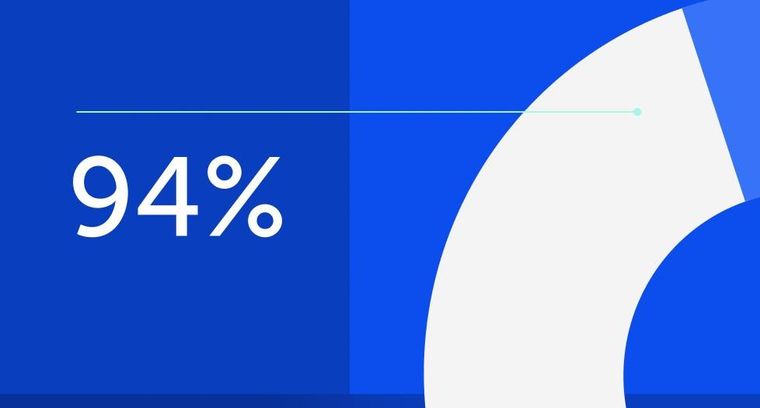
94% of researchers rate our articles as excellent or good
Learn more about the work of our research integrity team to safeguard the quality of each article we publish.
Find out more
PERSPECTIVE article
Front. Energy Res., 06 January 2023
Sec. Nuclear Energy
Volume 10 - 2022 | https://doi.org/10.3389/fenrg.2022.1102466
This article is part of the Research TopicAdvancements in Nuclear and Irradiation ExperimentsView all 6 articles
The behavior of fission gases in molten fuel salt reactors governs activity transport from the reactor and can also affect the performance of the reactor itself. The gas solubility can be described thermodynamically by Henry’s law. However, the coupling of the condensed and gas phases depends on the interfacial area, which is difficult to measure or even to estimate. Surfaces of materials in the reactor will include disperse phases in the salt and porosity within the structural materials, covering a range of compositions and sizes. These attributes can affect measurements of fundamental properties such as gas solubility. Methods to obtain gas solubility, surface tension, interfacial energies, and bubble gas transport are reviewed. Recent data from manometric experiments are interpreted based on xenon sorption onto salt-wetted quartz.
Unlike ceramic fuels, fission products’ transport in molten salt reactors is not restricted by cladding. Instead, the pressure boundary is the off-gas system, which may be swept by a carrier gas (Andrews et al., 2021). Xenon, krypton, and tritium transport directly into the gas where it contacts the salt, such as the pump bowl, and are continuously removed. The fission products that are readily soluble in the salt can move into the cover-gas flow by forming mists or aerosols. Confinement in the off-gas allows these radionuclides to decay and the nonvolatile daughters condense in the off-gas components, decay tank, scrubbers, or filters (McFarlane et al., 2018).
Fission gases comprise the largest fraction of fission products—almost half—and xenon isotopes are the largest contributors. The isotopes of xenon and krypton have a wide range of half-lives. Fission gases are of concern for two main reasons (Price et al., 2020). Some, notably 135Xe, can affect reactor operation as a neutron poison under shutdown and restart conditions. Reaction 1) shows the decay chain from 135I that forms 135Xe. During normal operation, the abundance of 135Xe is controlled by neutron capture to 136Xe. Furthermore, because of their abundance and volatility, noble gas isotopes are the main contributors to the radionuclide load in the cover gas system. For instance, 137Xe, which has a half-life of 3.95 min, decays to 137Cs, as shown in Reaction 2). This fission product is tracked because of its potential uptake into the environment and its 30-year half-life, which contributes a significant decay heat load to cover gas components.
In molten salt reactors, gas behavior is governed by several dynamic and complex processes, including generation by fission or through radioactive decay, transport through temperature gradients in the primary heat transport system, gas sparging to push bubbles from the melt, adsorption onto surfaces, and ingress into structural materials such as graphite. Despite these complications, the solubility of the gas in the salt is fundamental to understanding noble gas transport.
The partitioning of noble gases between the gas and condensed phases can be described by chemical thermodynamics, which relates the partial pressure of the gas to the concentration in the condensed phase, Eq. 1a and Eq. 1b, where
This perspective discusses the measurements of noble gas solubilities that support reactor simulation and the difficulties experienced in the design of the experiments.
