment lifetime and plant uptime. Stalk thickness directly impacts the load on milling devices to the point where staged milling may be necessary to realize a cost-effective attrition to the desired pretreatment particle size (Zhang et al., 2017). Heterogeneity of feedstock quality also has profound impacts upon the particle size distribution of pre-processing: higher moisture contents in milling tend towards larger particles, while dry biomass produces more fine particles and dust, frequently with a wider particle size distribution (Smith et al., 2013). It is here that the benefits of captive [processed] fibers have distinct advantages, with the cost and consistency burden of grinding already being paid for by the primary product (starch ethanol).
Grinding technology also provides process variability that can cause challenges in hydrolysis. Cutting/chipping, shearing, grinding and crushing modes of attrition can alter the biomass particle morphology and size distribution differently. Cutting and chipping—often used for primary milling of biomass to more homogeneous sizes—are limited by cost at lower particle sizes, yet leave the material still too large to effectively convey and seal into high-pressure reactors (Karinkanta, 2015). Secondary milling is often accomplished by hammermills and similar equipment. While effective, they produce a broad particle size distribution. During the United States Department of Energy’s Integrated BioRefinery (DOE IBR) funding of 1,000-h pilot trials at ICM, a population of unconverted particles persisted even after hydrolysis for both switchgrass and energy sorghum (Figure 1). A sugar clarification step was applied to prevent these large pieces from moving forward in the process and causing issues.
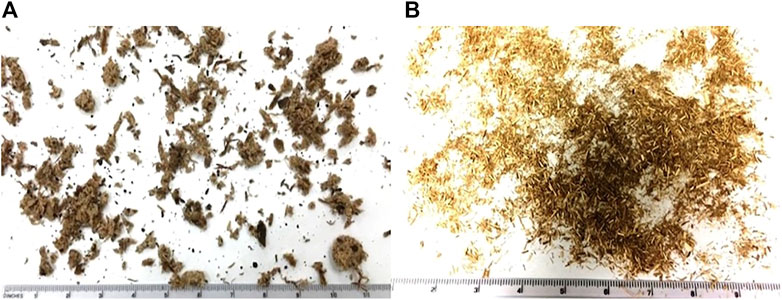
FIGURE 1. Comparison of woody and herbaceous feedstocks under similar pre-processing particle size reduction. Wood chips (A) and switchgrass (B) were processed with a hammer mill with screen size #10. The particle and conglomerate material attributes differ substantially using similar processing methods. Thus, the need to tailor handling equipment designs for each feedstock is critical.
Normalizing Storage to Manage Heterogeneity
Storage can play a large role in downtime resulting from some of the above variations. Recently, the national laboratories successfully demonstrated the impact of adaptive controls on reducing downtime of a mill grinding corn stover with variable moisture content (US Department of Energy, 2016). Although uptime was increased, there was a significant loss of rate.
A second approach is to implement a standard feedstock form, such as pellets (Kim et al., 2019). Pellets have the advantages of pre-milled feedstock, reduced moisture variation, improved transportation costs, and ability to use existing infrastructure. Care must be taken with a pelleting approach however to ensure full, rapid and cost effective rehydration at the biorefinery. As of this writing, there are no known uses of pelleting in the biomass process (Tumuluru et al., 2011).
In the end, to process slurries with high solids concentrations (>20% solids to liquid) it may be more pragmatic to control variation by employing bulk averaging to dampen inhomogeneity. This introduces a substantial cost to many biomass feedstocks, and in some cases makes it more expensive than its competitive carbohydrate source: grain starch.
The Biomass Conveying Challenge
The compressibility, cohesivity, plasticity and low bulk density of most biomass sources make it difficult to design, and many pioneer plants are known to have gone through redesigns of the feedstock conveyance systems. Some IBRs found that pre-ground feedstocks pneumatically transported from storage into the plant required a steady feed rate to prevent line plugging (US Department of Energy, 2016).
