- 1CNOOC Research Institute Co. Ltd., Beijing, China
- 2School of Civil and Resource Engineering, University of Science and Technology, Beijing, China
In the production process of low permeability condensate gas reservoir, the reverse condensate action and stress sensitivity will lead to the decrease of reservoir permeability and gas well productivity. However, there are few studies on the effect of retrograde condensation and stress sensitivity on permeability. In this study, the stress-sensitive experiments using the method with constant confining pressure but variable inner pressure were carried out on three cores from the BZ gas field in southwestern Bohai Sea. The test result that use the nitrogen as the experimental fluid represent the effect of core skeleton damage on reservoir permeability during formation pressure drop, and the test result that use the condensate gas as the experimental fluid represent the effect of core skeleton damage and retrograde condensation on reservoir permeability. The results reveal that when the formation pressure drops to the dew point pressure, retrograde condensation damage is the main cause of permeability decline. And the effects of core skeleton damage will increase as the formation pressure drops and exceed the retrograde condensation damage when the net stress is greater 7 MPa. When the net stress reaches 20 MPa, the core skeleton damage accounts for more than 90% of the total damage. Furthermore, the gas well production was calculated considering stress sensitivity and retrograde condensation based on the experimental results, the result shows that gas well production decreases by 97.65% when retrograde condensation is considered. This study can provide a reference for quantitative evaluation of the retrograde condensation and stress sensitivity in the production process of condensate gas reservoir.
Introduction
In recent decades, the development of unconventional reservoirs, including condensate reservoirs, has attracted increasing attention as the global demand for oil and gas has increased. Among different gas reservoirs, the condensate gas reservoir is special and complex. Due to the existence of condensate and retrograde condensate in a certain temperature and pressure range, gas and condensate can be produced simultaneously in the process of gas reservoir exploitation, which has extremely high economic value (Chen, 2016; Hekmatzadeh and Gerami, 2018). Condensate gas reservoirs are important for improving the efficiency of the oil industry in China and the world because of their rich oil and gas reserves (Xu et al., 2017; Yang et al., 2021).
For conventional oil and gas reservoirs, the reservoir pressure continuously decreases during the development process while the overlying pressure remains unchanged, leading to increasing net stress in the reservoir. As a result, the structural deformation of the core occurs, resulting in changes in core porosity, permeability, and other parameters (Settari et al., 1989; Buchsteiner et al., 1994). This phenomenon is called the stress-sensitive effect. According to Davies and Davies, 2001, stress sensitivity is caused by particle slip motion, particle deformation, and particle fracturing in the core. Geertsma, 1957 argued that the decrease in overlying pressure could cause an increase in core pore volume. Tian et al. (2015) conducted stress-sensitive experiments by changing the back pressure. The results suggested that the inner compressive stress caused by back pressure variation acts directly on the skeleton particles of rock pores. Jiao et al. (2011) carried out the stress-sensitive experiments using the method with constant internal pressure but variable peripheral pressure. The result shows that the process of rock deformation could be divided into two phases, namely the microcrack closure phase and the rock compression phase. For condensate reservoirs, Bentsen, 1998 found that when the internal pressure of the reservoir drops below the dew point pressure of condensate gas, retrograde condensation occurs. Some scholars found that when retrograde condensation occurs, a part of condensate oil is attached to the surface of the larger pores of the reservoir rocks in the form of a liquid film, reducing the gas-phase permeation channels, and the other part of condensate oil is in the form of liquid droplets at the smaller pore throats, blocking the originally connected flow channels and causing the effective permeability of the gas phase to decrease. Zhu et al. (2007) found that the condensate oil is easy to accumulate in the small pores in the pore and on the surface of rock particles, and the condensate oil gradually evolves to form slug after aggregation. Song and Li, 2005 analyzed the mechanism of the phenomena of the streaming along the pore-wall with condensate oil. The streaming along the pore-wall with condensate oil can be divided into three layers: adsorbed oil, bound oil and outer oil. In addition, more retrograde condensation leads to more pore space occupied by condensate oil before the saturation reaches the critical point. The higher decrease rate of gas