- 1China Electric Power Research Institute Co., Ltd., Nanjing, China
- 2College of Electrical Engineering, Zhejiang University, Hangzhou, China
- 3State Grid Fujian Electric Power Research Institute, Fuzhou, China
Transactive energy systems (TESs) combine both economical and control mechanisms, and have become promising solutions to integrate distributed energy resources (DERs) in modern power systems. This article will introduce the basic concept of a TES, including its definition, process, time scale, and benefits. The configuration of the TES is then described in detail from the perspectives of the physical system, information system, transaction system, and regulatory system. The transaction mechanism allows participants (e.g., customer, generator, transmission operator, marketer, etc.) to conduct various transactions with any other party to the extent that regulatory policy permits. Transactive control is regarded as one of the most advanced approaches to realize the full response potential of flexible devices and respect the end user’s privacy, preference, and free will. Finally, some challenges to the development of TESs that arise from the limitations of current equipment level and methodological concepts will be discussed. In summary, TESs provide a more efficient, fair, and transparent environment for participants to promote DERs utilization, improve market efficiency, and increase economic benefits.
1 Introduction
The energy industry is currently at a critical juncture of transition. Many changes are taking place in the power system—such as, increasing complexity of power grids, growing penetration of renewable generations, and proliferating distributed energy resources (DERs)—, which lead to an increased requirement for efficiency, reliability, security, economic, and environmentally sustainable (Moslehi and Kumar, 2010; Barrager and Cazalet, 2014; Lezama et al., 2019). Consequently, the operation and regulatory models in generation-based power systems find it difficult to deal with these issues at both bulk-power and distribution levels (Rahimi et al., 2016).
Demand side management (DSM) refers to the energy management of the demand side, using effective incentives or other suitable measures to improve the efficiency of terminal electricity consumption, both for economic profits and grid reliability. DSM was at first utility driven but more attention has been paid to the customer driven approach in recent years (Mohsenian-Rad et al., 2010; Palensky and Dietrich, 2011; Yan et al., 2021). Demand response (DR) describes the changes in electricity usage by end users from their normal consumption patterns in response to changes in the price of electricity over time and was first classified into two main types: incentive-based and price-based (Albadi and El-Saadany, 2008). The incentive-based method is a centralized mechanism that provides users with an incentive (or compensation) from top to bottom. In the price-based method, users respond to the market price in a one-way communication. In addition, the price-based approach is the basis for the development of the market-based mechanism. Hence, the electricity market has developed rapidly due to the excess of power, and therefore, a third type called the transaction-based or market-based approach has emerged in the last few years.
As an expansion form of DR, transactive energy manages both supply and demand sides simultaneously (Chen and Liu, 2017). Transactive energy (TE) is a new market-based energy management approach to cope with the increasing demand for DERs and smart devices (Chang et al., 2017), which is a decentralized mechanism. In addition, unlike the price-based mechanism, transactive energy systems (TESs) pay more attention to the two-way interaction between the market and users, and the combination of the market mechanism and control mechanism. The term “transactive energy” was first proposed by the GridWise Architecture Council (GWAC), which was established by the US Department of Energy (DOE) in May 2004 to realize the combination of economic and control mechanisms improving grid efficiency and reliability (Melton, 2015). TES is a more efficient, fair, and transparent version, which proposes innovative and disruptive technologies to facilitate energy efficiency, storage, DERs, and renewable resources.