Mitigating the conveying challenge is not easy or inexpensive for dry solids streams. Narrowing the particle size is often the easiest and most effective mitigation for heterogeneous materials. At smaller particle sizes (50–1,500 micron), most feedstocks act like spheres and flow freely. However, much research has demonstrated that grinding of biomass below 10 mm introduces an unacceptable cost to production (Humbird et al., 2011; Hartley et al., 2021).
To reduce the impact of mechanical cohesivity, dilution of the feedstock with air can help. Making a robust, functioning system involves careful consideration of the feed rate, air velocity and line layout. Overfeeding the conveyance system can result in settling/packing of material. At high air velocity, the biomass stays dilute but wear at piping elbows increases (Supplementary Figure S1); too low velocity and material falls out and settles in the line. Too long of a horizontal run—especially one with many elbows—and gravity settles the biomass out in the lines, often requiring human intervention to restore flow.
Hydration—The Floaters and Sinkers Challenge
In order to achieve optimal heating of biomass, fully hydrating the material is not easily achieved for all feedstocks. Switchgrass—presumably due to its stem coating—is easy to wash but problematic to wet, making tempering of the feedstock to constant total solids challenging. Switchgrass formed floating mats on top of IBR slurring vessels; it was difficult to maintain homogeneity, a problem that was solved only after many attempts and equipment modifications (Figure 2A).
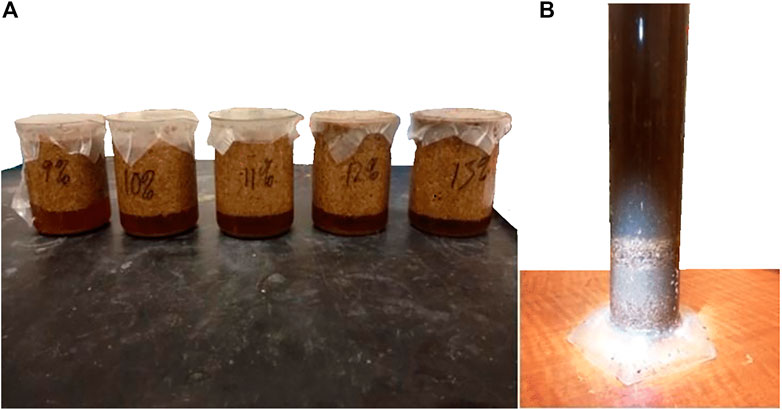
FIGURE 2. Dilute acid pretreatment switchgrass (A) and energy sorghum (B) illustrating the floater and sinker characteristic, respectively. Two seemingly similar herbaceous materials pre-processed and thermochemically pre-treated behave quite differently when suspended in liquid. Thus, the equipment design and process for the transport of materials needs is unique for specific feedstock properties throughout the process.
Conversely, energy sorghum wet too readily. In washing steps, sorghum retained water, resulting in inefficient ash removal and leading to a higher acid requirement and elevated levels of equipment wear. In the slurry vessel, the sorghum would readily dewater and sink. Special controls had to be employed to maintain consistent total solids while continuous feeding into the downstream reactor (Figure 2B).
Industry processes used suspended solids instead of wetted solids, thus more aggressive horsepower and thoughtful agitation were adequate to maintain the homogeneity necessary to achieve reliable flow control.
Avoiding Backpressure at the Reactor Throat
Achieving the seal at a high-pressure reactor throat is challenging for solids systems like biomass and can have catastrophic impact on plant uptime and safety if the boundary between high and low pressure fails. If corrosive catalysts are able to blow back in the process, the result can introduce rapid degradation of upstream equipment, especially seals. Backpressure can also cause elevated reaction temperatures that make the area an unsafe work environment.
Many high solids approaches involve mechanically compressing the biomass feed material into a plug, while others attempt to form a seal with the biomass directly. In early designs, one IBR attempted both approaches, neither of which ran successfully for more than a day or two. One key issue was gas permeability in and around the biomass. It was difficult to consistently and sustainably create a seal with material present in the seal; soft seals broke down under the abrasiveness of the biomass, and hard seals would not close completely unless they managed to cut the material or move it from the sealing surface.