effective permeability eventually causes a decrease in the recovery of condensate (Barnum et al., 1995; Liu et al., 2001; Shi et al., 2006; Zhu and Huang, 1992). Fevang and Whitson, 1997 and Mott, 2003 found that when bottom flow pressure drops below the dew point, an area of high condensate saturation forms near the wellbore, resulting in reduced gas permeability. Zhang et al. (2020) carried out the experimental test of pollution damage of retrograde condensate. The result show that the retrograde condensate pollution will lead to a significant reduction of gas permeability and when the formation pressure drops to 7 MPa, the gas permeability is only 1/6 of that in the early stage of development. Wang et al. (2018) found that condensate gas reservoirs during natural development have persistent condensate blockage, and the core permeability decreases with decreasing pressure. Several experimental studies have been conducted on the retrograde condensation damage of gas reservoirs (Feng et al., 2020; Li et al., 2004; Tong et al., 2004) However, the influence of retrograde condensation and stress sensitivity on reservoir permeability and its changing pattern during pressure changes need to be explored.
In this study, nitrogen and condensate gas were used as experimental fluids to conduct the stress-sensitive experiments on cores of three permeabilities. The percentages of core skeleton damage and retrograde condensation damage during the rise of net stress are determined based on the experimental results. The influence of the two types of damage on the decrease of permeability and the change law are analyzed, and the equation of gas well production is established considering stress sensitivity and retrograde condensation. This study aims to analyze the influence of retrograde condensation and stress sensitivity and summarize the change law of core skeleton damage and retrograde condensation damage on reservoir permeability in the production process.
Experimental samples and methods
Core parameter
The core samples (Figure 1) used in the experiment were taken from the BZ gas field in southwestern Bohai Sea, the largest metamorphic buried hill condensate gas field in the Bohai Sea. BZ gas field is characterized by diverse reservoir types, extremely high condensate content, and great stress sensitivity. The buried depth is 3,400∼5,600 m, the formation temperature is 158.4∼198°C, the condensate content is 711∼751 g/m3, the porosity is 8.7% and the permeability is 0.3∼4 mD. The basic parameters of each core are shown in Table 1. Moreover, the mineral composition of core samples was tested, and the test results are listed in Table 2.
Condensate gas parameters
The condensate gas used in the experiments was prepared based on PVT data from the gas field. The fluid composition distribution, fluid phase, and condensate gas precipitation are shown in Table 3; Figures 2, 3, respectively. Data in Figure 2 was experimentally obtained with collected oil and gas samples in the PVT cylinder, reflecting the percentage of oil and gas in the mixture under certain temperature and pressure conditions. It can be found that the critical temperature of the field is 52.4°C, the critical condensation temperature is 384.8°C, and the formation temperature is 152°C. The critical pressure is 40.76 MPa, the critical condensate pressure is 45.74, and the formation pressure is 46.93 MPa. Figure 3 shows the variation of condensate saturation with pressure in a constant volume decay experiment of the formation fluid. It can be seen that the dew point pressure of this gas field is 43.97 MPa, and the condensate saturation is maximum at 23 MPa, which is 32.31%.
Experimental principles and methods
Rock stress-sensitive experiments can be divided into two types: one with variable confining pressure and constant inner pressure, and the other with constant confining pressure and variable inner pressure. Considering that the overburden pressure of the reservoir does not change during the actual exploitation and the internal pressure gradually decreases with the production, the measurement method of fixed circumferential pressure and variable internal pressure is more consistent with the practical production (Guo et al., 2007). In this study, the stress sensitivity experiment method of constant confining pressure and variable inner pressure was adopted. The confining pressure of the core gripper was kept unchanged, and the back pressure was gradually decreased to change the net stress of the core, thus altering the core permeability. Finally, the stress sensitivity of the core was obtained by calculating the core permeability at each pressure point. The experimental setup is shown in Figure 4. The main experimental devices include an injection pump, a core holder, a confining pressure pump, an intermediate container, a differential pressure gauge, a back pressure value, and a separator. In addition, the maximum working pressure of the injection pump is 150 MPa, and the working pressure range of the core holder, the confining pressure pump, the intermediate container and the back pressure value is 0–70 MPa.