TE research pays attention to intelligent agent-based innovation in smart building equipment and local demonstrations involving operators, energy suppliers, consumers, prosumers, marketers, and regulators (Melton, 2015). Implementations in the United States and Europe have shown that TESs are feasible and high-potential mechanisms, particularly in three major demonstration projects that were performed by the US DOE partnered with several organizations. The first project of TES conceptual implementation was the GridWise Olympic Peninsula Demonstration (2005–2007), which was located in Washington state (Ambrosio, 2016). Its initial purpose was to test the potential for taking advantage of DERs to postpone or reduce the need for a transmission upgrade. The market mechanism used 5-min double auctions to coordinate over 100 homes, a marine science laboratory, and four large water pumps. This project established multiple objectives, such as reducing system peak load, managing distribution operations, and saving energy costs to explain the viability of TE (Kok and Widergren, 2016). In support of the American Reinvestment and Recovery Act (ARRA) of 2009, a group of smart grid field demonstrations was built across the United States (Hammerstrom et al., 2016a). Based on the Olympic Peninsula project, the AEP Ohio Real-Time Pricing gridSMART demonstration project (2010–2014) used a double-auction market mechanism in a real-time price (RTP) model to dispatch participating responsive loads (Pratt, 2012; Kok and Widergren, 2016). The results showed that the bills of wholesale buyers were reduced by around 5%, and its data were used to calibrate simulated DR models that searched for participants in higher penetration levels. The Pacific Northwest Smart Grid Demonstration (PNWSGD, 2010–2015) involved several states and electric utilities, comprising around 60,000 end users (Ambrosio, 2016), (Huang et al., 2010; Jin et al., 2012). A TES was implemented to coordinate the operation of DERs and regional objectives across 11 utilities to mitigate the intermittency of renewable generations and flatten system load, and showed the viability of DERs that were dynamically integrated on a large scale (Kok and Widergren, 2016).
TE is becoming one of the more novel and interesting approaches to the grid of the future (Olken, 2016). TESs provide an effective and healthy transaction environment for participants who wish to buy and sell energy using automated control. As a solution to deal with the growing penetration of highly uncertain renewable energy, TES has great potential in mobilizing demand response to integrating DERs in more effective, fair, and transparent ways.
2 Basic concept
2.1 Definition
A TES is defined by the GWAC as “a system of economic and control mechanisms that allows the dynamic balance of supply and demand across the entire electrical infrastructure using value as a key operational parameter” (Melton, 2015). This definition seems to be broad, given that it considers both the existing use of current techniques and the potential use of new techniques.
The following 11 attributes (shown in Table 1) are defined for two reasons: to present a broader view of TE and to provide common approaches to implement TES (Melton, 2015).
The necessity to define the principles of TES was discussed during the GWAC workshop in Philadelphia (February 2014). Six principles were defined, as follows (Transactive Energy Principles (V1.0), 2022):
1) Implement some form of highly coordinated self-optimization. This principle explains the efficient operation of TE.
2) Maintain system reliability and control while enabling optimal integration of renewables and DERs. This shows the high consumption capacity of renewables and DERs.
3) Provide for non-discriminatory participation by qualified participants. TE is committed to constructing a fair transaction milieu.
4) Observable and auditable at interfaces. Transparency and supervision are the sources of trust for TE customers.
5) Scalable, adaptable, and extensible across several devices, participants, and geographic extents. The high degree of extensibility is a strong boost to TE’s sustainable development.
6) Transacting parties are accountable for standards of performance. Rich information can be sought in this principle, including the hint of distributed operation, the respect for personal privacy, and the improvement of system efficiency.
In short, these six principles demonstrate that TE operates in an efficient, fair, and transparent manner, realizing the efficient market and the full potential of demand response.
2.2 Process and time scale
Three actors in TES are related to the transaction process: energy buyers, energy sellers, and marketers. Based on the market price, buyers submit bids to purchase energy considering their costs and preference, while sellers make tenders to sell energy by maximizing their profits. A transaction will be established when their requirements are matched. The bids and transactions can be made either in exchanges or bilaterally. In addition, transaction platforms are virtualized as software applications on the “cloud”. Marketers are responsible for managing transactions and reducing the imbalance between energy demand and supply. With large-scale, frequent, and efficient transactions, the market price will gradually stabilize, and balance supply and demand.
The process in TES is dynamic. A buyer has a position after being transacted in the market. If something better happens or their energy needs to change, then the process will repeat until a better position is found for the buyer. The same process is used for sellers. The transaction can continue until delivery time.
The key objective of system operators is to provide reliable and secure power to loads, thereby avoiding power outages. Actions will take place in the period from milliseconds to years to guarantee power reliability. With the development of emerging trends, operations will gradually shift to shorter periods. This means that human control may not be sustainable, and therefore, automated and intelligent control with manual supervision will be needed.