The Move to Lower Solids Processing
Due to these challenges during development, one IBR process opted to take a different direction with the reactor design. Whereas making a high-pressure seal with biomass requires complex mechanical sealing systems, making a seal with liquids is relatively simple and reliable. By reducing the total solids target in pretreatment, the demonstration plant achieved a slurry with larger particles yet consistent operation. Additional benefits included superior heat transfer, mixing, pumping and flash. An artifact of running pretreatment at lower total solids was that hydrolysate sugar concentrations were low, but the costs were effectively mitigated with waste heat evaporators to concentrate the sugars. The approach effectively decoupled the pretreatment and hydrolysis operating conditions from the fermentation such that the pretreatment optima did not limit the fermentation ethanol titer. It was possible to run hydrolysis at only 100 g-sugars/L but still run fermentation at 300 g-sugars/L to realize cost-effective distillation.
Impacts of Lower Solids Front End
A slurry step is often used prior to the pretreatment reactor to condition and temper the biomass for optimal reaction. Removal of buffers, such as ash or proteins, improves the catalyst performance. Additionally, elevating the biomass temperature can improve the heating process by reducing the amount of steam required to reach the reaction temperature. The slurry step also can be utilized to introduce some (or all) of the catalyst where it can be done in a more dilute environment with better mixing.
Pretreatment slurry conditioning depends upon reactor design. Direct steam injection is used in many applications because it is efficient at heating. However, steam injection has the disadvantage of being difficult to distribute evenly due to increased solids, diluted biomass, and requirements on steam quality. Similarly, chemical additions can be problematic (pH adjustment and control) in packed fiber beds where tempering the biomass in a slurry may be limited to avoid over-dilution.
Some process designs utilize slurry to wash and/or transport the biomass to the rector throat, then recover the water and recycle it back to the front of the transport. This approach can mitigate several issues in the process while also saving water, provided considerations are made to reduce build-up of unwanted compounds in the biomass flow that enters the reactor (ex. ash, dirt, and sugars). This must be balanced with additional capital cost and water balance management. The slurry and/or hydration liquids may not be sent forward with the biomass in the reactor to reduce dilution. When catalyst is applied prior to the reactor where some hydrolysis may occur, it is generally necessary to send the liquid into the reactor with the solids to maintain mass balance control.
A Case for Co-Location
Water balance is arguably the most important consideration with the frontend of a biorefinery. Water provides the heating and cooling, some of the catalyst, and functionally distributes all other chemistry at play. When a biorefinery is designed to be a standalone operation, water and energy must balance. However, when a cellulosic process is co-located with another process, many new and advantageous integrations can be realized.
Consider the biorefinery design where all of the lignin is combusted for energy (Davis et al., 2018). In this case, the lignin volume provides a net electricity export to the grid. However, electricity gets discounted when it goes onto the grid because of unavoidable power transmission losses. If a suitable colocation partner is at-site, all value of the electricity can be utilized by that process at (green) market price.
In some 1.5 generation (captive/corn fiber) processes, sulfuric acid and ammonia used in pretreatment are carried over into the starch based 1st Generation (1G) process at the integrated corn fiber fermentation. The chemicals are still able to perform their normal functions as nitrogen source for yeast growth and pH control for evaporation, effectively cutting the acid/base consumption in half relative to a standalone ethanol plant. Additionally, waste heat from the pretreatment can be used to drive 1G evaporation, and cook water from the 1G ethanol process used for fiber treatment and washing prior to dilute acid pretreatment. Recalcitrant protein on the incoming fiber is partially hydrolyzed during pretreatment, increasing the overall protein efficacy (digestibility) in the feed product (Karinkanta, 2015). Ultimately, all insoluble solids from the 1G process end up in the animal feed stream, providing higher value to a plant than combustion for power.
In a co-located 1st and 2nd generation (cellulosic) biorefinery, the size of the cellulosic site relative to the 1G site next door depends upon integration of the nominal sulfuric acid dose in pretreatment, somewhere between 25–50% of the starch ethanol output. The 1G plant provides inexpensive carbohydrate for the yeast propagation, such that an excess of yeast is produced and can be sold as a high value single cell protein or combined with the rest of the feed ration to boost the overall protein content. The pretreatment provides both steam and waste heat to the 1G process as well.