The stress-sensitive experiments were divided into two groups. Nitrogen was used as the experimental fluid in the first group of stress-sensitive experiments, and condensate gas was used in the second group. In order to facilitate the control of the experimental group and match the actual situation, the experimental process only simulated the stress sensitivity during the rise of the net stress. The specific operation steps are as follows: 1) The experimental equipment is connected, and the dried core is loaded into the gripper and heated to the actual formation temperature. 2) The initial confining pressure is 3 MPa. The inlet pressure and the back pressure are equal and slowly increased with the confining pressure at a step of 2 MPa and an interval of 30 min until the confining pressure rose to 50 MPa. The inlet pressure and back pressure rise to the original formation pressure. 3) The confining pressure and inlet pressure are kept constant, and the back pressure value is reduced step by step according to the setback pressure point. The permeability under each back pressure point is measured when the gas seepage is stable. 4) After measuring all the set pressure points, the source valve is closed to end the experiment.
The experimental results
Stress sensitivity of cores with nitrogen as the flow medium
The stress-sensitive test results of the three cores are shown in Figure 5. The permeability retention rate (i.e., the ratio of permeability to initial permeability after core deformation) is basically the same for the three cores at different net stresses. It can be seen that the permeability rate gradually decreases with the increase of the net stress. When the net stress increases from 2.5 MPa to 20 MPa, the permeability decreases rapidly, and the permeability retention rate of all three cores decreases to less than 20%. As the net stress increases from 20 MPa to 45 MPa, the decreasing of permeability slows down, and the permeability retention rate of the three cores decreases by about 10%. Finally, the permeability retention of all three cores decreases to less than 10%. When the net stress is 45 MPa, the permeability retention rate is 6.89% for BZ-1, 9.26% for BZ-2, and 9.41% for BZ-3. When the net stress is less than 20 MPa, the permeability significantly decreases with the rise of effective force. When the net stress is greater than 20 MPa, the decrease of permeability slows down, indicating that at the initial stage of net stress increase, the core is compressed, the internal structure is deformed and destroyed, and the change of permeability is significant. When the net stress increases to 20 MPa, the variation degree of the core structure stabilizes, and the permeability retention rate remains unchanged. Because the mineral composition of cores from the same formation is basically the same, the deformation ability and degree of different permeability cores are similar, and the variation curves at different permeability show the same trend.
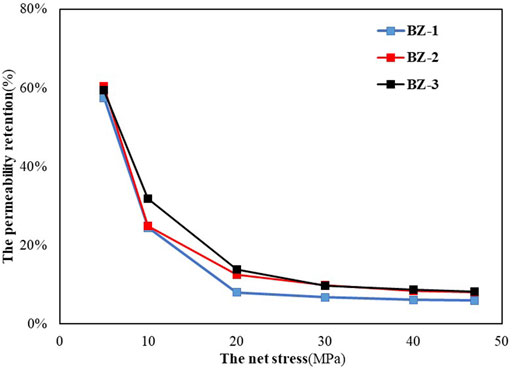
FIGURE 5. Relationship curves between the permeability retention and net stress at the different net stresses.