The system will also become harder to operate by manual control because of the growing number of data sources, communication spots, and control points. The time frame of TE may have a large period from minutes (real-time transaction) to a day (day-ahead transaction), or even years (forward transaction). A span from minutes to a few hours is regarded as the most critical timeframe where demand response works effectively.
3 System configuration
As was performed for the topic of grid interoperability with the publication of the “GridWise Interoperability Context Setting Framework,” the GWAC created the TE framework to provide a common ground and facilitate further development of TESs (Forfia et al., 2016). This is defined as a shared tool to spread and serve smart communities (e.g., smart grid).
There are four main systems in the TE model: physical system, information system, transaction system, and regulatory system. The physical system includes energy generation, storage, transmission, and distribution, together with the end user’s smart devices. The information system is built to collect, process, organize, and transmit information, both in the power system and energy markets. The transaction system manages various types of energy transactions to establish an effective energy market. The regulatory system safeguards against economic abuse and rule violation. It also maintains market safety and reliability. TES offers a method to ensure the reliability and security of the power system while improving efficiency by coordinating a growing number of DERs. A brief configuration of TES is shown in Figure 1.
3.1 Physical system
3.1.1 Inherit and develop current systems
TES can be implemented with a few modifications (depending on the size and complexity) of the current physical systems. Communication and information technology (CIT) is needed to build the physical system of TES. The cyber-physical elements of the grid also need to be developed for further support of TES with new sensors, actuators, and control elements. The current physical systems and devices need to be developed to realize information collection and automation more flexibly than required for operating the traditional grid. Features such as information exchange, data dealing, system decoupling, and interactions are needed to enhance flexibility.
3.1.2 Smart connections
The connection and communication technology required for TE is in place due to the fast, universal, and wireless Internet. The Internet enables two-way communication between all entities connected to the TE Platform. All devices can get decision-related information on the Internet, such as weather, emergency warnings, and market signals. Wireless Internet or Wi-Fi enables different devices to communicate with each other. Humans, data, and devices can be connected to various places with access to the Internet. In addition, Wi-Fi completes the link from the TE Platform to EMS to smart appliances. TES communicates over the Internet and connects the TE platforms with different parties through TE Interface and EMS, which can be a device in a building or an application in the cloud.
3.1.3 Smart devices
Devices are becoming smarter thanks to the fast development of automation technology and artificial intelligence technology. In addition, more devices related to renewables are being deployed, driven by the growing demand for clean energy. Many appliances equipped with Wi-Fi capability are available nowadays. For example, smart clothes dryers can be closed at a high electricity price (or by the EMS instruction), and smart water heaters can adjust heat power depending on the price signals (Barrager and Cazalet, 2014). The control strategy of devices aims to coordinate the end-user’s comfort and energy cost. Smart devices apply advanced technologies, including the ability of wireless communication with other devices throughout the building and the Internet. Devices such as heating, ventilation, air conditioning (HVAC), and electric vehicles can access the TES directly. EVs can make decisions considering the current and predicted charging or discharging price send in the charging/discharging market of EVs.
Smart meters, EMS, Wi-Fi, and smart devices are in place to build TES. In addition, smart buildings (e.g., commercial buildings, smart homes, and factories) are also prepared for TES implementation. Consequently, smart energy and the smart city will become a reality in the near future.
3.2 Information system
Information interoperability is an important element of TES. It not only needs to clear the valuation and process of transactions but also needs to understand operation mechanisms and the control approaches both in the TES and the grid. Therefore, the concept of information interoperability is directly related to both the transaction and operation models.
3.2.1 Information models
The Transactive Energy Market Information Exchange (TeMIX) is a framework and protocol for spot and forward transactions, which are based on standards. Transactions can then be made automatically on a large scale and at high speed with smart meters, Internet communications, smart applications, and TeMIX protocols (TeMIX, 2010). TeMIX supports decentralized control or decision-making at the edges of the grid. End-user applications (e.g., HVAC, EVs, distributed photovoltaic generation, and storage) are automatically transactive with distribution grid devices. Therefore, TeMIX implements a smart grid, quickly adapting to the high penetration of renewables, EVs, and distributed storage.