Tomkins (2003) reviewed the methods for measuring gases in molten salts. These measurements can be obtained either by directly monitoring the absorption of the gases into the molten salt or by using a two-step approach that involves a sparging step to saturate the salt with the gas of interest followed by a stripping and analysis step.
Absorption of a gas into a molten salt can be monitored in a quiescent system. In these experiments, a physical change in the system results from the uptake of gas into the salt mixture. This change manifests as changes in volume at constant temperature and pressure or as changes in pressure at constant temperature and volume. Because no active mixing occurs, except by convection, these measurements can take several hours. Another approach is to monitor absorption actively in a molten salt, that is, well stirred by a sparge of the analyte in a carrier gas. If the analyte has an appropriate spectroscopic signature, then these measurements can be performed on the salt in situ because the carrier is often optically transparent (Medina et al., 2022). Thermogravimetry tracks increases in salt mass as a gas mixture carrying the analyte contacts the salt (Bratland and Corbett 1966). This method requires the gas to have significant solubility in the salt and is sensitive to changes in buoyancy with the flow of gas and to losses of semivolatile components from the salt by evaporation. Consequently, traditional methods for direct observation of gas uptake are not likely to be feasible for tracking absorption of noble gases into molten salts.
Two-step measurements include saturation of the salt with the gas mixture, followed by posttest analysis in a second step. This approach was demonstrated at the United States Department of Energy’s Oak Ridge National Laboratory during the 1950s and 1960s. Colligative properties such as freezing point depression can give dissolved gas concentration, should they provide sufficient sensitivity. This method is not appropriate for measurements on noble gases, which evaporate below the solidus. However, as the gas comes out of solution during freezing, it can be captured and analyzed by mass spectrometry. The molten salt can be transferred to a second vessel to minimize contaminating the collection system with the initial sparging mixture. Alternatively, the salt can be actively stripped by a different carrier gas. The mixture containing the stripped analyte can be analyzed by mass spectroscopy, titration, electrochemistry, and gas chromatography (Field and Shaffer 1967). Grimes and others (1958) used the sparging/stripping method to measure gas solubilities in both fluoride and chloride salts by gas chromatography. Hamilton and Inman (1992) performed similar measurements on chloride salts. These studies all required kilograms of salt to achieve the needed sensitivity. Higher sensitivity can come from radiometric analysis, e.g., measuring 14C for tracking CO2 uptake (Numata and Bockris 1984).
Recent experiments investigated the uptake of argon and xenon in chloride salts in chloride salt mixtures (Moon et al., 2022a). The salt mixture was melted in an open-ended quartz tube in an argon-filled glove box. The tube was placed inside a larger stainless-steel tube that could be isolated form the atmosphere by two valves, one on the gas inlet to the system and the other on the outlet. After being sealed, the apparatus was moved to a vertical furnace. The vessel was attached to vacuum and gas lines that allowed the space above the salt to be evacuated or permitted the introduction of the gas of interest, either argon or xenon.
Manometric experiments were conducted by evacuating the headspace above the salt and heating the salt to the desired temperature while under vacuum. The salt was once again degassed while at temperature to −800 mbar. The gas of interest was added to the system, to 0.5, 1.0, or 1.8 bar absolute. The pressure drop was measured over time until it stabilized corresponding to an equilibrium between the gas and the molten salt. These measurements were repeated for several different chloride salt systems that included NaCl, MgCl2, KCl, and UCl3 at temperatures from 525°C to 725 °C. The pressure drop data were fit to a kinetic model as published by Librovich et al. (2017), Eq. 2 (Weber 2022).