Stress sensitivity of cores with condensate gas as the flow medium
The stress-sensitive test results of the three cores are shown in Figure 6. When the net stress increases, the permeability decreases rapidly, and the permeability retention rate of all three cores decreases to less than 10%. When the net differential pressure increases from 30 MPa, the permeability retention rate also increases. When the net stress reaches 45 MPa, the permeability retention rate of all three cores decreases to less than 10% (6.85% for core BZ-1, 8.04% for core BZ-2, and 7.8% for core BZ-3). When the net stress increases to 5 MPa, the permeability retention rate rapidly decreases to less than 10%. According to Figure 3, the pressure drops below the dew point pressure of 43.97 MPa when the net stress increases to 5 MPa. At this time, retrograde condensation occurs, and the saturation of condensate oil rapidly increases. After the formation pressure drops by 3 MPa, the oil saturation in the core reaches about 30%, causing pore throat blockage. This situation keeps aggravating and leads to a rapid drop in permeability. When the net differential pressure reaches 30 MPa, the permeate retention rate increases with the increase of the net differential pressure. When the net stress reaches 20 MPa, the pore throat blockage decreases, and the permeate rate increases.
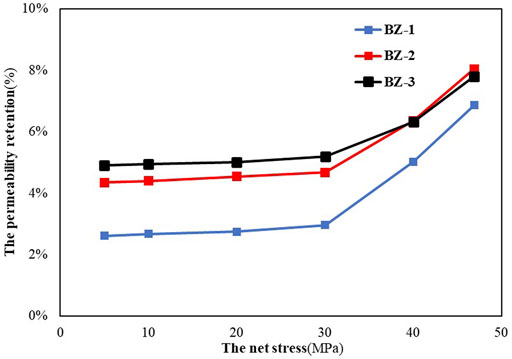
FIGURE 6. Relationship between the permeability retention and net stress at the different net stresses.
Core skeleton damage and retrograde condensation damage discrimination
The experimental fluid used in the first group was nitrogen, and the stress-sensitive damage factor in the experiment can be considered as only core skeleton damage. In the second group, condensate gas was used as the experimental fluid. During the experiment, retrograde condensation occurs in the core with the change of the net stress, producing condensate oil. The factors causing the decrease in permeability can be regarded as a combination of core skeleton damage and retrograde condensation damage. By comparing the damage at the same permeability level in the two sets of experiments, the percentage of the total damage caused by retrograde condensation damage and core skeleton damage can be obtained:
where Dst is the percentage of damage caused by the core skeleton (%); Da is the permeability retention rate under experimental nitrogen conditions (%); Db is the permeability retention rate under experimental conditions of condensate gas (%).
where Dcon is the percentage of damage caused by condensate (%).
According to Eqs. 1, 2, the percentage of retrograde condensation damage and core skeleton damage of the three cores were obtained, as shown in Figure 7. It can be seen that the damage percentage change of the three cores shows the same trend. When the net stress is 5 MPa, the percentage of retrograde condensation damage is greater than that of core skeleton damage. However, as the net stress increases, the percentage of retrograde condensation damage gradually decreases, and the core skeleton damage gradually increases. Finally, the percentage of core skeleton damage exceeds 90%, while the percentage of retrograde condensation damage is less than 10%. With a smaller core permeability, the percentage of core skeleton damage is smaller, and the percentage of retrograde condensation damage is larger.
According to the variation curve, retrograde condensation damage and core skeleton damage can be divided into three stages (Figure 8). Stage 1 occurs before the net differential pressure reaches 7 MPa. At this stage, the pressure drops below the dew point, and some component phases in the condensate gas become condensate oil, rapidly occupying the core pore throat. However, the core skeleton is not broken. The retrograde condensation damage is the main factor leading to the decrease in permeability. Stage 2 is between 7MPa and 20 MPa. The oil saturation of the core stabilizes at about 30%, while the degree of deformation and damage of the core structure increases, resulting in a higher percentage of core skeletal damage than the percentage of retrograde condensation damage. In stage 3, the percentage of each damage stabilizes after 20 MPa. After 20 MPa, the core skeleton damage accounts for about 90% of the net pressure drop and tends to be stable. Therefore, the core skeleton damage can be considered the main cause of the permeability drop, while the proportion of reverse retrograde condensation damage is smaller.