To date, the process decision to use high dry matter pretreatment for cellulosic processes has been challenging for a large portion of the pioneer cellulosic ethanol plants. The high level of variation in real-world feedstocks has made it difficult to design a robust frontend process to deliver the feedstock to the high-temperature and -pressure reactors with low free water. For biochemical route processes, the lower solids approach of the process will average many of the feedstock quality differences and provide reliable flow into the pretreatment reactor.
IBR process integration allowed for low solids to be done so economically. Colocation afforded many advantages to the water and energy balances of the lignocellulosic facility. As a result, the resulting cellulosic process has a lower capital cost per gallon ethanol than most reported cellulose plants in operation. There may also be other advantages of co-localization such as utilizing the waste CO2, fertilizer and nutrient separation to take advantage of national and regional incentives to realize low carbon fuels and chemicals.
Discussion
Cellulosic ethanol was validated with over 4,000 h of integrated run at 10 tons per day in the ICM IBR pilot plant, using switchgrass, energy sorghum and two types of corn kernel fiber. The validation process also used a frontend capable of mitigating feedstock variation for more than 400 tons of biomass processed during each trial. It was facilitated by use of incremental engineering changes from the first-generation ethanol process, instead of the more common adaptation of the industrial Pulp and Paper process. There is a foundational truth that feedstock diversity can (and will) resist a “one size fits all” process design for all sources of biomass. The perspectives described in this article are a recognition of the importance of employing demonstrated tools for reducing material flow heterogeneity and variation over time—most notably, use of dilution and benefits of pairing the process with adjacent manufacturing to mitigate water balance and ash limitations. Agricultural feedstock variability is inevitable but leveraging decades of experience with processing cereal grains has demonstrated at scale a cellulosic systems that can be successful in realizing robust operations with low downtime, and as such, should be a consideration of anyone trying to mitigate risk in biorefinery development.
Data Availability Statement
The original contributions presented in the study are included in the article/Supplementary Material, further inquiries can be directed to the corresponding author.
Author Contributions
BE drafted and created the concept of the article. MR contributed to the writing and editing of the article.
Funding
This work was authored in part by the National Renewable Energy Laboratory, operated by Alliance for Sustainable Energy, LLC, for the United States Department of Energy (DOE) under Contract No. DE-AC36-08GO28308. Funding provided by the United States Department of Energy Office of Energy Efficiency and Renewable Energy Bioenergy Technologies Office. The views expressed in the article do not necessarily represent the views of the DOE or the United States Government. The United States Government retains and the publisher, by accepting the article for publication, acknowledges that the United States Government retains a nonexclusive, paid-up, irrevocable, worldwide license to publish or reproduce the published form of this work, or allow others to do so, for United States Government purposes. Development work described in this article was funded in part by the US Dept of Energy via the Recovery Act: Pilot Integrated Cellulosic Biorefinery Operations to Fuel Ethanol FOA award number DE-EE0002875, and via private funding by ICM, Inc.
Conflict of Interest
Author BE is employed by ICM, Inc., a privately owned and for-profit company providing technology services to the bioethanol industry.
The remaining authors declare that the research was conducted in the absence of any commercial or financial relationships that could be construed as a potential conflict of interest.
Publisher’s Note
All claims expressed in this article are solely those of the authors and do not necessarily represent those of their affiliated organizations, or those of the publisher, the editors and the reviewers. Any product that may be evaluated in this article, or claim that may be made by its manufacturer, is not guaranteed or endorsed by the publisher.
Acknowledgments
The authors would like to acknowledge the support of the National Renewable Energy Laboratory in Golden, CO and ICM, Inc. in Colwich, KS for their contributions to the article contents.
Supplementary Material
The Supplementary Material for this article can be found online at: https://www.frontiersin.org/articles/10.3389/fenrg.2022.835714/full#supplementary-material
References
Chauhan, V., Chakrabarti, S. K., and Thapar, S. K. (2011). Effect of Separate and Mixed Refining of Hardwood and Softwood Pulps on Paper Properties. Palpu Chongi Gisul/Journal Korea Tech. Assoc. Pulp Paper Industry 43, 142.