The symbols include: Si—rate of mass transfer (mol∙s−1) at time t s), Γi—mass transfer coefficient (unitless), A—interfacial area between the salt and the gas phase (m2), R—ideal gas constant (g∙m2∙s−2∙mol−1∙K−1), T—temperature (K), and
These values were compared with solubilities reported in the literature. None of the reported salt systems were identical to those reported here but serve as a rough comparison. Watson et al. (1962) measured Henry’s law coefficients for xenon in LiF-BeF2 as 2.35 × 10–6 to 8.63 × 10–6 mol dm−3 bar−1 for 600°C–800°C. They also performed measurements on NaF-ZrF4-UF4, NaF-ZrF4 (Grimes et al., 1958), and LiF-KF-NaF (Blander et al., 1958) mixtures that showed comparable results. Published noble gas solubilities in chloride systems include argon in NaCl from 850°C to 950°C and in KCl from 827°C to 1227°C (Noviozhilov 1973; Woelk 1960), which yielded Henry’s law coefficients in the range of 10–4 to 10–3 mol dm−3 bar−1. The current experimental results were much higher than those previously reported, except for the third measurement for xenon at 725°C. When comparing the noble gases, results showed that uptake into the salt was higher for argon than for xenon by a factor of two, which is consistent with the literature (Grimes et al., 1958). The enthalpy of solution (∆Hsoln) for xenon was close to that reported for xenon in fluoride systems: ΔHsoln = 48 kJ mol−1 vs. 46.4 kJ mol−1 for xenon in NaF-ZrF4 (Watson et al., 1962). The accommodation coefficient, or Γ, derived from the fit of Eq. 1, ranged from 4 to 25 for the xenon and argon systems. This result is unphysical because, by definition, the coefficient varies from 0 (no uptake) to 1 (every atom contacting the surface dissolves into the salt).
The rates of noble gas uptake into molten salts and their measured solubilities were higher than expected. These effects may have arisen from the surface tension of the salt and the interfacial interaction between the salt and the quartz tubing containing the salt.
Although not numerous, surface tension measurements for molten salt systems are available. Data for surface tension of chloride salts are reviewed by Janz et al. (1975), from maximum bubble pressure, Whilhemy slide plate, and pin detachment methods. Data for the NaCl-MgCl2 systems were obtained by measuring the force required to pass a bubble of gas through a platinum capillary. Measurements started at 700°C giving a value 0.088 N m−1 (Sokolova and Voskresenskaya, 1966). These values are very close to the surface tension of liquid water (Rivera et al., 2006). Many salts also exhibit strong interfacial properties, e. g., creep of chloride salts was measured by Stepanov et al. (1989); Stepanov (2018) on a variety of surfaces. Dogel et al. (2003) described chloride salt creep in term of the excess Gibbs energies. This excess energy may have complicated our recent gas solubility measurements.
Neutron radiography gives credence to the possible role of interfacial interactions. The radiographs were taken to determine the density of the chloride salt mixtures above the melting point (Moon et al., 2022b). Selected radiographs are shown in Figure 1: each quartz tube contains a different mixture. Figure 1A shows a radiograph of a molten chloride salt with entrained bubbles. Figure 1B shows a salt sample heated in an evacuated quartz tube, illustrating variations in contact angles that depend on whether the system is advancing (being heated) or retreating (being cooled). Chloride salts are not expected to react with quartz, and certainly any reaction is slow. However, some literature report that cations can exchange with the quartz, leading to irreversible surface modification (Gao et al., 2005). In our tests, 1 day of annealing at temperature did not affect the contact angle variation.
FIGURE 1. Radiographs of molten chloride salts. (A) Above the melting point (800°C), entrained bubbles occur in NaCl-KCl. (B) A salt, that is, undergoing its second heating cycle has menisci that indicate wetting and nonwetting for the same sample. This figure is modified from Moon et al. (2022b) (Figure 2).
Thus, surface wetting could effectively increase the salt surface area exposed to the gas, thereby increasing the rate of uptake into the salt. This phenomenon explains the unphysically high accommodation coefficient observed in these experiments. For instance, the cross-sectional area of the salt enclosed in a 4.1 mm inner-diameter quartz tube should be 13.1 ± 0.2 mm2. However, salt wetting 0.3–2.4 cm of tubing above the expected meniscus leads to an effective increase in surface area of 52–328 mm2, explaining the larger accommodation coefficient.
The accommodation coefficient is plotted vs. temperature in Figure 2, decreasing as expected with physisorption. If the data are plotted as an Arrhenius plot (ln Γ) vs. inverse temperature, then the slopes of the lines correspond to −14 kJ mol−1 for the argon system and −42 kJ mol−1 for the xenon system. The enthalpy of adsorption (∆Hads) results for argon are close to ΔHads = −8 kJ mol−1, comparable to literature values for adsorption of argon on graphite (Farías Hermosilla and Albesa 2020). This result suggests that the gas, when introduced into the system, rapidly adsorbed onto salt-wetted surfaces. Once physisorbed, the gas would remain bound unless deliberately degassed, such as stripping with a flow of inert gas or sharply increasing the temperature. Such efforts would also perturb the equilibrium of the salt with the dissolved gas.