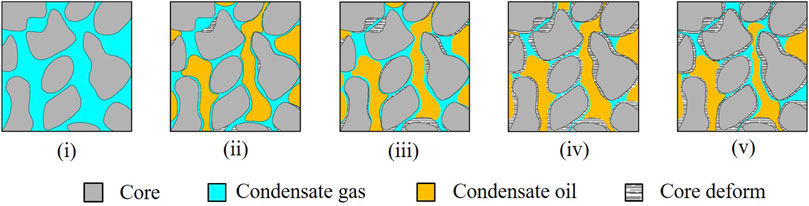
FIGURE 8. Five stages of the impact of retrograde condensate and stress sensitivity on the permeability of the reservoir during production: (i)The initial stage; (ii) The leading stage of retrograde condensate; (iii) The joint action stage; (iv) The leading stage of core skeleton damage; (v)The final stage.
According to the experimental results, the impact of retrograde condensate and stress sensitivity on the permeability of the reservoir during production can be divided into five stages: 1) The initial stage: In this state, the formation pressure is greater than the dew point pressure, there is only condensate gas in the core, and the core skeleton does not change; 2) The leading stage of retrograde condensate: When the formation pressure drops to the dew point pressure, the condensate gas condensate phenomenon occurs, condensate oil occupies the core pore throat, resulting in a rapid decline in core permeability, and the core skeleton is not broken; 3) Joint action stage: when the formation pressure drops between 5 and 7 MPa, the core skeleton begins to broken. At this stage, the retrograde condensation damage and core skeleton damage jointly lead to the decrease of reservoir permeability; 4) The leading stage of core skeleton damage: when the formation pressure drops to 7 MPa, the degree of deformation and damage of the core structure increases, while the condensate content in the core remains unchanged, and core skeleton damage is the main reason for the decrease of permeability; 5) Final stage: After the formation pressure drops by 20 MPa, the degree of deformation and damage of the core structure is maximum and condensate oil content remain unchanged, and the permeability remains stable.
The impact of condensation on capacity
The experimental results can be used to fit the equation for calculating gas well production. Moreover, gas well production under different bottom flow pressures can be obtained to evaluate the degree of condensation damage.
Penetration rate calculation formula
Permeability calculation requires the consideration of stress sensitivity and condensate oil. The variation of condensate saturation is related to the variation of formation pressure. By fitting the condensate saturation and pressure curves to Figure 3, the following equation can be obtained:
where Sw(p) is the condensate oil content saturation (%); p is the formation pressure (MPa); a, b, c, d, and e are the coefficients.
Based on the results of stress-sensitive experiments with condensate gas as the experimental fluid and Eq. 1, the equation for calculating the permeability is fitted considering the stress-sensitive case where the phase occurs in condensate gas:
where k is permeability (mD); k0 is the initial permeability (mD); pe is the initial pressure (MPa); p0 is the test pressure (MPa); a, b, and c are the coefficients.
Calculation of gas well production
To facilitate the modeling and solution requirements, the following assumptions need to be made: 1) Rocks and stratigraphic fluids are compressible, and the compression coefficient is constant; 2) Percolation of gases in the reservoir obeys Darcy’s law; 3) The effects of capillary forces and gravity on seepage is neglected; 4) No change in temperature of the reservoir; 5) The strata are homogeneous and isotropic. Under stable production conditions, the production equation for gas wells can be expressed as follows:
where Qsc is the gas well production (m3/d); Zsc is the gas compression coefficient under standard conditions; Tsc is the gas temperature at standard conditions, (K); Z is the gas compression coefficient of the reservoir; T is the reservoir temperature (K); psc is the reservoir pressure (MPa); r is the radius of the well (m); h is the thickness of the reservoir (m); p is the bottom flow pressure (MPa); k is the reservoir permeability (mD); μ is the fluid viscosity (mPas).