Davis, R. E., Grundl, N., Tao, L., Biddy, M. J., Tan, E. C. D., Beckham, G. T., et al. (2018). Process Design and Economics for the Conversion of Lignocellulosic Biomass to Hydrocarbon Fuels and Coproducts: 2018 Biochemical Design Case Update; Biochemical Deconstruction and Conversion of Biomass to Fuels and Products via Integrated Biorefinery Pathways. Golden, CO (United States): National Renewable Energy Lab. NREL.
Hartley, D. S., Thompson, D. N., and Cai, H. (2021). Woody Feedstocks 2020 State of Technology Report. United States. Medium: ED.
Humbird, D., Davis, R., Tao, L., Kinchin, C., Hsu, D., Aden, A., et al. (2011). Process Design and Economics for Biochemical Conversion of Lignocellulosic Biomass to Ethanol. Renew. Energ. 303 (May), 147.
Javers, J. (2017). Recovery Act: Pilot Integrated Cellulosic Biorefinery Operations to Fuel Ethanol. United States, 152. Medium: ED; Size.
Karinkanta, P. (2015). Dry fine Grinding of Norway spruce (Picea Abies) wood in Impact-Based fine Grinding Mills.
Kim, S., Dale, B. E., Jin, M., Thelen, K. D., Zhang, X., Meier, P., et al. (2019). Integration in a Depot‐based Decentralized Biorefinery System: Corn stover‐based Cellulosic Biofuel. GCB Bioenergy 11 (7), 871–882. doi:10.1111/gcbb.12613
Nugroho, D. (2012). Low Consistency Refining of Mixtures of Softwood & Hardwood Bleached Kraft Pulp: Effects of Refining and Power.
Slupska, M., and Bushong, D. (2019). Lessons from Commercialization of Cellulosic Ethanol - A POET Perspective. Biofuels, Bioprod. Bioref. 13, 857–859. doi:10.1002/bbb.2033
Smith, W. A., Bonner, I. J., Kenney, K. L., and Wendt, L. M. (2013). Practical Considerations of Moisture in Baled Biomass Feedstocks. Biofuels 4 (1), 95–110. doi:10.4155/bfs.12.74
Tumuluru, J. S., Wright, C. T., Hess, J. R., and Kenney, K. L. (2011). Erratum: A Review of Biomass Densification Systems to Develop Uniform Feedstock Commodities for Bioenergy Application. Biofuels Bioproducts and Biorefining 1, 5. doi:10.1002/bbb.324
Us Department of Energy, (2016). “US Department of Energy-Office of Energy Efficiency and Renewable Energy–Bioenergy Technologies Office. Summary Report from the October 5-6,” in 2016 Biorefinery Optimization Workshop in Chicago, Illinois. DOE/EE-1514 December, Chicago, Illinois, October 5-6.
Keywords: biomass, biofuels (biodiesel, bioethanol, biogas), biochemicals and bioenergy, biochemical conversions, lignocelllulose saccharification, biorefineries
Citation: Resch MG and Emme B (2022) Using Incremental Changes to Convert Lignocellulosic Feedstocks to Cellulosic Ethanol. Front. Energy Res. 10:835714. doi: 10.3389/fenrg.2022.835714
Received: 14 December 2021; Accepted: 16 February 2022;
Published: 21 March 2022.
Edited by:
Timothy G. Rials, The University of Tennessee, Knoxville, United StatesReviewed by:
Nídia S. Caetano, Instituto Superior de Engenharia do Porto (ISEP), PortugalCopyright © 2022 Resch and Emme. This is an open-access article distributed under the terms of the Creative Commons Attribution License (CC BY). The use, distribution or reproduction in other forums is permitted, provided the original author(s) and the copyright owner(s) are credited and that the original publication in this journal is cited, in accordance with accepted academic practice. No use, distribution or reproduction is permitted which does not comply with these terms.
*Correspondence: Brandon Emme, YnJhbmRvbi5lbW1lQGljbWluYy5jb20=