FIGURE 2. Accommodation coefficient as a function of temperature for argon in NaCl-MgCl2, (A,B) and xenon in NaCl-MgCl2 (C,D) The accommodation coefficients decrease with temperature (A,C). The enthalpy can be calculated from ln(Γ) vs. 1/T.
Other effects could cause problems for noble gas solubility measurements. The volatility of salts containing MgCl2 is well understood (Schrier and Clark 1963). When heated to 400°C, even purified salts can produce HCl(g), which is generated by the liberation of hydration water and the reaction with the chloride salt (McFarlane et al., 2022). Gas uptake cannot be measured in salts that evolve gases or that have volatile components because these systems are unstable. Consequently, few data on gas solubility in molten MgCl2 are available. This same concern applies to salts that react with their containers, slowly changing composition during the measurement. In molten salt reactors, adding fission products to the salts would be expected to decrease the density. However, because some of the fission products are themselves volatile or semivolatile, this assumption is difficult to validate in a static system. Noble gases are expected to coalesce into bubbles and transport out of solution (Frederix 2022).
Radiolysis is expected to generate secondary phases in molten salt systems, even those that operate at temperatures above 400°C (Roy et al., 2021). Radiolytic damage of reactor surfaces, such as graphite, will generate fine particles (Lee et al., 2020). Noble metal fission products have unstable fluorides and chlorides and will form dispersed solids in the salt, perhaps serving as nucleation loci for noble gas bubble generation. Corrosion products, such as chromium, generated from the reaction of stainless steel with salts, can accumulate in solution and form ternary compounds (Sprouster et al., 2022). Certain fission products, such as tellurium, have complicated chemistry. Tellurium can combine with noble metals to form compounds such as PdTe, and tellurium has also been implicated in stress-corrosion cracking of reactor alloys (Ignatiev et al., 2013). Tritium as a ternary fission or activation product is expected (Dolan et al., 2021). Fission changes the salt’s redox state and the chemistry of fission products within the salt, including noble gas solubility. Fission gases can penetrate and degrade nuclear graphite, which enhances alloy corrosion (Lee et al., 2020). Therefore, the transport and partitioning of fission gases between phases in a molten salt reactor governs complex and interdependent phenomena.
The characterization of complex behavior must start somewhere: gas solubilities provide an initial explanation of noble gas behavior in molten salts. Solubility measurements provide a starting point for modeling and simulation of noble gas behavior during burnup. Successful solubility measurements on molten salts have used kilograms of salt, maximizing the volume of the salt relative to the surface area of the apparatus. Gases were introduced by bubbling or sparging the gas through the salt, creating a well-mixed system. However, such large-scale experiments are not feasible for highly radioactive salts, such as those containing plutonium and higher actinides.
To acquire solubility data, controlling the dynamic processes and minimizing the perturbations in the salt is of utmost importance. The salt must be contained in a material with which it does not react. Even refractory materials can experience irreversible changes during a measurement over several hours. Measurements in fluoride salts benefit from vessel contact with a sacrificial salt, that is, removed for disposal before the actual tests take place. Chloride salt containers may require coatings of protective noble metals, e.g., iridium. Alternatively, salts could be transferred to a secondary vessel before analyzing the gas uptake, selectively sampling away from vessel walls. Controlling creep, capillary transport, and other effects that distort the salt’s geometry is also important. Changes in salt composition via incongruent volatilization must be minimized.
Measuring the salt temperature can be difficult because temperature gradients in the experiment can cause the salt to be several degrees hotter than the gas above the salt. This effect can be minimized by applying additional heaters, like the guard heaters used in thermal conductivity measurements (Gallagher et al., 2022), as well as thermal insulation, well-calibrated systems, and long equilibration times.
As was observed in the experiments discussed herein, adsorption of gases, even noble gases, onto surfaces can occur (Stach et al., 1986). The apparatus and methodology must be designed to promote the measurement of gas absorption into the condensed phase rather than adsorption onto apparatus surfaces. This outcome can be achieved by separating the uptake and measurement steps, moving the saturated salt to a secondary container where measurements can be made. Direct measurement of the analyte in the salt itself will avoid errors, by spectroscopy or radiotracers. Gas-phase measurements of noble gases have been achieved by laser-induced breakdown spectroscopy and could be applied to the liquid (Andrews et al., 2022).