By substituting Eq. 4 into the gas production equation and integrating, the gas production equation considering stress sensitivity and condensate gas phase is obtained:
Gas well production calculation
Based on the actual reservoir conditions, the parameters are given in Table 4, and the gas well production is calculated for different bottom flow pressures with and without retrograde condensation.
The calculated results are shown in Figure 9. It can be seen that the production increases as the bottom flow pressure decreases. When the flow pressure at the bottom of the well is 0 MPa, the gas well production without retrograde condensation decreases by 97.65%, suggesting that the precipitation of condensate gas seriously affects gas well production. However, this equation is a steady-state production equation, and the calculation process does not consider the impact of production time on capacity, which has certain limitations.
Conclusion
In this study, the change of retrograde condensate damage and core skeleton damage during pressure drop was analyzed through the stress-sensitive experiment with nitrogen and condensate gas as the experimental fluid. According to the experimental results, the calculation formula of gas well production considering the phase change of condensate gas was derived. The following conclusions were made:
(1) The results of stress-sensitive experiments using nitrogen as the experimental fluid show that when the net stress is less than 20 MPa, the core structure is deformed and damaged, resulting in a decrease in permeability. When the net stress exceeds 20 MPa, the structural damage and permeability change tend to be stable. The results of stress-sensitive experiments using condensate gas as the experimental fluid show that after the net stress increases to 5 MPa, the condensate gas undergoes a phase change, and condensate oil rapidly occupies the pore throat, resulting in a rapid decrease in rock permeability. When the net stress reaches 20 MPa, the condensate saturation decreases, the pore throat blockage slows down, and the permeability increases.
(2) The discrimination between core skeleton damage and retrograde condensation damage indicates that the damage can be divided into three stages. At Stage 1 (the net stress is less than 7 MPa), the core skeleton damage accounts for about 35%, and the retrograde condensation damage accounts for about 65%. At Stage 2 (the net stress is greater than 7 MPa and less than 20 MPa), the percentage of core skeleton damage gradually increases from 35% to 70%–80%, and the percentage of retrograde condensation damage decreases to 20%–30%. At Stage 3 (the net stress is greater than 20 MPa), two kinds of damage tend to stabilize, in which the core skeleton damage accounts for about 90%, and the percentage of retrograde condensation damage is about 10%.
(3) Because core skeleton damage and retrograde condensate damage have different sensitivity to pressure changes, the main damage of each stage is different. After the pressure drops below the dew point, part of the component phase in the condensate gas becomes condensate oil, the core skeleton does not break the ring to a large extent, suggesting that retrograde condensation damage is the main factor contributing to the decrease in permeability in stage 1. At stage 2, the degree of phase transformation of condensate gas stabilizes, while the degree of deformation and damage to the core structure increases. The percentage of core skeleton damage starts to increase and exceeds the percentage of retrograde condensation damage. At stage 3, the deformation and failure degree of the core skeleton stabilize when the net stress reaches 20 MPa, with little change in the percentage of both types of damage.
(4) According to the experimental results, the equation considering stress sensitivity and condensate gas phase transformation is obtained to calculate gas well production. The calculation results show that the production decreases by 97.65% when the phase transformation is considered. The phase transformation of condensate gas seriously affects the exploitation of condensate gas reservoirs, leading to a significant decrease in production.
Data availability statement
The raw data supporting the conclusion of this article will be made available by the authors, without undue reservation.
Author contributions
NL, XT, and LZ contributed to conception and design of the study. HL and YL performed the numeral calculations and statistical analysis. NL, XT, LZ, HL, and YL wrote sections of the manuscript. All authors contributed to manuscript revision, read, and approved the submitted version.
Funding
This work was supported by the CNOOC Group Major Project (Grant No. KJGG 2022-0700 and Grant No. YXKYZX 07 2021). The authors declare that the funder was not involved in the study design, collection, analysis, interpretation of data, the writing of this article, or the decision to submit it for publication.