Knowing the noble gas solubilities in molten salts is crucial for understanding reactor behavior and radionuclide transport. Because the physical phenomena governing noble gas partitioning are complex, it is important to ensure that solubility measurements target the correct behavior. This accuracy can be achieved by careful apparatus design, calibration with known salt/gas systems, and development of a methodology for accurate measurements.
The datasets presented in this study can be found in online repositories. The names of the repository/repositories and accession number(s) can be found below: www.osti.gov.
JoM is first author and corresponding author. She interpreted the results and wrote the manuscript. HA provided guidance and contributed to the manuscript. JiM, JoM, AM, and KR participated in the experiments. CW, KR, and AB provided guidance on the experiments and interpretation. KR was the PI on the project at Oak Ridge National Laboratory and AB the PI on the project at Moltex Canada.
Funding was provided by the United States Department of Energy ARPA-E Meitner project in collaboration with Moltex Energy Canada, and the United States Department of Energy Nuclear Energy Advanced Reactor Technology Molten Salt Reactor Licensing and Regulatory Development Campaign. Neutron radiography used resources at the CG1D Beamline a the ORNL High Flux Isotope Reactor, a United States DOE Office of Science User Facilities operated by ORNL.
This manuscript has been authored by UT-Battelle, LLC, under contract DE-AC05-00OR22725 with the United States Department of Energy (DOE). The United States government retains and the publisher, by accepting the article for publication, acknowledges that the United States government retains a nonexclusive, paid-up, irrevocable, worldwide license to publish or reproduce the published form of this manuscript, or allow others to do so, for United States government purposes. DOE will provide public access to these results of federally sponsored research in accordance with the DOE Public Access Plan (http://energy.gov/downloads/doe-public-access-plan).
The authors declare that the research was conducted in the absence of any commercial or financial relationships that could be construed as a potential conflict of interest.
All claims expressed in this article are solely those of the authors and do not necessarily represent those of their affiliated organizations, or those of the publisher, the editors and the reviewers. Any product that may be evaluated in this article, or claim that may be made by its manufacturer, is not guaranteed or endorsed by the publisher.
Andrews, H. B., McFarlane, J., Chapel, A. S., Ezell, N. D. B., Holcomb, D. E., De Wet, D., et al. (2021). Review of molten salt reactor off-gas management considerations. Nucl. Eng. Des. 385, 111529. doi:10.1016/j.nucengdes.2021.111529
Andrews, H. B., McFarlane, J., and Myhre, K. G. (2022). Monitoring noble gases (Xe and Kr) and aerosols (Cs and Rb) in a molten salt reactor surrogate off-gas stream using laser-induced breakdown spectroscopy (LIBS). Appl. Spectrosc. 76 (8), 988–997. doi:10.1177/00037028221088625
Dogel, S., Nattland, D., and Freyland, W. (2003). Wetting transitions in fluid Kx-KCl(1-x) revisited. Z. fuer Phys. Chem. 217 (7), 879–892. doi:10.1524/zpch.217.7.879.20390
Dolan, K., Zheng, G., Sun, K., Carpenter, D., and Hu, L.-W. (2021). Tritium generation, release, and retention from in-core fluoride salt irradiations. Prog. Nucl. Energy 131, 103576. doi:10.1016/j.pnucene.2020.103576
Farías Hermosilla, M. E., and Albesa, A. G. (2020). Monte Carlo simulations of simple gases adsorbed onto graphite and molecular models of activated carbon. Adsorption 26, 1301–1322. doi:10.1007/s10450-020-00254-z
Field, P. E., and Shaffer, J. H. (1967). The solubilities of hydrogen fluoride and deuterium fluoride in molten fluorides. J. Phys. Chem. 71 (10), 3218–3222. doi:10.1021/j100869a013
Frederix, E. M. A. (2022). Estimates of noble metal particle growth in a molten salt reactor. Colloid Surf. A 655, 130167. doi:10.1016/j.colsurfa.2022.130167
Gallagher, R. C., Birri, A., Russell, N., and Ezell, N. D. B. (2022). Design and performance of a variable gap system for thermal conductivity measurements of high temperature, corrosive, and reactive fluids. Int. J. Heat. Mass Tran. 192, 122763. doi:10.1016/j.ijheatmasstransfer.2022.122763
Gao, P., Jin, X., Wang, D., Hu, X., and Chen, G. Z. (2005). A quartz sealed Ag/AgCl reference electrode for CaCl2 based molten salts. J. Electroanal. Chem. 579, 321–328. doi:10.1016/j.jelechem.2005.03.004
Grimes, W. R., Smith, N. V., and Watson, G. M. (1958). Solubility of noble gases in molten fluorides. I. In mixtures of NaF-ZrF4 (53-47 mole %) and NaF-ZrF4-UF4 (50-46-4 mole %). J. Phys. Chem. 62, 862–866. doi:10.1021/j150565a024
Hamilton, H. G., and Inman, D. (1992). Solubility of hydrogen chloride in zinc chloride + sodium chloride melts. J. Chem. Eng. Data 37 (4), 456–458. doi:10.1021/je00008a018
Ignatiev, V., Surenkov, A., Gnidoy, I., Kulakov, A., Uglov, V., Vasiliev, A., et al. (2013). Intergranular tellurium cracking of nickel-based alloys in molten Li, Be, Th, U/F salt mixture. J. Nucl. Mat. 440 (1–3), 243–249. doi:10.1016/j.jnucmat.2013.05.001
Janz, G. J., Tomkins, R. P. T., Allen, C. B., Downey, J. R., Gamer, G. L., Krebs, U., et al. (1975). Molten salts: Volume 4, part 2, chlorides and mixtures—electrical conductance, density, viscosity, and surface tension data. J. Phys. Chem. Ref. Data 4, 871–1178. doi:10.1063/1.555527
Lee, J. J., Arregui-Mena, J. D., Contescu, C. I., Burchell, T. D., Katoh, Y., and Loyalka, S. J. (2020). Protection of graphite from salt and gas permeation in molten salt reactors. J. Nucl. Mat. 534, 152119. doi:10.1016/j.jnucmat.2020.152119
Librovich, B. V., Nowakowski, A. F., Nicolleau, F. C. G. A., and Michelitsch, T. M. (2017). Non-equilibrium evaporation/condensation model. Int. J. Appl. Mech. 09 (8), 1750111. doi:10.1142/S1758825117501113
McFarlane, J., Del Cul, G. D., Massengale, J. R., Mayes, R. T., Robb, K. R., and Sulejmanovic, D. (2022). Chloride salt purification by reaction with thionyl chloride vapors to remove oxygen, oxygenated compounds, and hydroxides. Front. Chem. Eng. 4, 811513. doi:10.3389/fceng.2022.811513
McFarlane, J., Weber, C. F., Greenwood, M. S., and Qualls, A. L. (2018). Thermochemical and transport properties important to molten salt reactor operation: Off-gas performance and the fission product mechanistic source term. Oak Ridge, Tennessee: Oak Ridge National Laboratory. ORNL/TM-2018/958.
Medina, A. S., Felmy, H., Vitale-Sullivan, M., Branch, S., Bryan, S., Lines, A., et al. (2022). Iodine and carbonate species monitoring in molten NaOH-KOH eutectic scrubber via dual-phase in situ Raman spectroscopy. ACS Omega Artic. ASAP, Oct. 28, 40456–40465. doi:10.1021/acsomega.2c05522
Moon, J., Andrews, H., Agca, C., Bilheux, J.-C., Braatz, A., McAlister, A., et al. (2022b). Density measurements of various molten sodium, magnesium, potassium, and uranium chloride salt compositions using neutron imaging, industrial and engineering chemistry research. Ind. Eng. Chem. Res. 61 (48), 17665–17673. doi:10.1021/acs.iecr.2c02967
Moon, J., McFarlane, J., Robb, K., McAlister, A., Braatz, A., Taylor, Z., et al. (2022a). Measuring the solubility of xenon in molten chloride salt, ORNL/TM-2022/1. Oak Ridge, Tennessee: Oak Ridge National Laboratory.