Conflict of interest
NL, XT, and LZ were employed by CNOOC Research Institute Co. Ltd.
The remaining authors declare that the research was conducted in the absence of any commercial or financial relationships that could be construed as a potential conflict of interest.
Publisher’s note
All claims expressed in this article are solely those of the authors and do not necessarily represent those of their affiliated organizations, or those of the publisher, the editors and the reviewers. Any product that may be evaluated in this article, or claim that may be made by its manufacturer, is not guaranteed or endorsed by the publisher.
References
Barnum, R. S., Brinkman, F. P., Richardson, T. W., and Spillette, A. G. (1995). Gas condensate reservoir behaviour: Productivity and recovery reduction due to condensation. Spe Tech. Conf. Exhib. 32 (3), 451–456. doi:10.2118/30767-MS
Bentsen, R. G. (1998). Effect of momentum transfer between fluid phases on effective mobility. J. Pet. Sci. Eng. 21 (1-2), 27–42. doi:10.1016/S0920-4105(98)00035-7
Buchsteiner, H., Warpinski, N. R., and Economides, M. J. (1994). Stress-induced permeability reduction in fissured reservoirs. SPE 265113. doi:10.2118/26513-MS
Chen, C. (2016). Multiscale imaging, modeling, and principal component analysis of gas transport in shale reservoirs. Fuel 182, 761–770. doi:10.1016/j.fuel.2016.06.020
Davies, J. P., and Davies, D. (2001). Stress-dependent permeability:characterization and modeling. SPE J. 6, 224–235. 56813. doi:10.2118/71750-PA
Feng, Q., Deng, B., Yang, Y., Jia, J., Peng, X., and Yuan, J. (2020). Evaluations and removing methods of the retrograde condensate damage of the gas condensate reservoirs in the tight sandstone. Petroleum Geol. Oilfield Dev. Daqing 39 (02), 139–146. doi:10.19597/J.ISSN.1000-3754.201905014
Fevang, Ø., and Whitson, C. H. (1997). Modeling gas-condensate well deliverability. SPE Reserv. Eng. 1997 (04), 221–230. doi:10.2118/30714-PA
Geertsma, J. (1957). The effect of fluid pressure decline on volumetric changes of porous rocks. Trans. AIME 210, 331–340. doi:10.2118/728-g
Guo, P., Xu, Y., Chen, Z., Jiang, Y., and Pang, Y. (2007). New ideas obtained from laboratory study of flowing mechanisms in low-permeability reservoirs. Nat. Gas. Ind. 2007 (07), 86–88+140-141. doi:10.3321/j.issn:1000-0976.2007.07.025
Hekmatzadeh, M., and Gerami, S. (2018). A new fast approach for well production prediction in gas-condensate reservoirs. J. Petroleum Sci. Eng. 160, 47–59. doi:10.1016/j.petrol.2017.10.032
Jiao, C., He, S., and Xie, Q. (2011). An experimental study on stress-dependent sensitivity of ultra-low permeability sandstone reserviors. Acta Pet. Sin. 32 (03), 489–494. doi:10.7623/syxb201103018
Li, J., Li, X., Tong, M., Cheng, S., and Kang, X. (2004). Study on a new method for liquid phase transformation of condensate gas in porous media. Petroleum Explor. Dev. 2004 (06), 101–103. doi:10.3321/j.issn:1000-0747.2004.06.027
Liu, Y., Yuan, S., Song, W., Li, B., and Wang, J. (2001). Mechanism study of condensate influencing on productivity. Petroleum Explor. Dev. 2001 (01), 54–56+12-4+3. doi:10.3321/j.issn:1000-0747.2001.01.018
Mott, R. (2003). Engineering calculations of gas-condensate-well productivity. SPE Reserv. Eval. Eng. 6 (5), 298–306. doi:10.2118/86298-PA
Settari, A., Kry, P. R., and Yee, C. T. (1989). Coupling of fluid flow and soil behaviour to model injection into uncemented oil sands. J. Can. Petroleum Technol. 28 (1). doi:10.2118/89-01-08
Shi, D., Li, X., and Liu, Y. (2006). Deliverability equation study of gas condensate well considering phase change. Oil Drill. Prod. Technol. 2006 (04), 68–70+85-86. doi:10.3969/j.issn.1000-7393.2006.04.021
Song, Y., and Li, Z. (2005). To analyze the mechanism of the phenomena of the streaming along the pore-wall with condensate oil. Nat. Gas. Geosci. 2005 (02), 214–215. doi:10.3321/j.issn:1000-0976.2005.09.030
Tian, W., Zhu, W., Zhu, H. Y., Zhang, X. L., Wang, R. M., and Yong, L. I. (2015). Study on testing method of back-pressuress sensitivity evaluation. Nat. Gas. Geosci. 26 (2), 377–383. doi:10.11764/j.issn.1672-1926.2015.02.0377
Tong, M., Li, X., Hu, Y., Wang, J., and Li, J. (2004). Experimental study on influence of porous media on phase behavior of gas condensate. J. Xi'an Shiyou Univ. Sci. Ed. 2004 (05), 61–64. doi:10.3321/j.issn:1000-5870.2004.05.013
Wang, Z., Zhu, S., Zhou, W., Liu, H., Hu, Y., Guo, P., et al. (2018). Experimental research of condensate blockage and mitigating effect of gas injection. Petroleum 4 (3), 292–299. doi:10.1016/j.petlm.2018.03.008
Xu, C., Zou, H., Yang, Y., Yong, D., Yang, S., Luo, B., et al. (2017). Status and prospects of exploration and exploitation of the deep oil and gas resources onshore China. Nat. Gas. Geosci. 28 (8), 1139–1153. doi:10.11764/j.issn.1672-1926.2017.07.014
Yang, X., Tian, J., Wang, Q., Li, Y., Yang, H., Tang, Y., et al. (2021). Geological understanding and favorable exploration fields of ultra-deep formations in Tarim Basin. China Pet. Explor. 26, 17–28. doi:10.3969/j.issn.1672-7703.2021.04.002
Zhang, C., Song, X., and Tang, Y. (2020). Experimental study on retrograde condensate damage of Longfengshan extra-low porosity and extra-low permeability condensate gas reservoir. J. Xi'an Shiyou Univ. Sci. Ed. 35 (02), 50–53+97. CNKI:SUN:XASY.0.2020-02-009.
Zhu, W., and Huang, Y. (1992). A description of microscopic flowing mechanisms of a gas-liquid condensate through porous media. Petroleum Explor. Dev. 1992 (01), 45–48+108-109. CNKI:SUN:SKYK.0.1992-01-006.
Keywords: condensate reservoirs, stress sensitivity, retrograde condensation damage, production calculation, gas well
Citation: Li N, Li H, Tan X, Zhang L and Liu Y (2023) Effect of retrograde condensation and stress sensitivity on properties of condensate gas reservoirs. Front. Energy Res. 10:1078755. doi: 10.3389/fenrg.2022.1078755
Received: 24 October 2022; Accepted: 23 November 2022;
Published: 03 January 2023.
Edited by:
Fuyong Wang, China University of Petroleum, Beijing, ChinaReviewed by:
Hu Guo, China University of Petroleum, Beijing, ChinaYouwei He, Southwest Petroleum University, China
Copyright © 2023 Li, Li, Tan, Zhang and Liu. This is an open-access article distributed under the terms of the Creative Commons Attribution License (CC BY). The use, distribution or reproduction in other forums is permitted, provided the original author(s) and the copyright owner(s) are credited and that the original publication in this journal is cited, in accordance with accepted academic practice. No use, distribution or reproduction is permitted which does not comply with these terms.
*Correspondence: Yuwei Liu, NzgyMzQzMzEyQHFxLmNvbQ==