Numata, H., and Bockris, J. O’M. (1984). Interactions of gases in molten salts: Carbon dioxide and oxygen in cryolite alumina melts. Metall. Trans. B 15 (1), 39–46. doi:10.1007/BF02661061
Price, T. J., Chvala, O., and Taylor, Z. (2020). Xenon in molten salt reactors: The effects of solubility, circulating particulate, ionization, and the sensitivity of the circulating void fraction. Nucl. Eng. Technol. 52, 1131–1136. doi:10.1016/j.net.2019.11.026
Rivera, J. L., Starr, F. W., Paricaud, P., and Cummings, P. T. (2006). Polarizable contributions to the surface tension of liquid water. J. Chem. Phys. 125 (9), 094712. doi:10.1063/1.2345063
Roy, S., Liu, Y., Topsakal, M., Dias, E., Gakhar, R., Phillips, W. C., et al. (2021). A holistic approach for elucidating local structure, dynamics, and speciation in molten salts with high structural disorder. J. Am. Chem. Soc. 143 (27), 15298–15308. doi:10.1021/jacs.1c06742
Schrier, E. E., and Clark, H. M. (1963). Interaction in salt vapors and activity coefficients in the potassium chloride-magnesium chloride system. J. Phys. Chem. 67, 1259–1263. doi:10.1021/j100800a023
Sprouster, D. J., Zheng, G., Lee, S. C., Olds, D., Agca, C., McFarlane, J., et al. (2022). Molecular structure and phase equilibria of molten fluoride salt with and without dissolved cesium: FLiNaK–CsF (5 mol %). ACS Appl. Energy Mat. 5 (7), 8067–8074. doi:10.1021/acsaem.2c00544
Stach, H., Janchen, J., Thamm, H., Stievitz, E., and Vetter, R. A. (1986). Influence of the pore diameter on the adsorption behavior of nonpolar molecules on SiO2-adsorbents. Adsorpt. Sci. Technol. 3 (4), 261–270. doi:10.1177/026361748600300407
Stepanov, V. P., Smirnov, M. V., Khokhlov, V. A., Rychkov, V. P., Obrosov, V. P., and Gornova, G. N. (1989). Physicochemical principles for trapping nonferrous metallurgy dusts by salt melts. III. Study of operation of the rotary cyclone-type high-temperature gas-cleaning apparatus. Rasplavy 3, 89–91.
Stepanov, V. P. (2018). Wetting of a charged surface of glassy carbon by molten alkali-metal chlorides. Russ. J. Phys. Chem. A 92 (3), 570–574. doi:10.1134/s0036024418030287
Sokolova, I. D., and Voskresenskaya, N. K. (1966). The surface tension of molten salts, Russ. Chem. Rev. 35 (7), 500–510. doi:10.1070/RC1966v035n07ABEH001491
Tomkins, R. P. T. (2003). “Solubility of gases in molten salts and molten metals,” in The experimental determination of solubilities. Editors G. T. Hefter, and R. P. T. Tomkins (West Sussex, England: John Wiley & Sons), 173–217.
Watson, G. M., Evans, R. B., Grimes, W. R., and Smith, N. V. (1962). Solubility of noble gases in molten fluorides. In LiF-BeF2. J. Chem. Eng. Data 7 (2), 285–287. doi:10.1021/je60013a038
Keywords: chloride molten salt reactor, Xe and Ar solubility, NaCl-MgCl2 molten salt, manometric solubility measurement, neutron imaging
Citation: McFarlane J, Andrews HB, McAlister AL, Moon J, Robb KR, Weber CF and Ballard A (2023) The effect of interfacial phenomena on gas solubility measurements in molten salts. Front. Energy Res. 10:1102466. doi: 10.3389/fenrg.2022.1102466
Received: 18 November 2022; Accepted: 23 December 2022;
Published: 06 January 2023.
Edited by:
Joshua Schlegel, Missouri University of Science and Technology, United StatesReviewed by:
Luteng Zhang, Chongqing University, ChinaCopyright © 2023 McFarlane, Andrews, McAlister, Moon, Robb, Weber and Ballard. This is an open-access article distributed under the terms of the Creative Commons Attribution License (CC BY). The use, distribution or reproduction in other forums is permitted, provided the original author(s) and the copyright owner(s) are credited and that the original publication in this journal is cited, in accordance with accepted academic practice. No use, distribution or reproduction is permitted which does not comply with these terms.
*Correspondence: Joanna McFarlane, bWNmYXJsYW5lakBvcm5sLmdvdg==
Disclaimer: All claims expressed in this article are solely those of the authors and do not necessarily represent those of their affiliated organizations, or those of the publisher, the editors and the reviewers. Any product that may be evaluated in this article or claim that may be made by its manufacturer is not guaranteed or endorsed by the publisher.
Research integrity at Frontiers
Learn more about the work of our research integrity team to safeguard the quality of each article we publish.