Four information models have been defined for the transactional energy model (Cazalet, 2022): energy transaction, energy offer, energy option transaction, and energy options offer. Energy transaction or energy offer is easy to understand. Energy option transaction means that there is an option for an energy transaction and energy options offer, which refers to an offer for an energy option transaction. The information model is mainly used for the effective exchange of energy transaction information. In addition to spot transactions, customers can make forward transactions at high or low prices using energy option transactions, which are similar to capacity and ancillary services. Energy options can also be energy contracts such as demand response contracts. The elements of the four information models are described in Table 2.
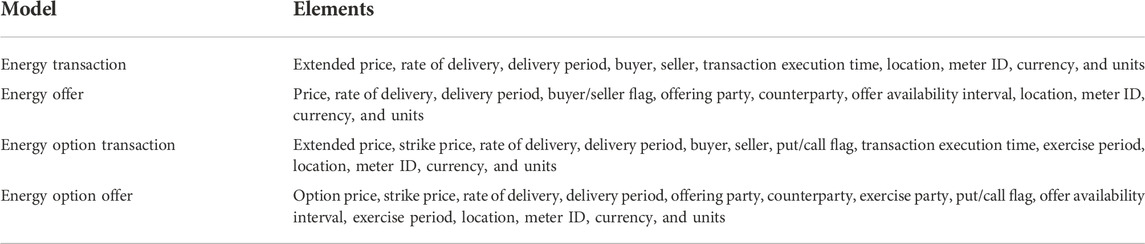
TABLE 2. Elements of the four information models (Cazalet, 2022).
3.2.2 Information security
The integration of ICT with the traditional transmission infrastructure may lead to new vulnerabilities. Although the standards and technologies of information security have improved dramatically over the past decades, they still need to be adjusted to meet the unique requirements of the power system and the continued evolving capabilities of hackers. Information disruption can be costly because a great number of services on the grid may be destroyed by exploiting security weaknesses.
3.3 Transaction system
3.3.1 Transaction parties
The transaction parties contain energy suppliers, consumers, energy transmission owners, and intermediaries (Barrager and Cazalet, 2014). Both energy suppliers and consumers are energy service parties, including power producers, consumers, prosumers, and storage owners. Marketers, retailers, exchangers, and system operators are all intermediary entities to provide transaction services and energy management. Transmission and distribution owners are also encouraged to participate in the transactive platform.
3.3.2 Transaction platform
As shown in Figure 2, the transaction platforms aggregate four transaction parties to build transactions and provide energy services. The exchanges are associated with the transactive platform, which is associated with the EMS of the grid. The intermediaries also connect with their customers and the exchanges through the platform. The intermediaries support a more active market to small-scale customers making transactions, as well as taking transaction risks for others. For example, some intermediaries may purchase long-term forward positions and sell them closer to delivery time.
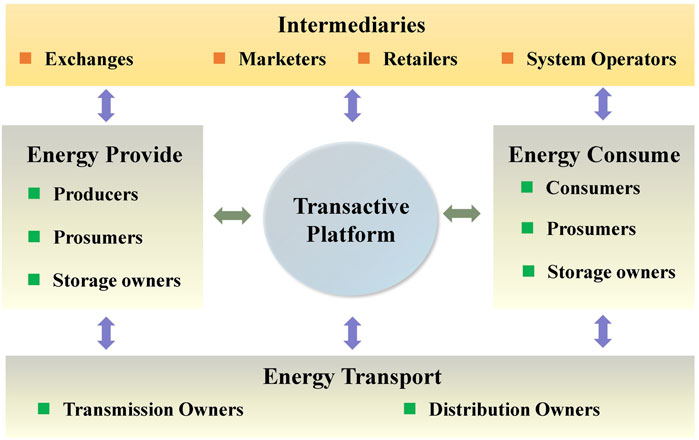
FIGURE 2. Transactive platform diagram (Barrager and Cazalet, 2014).
3.3.3 Transaction activities
Marketers play an important role in market mobility by adjusting the market price. First, several small forward bids will be posted by marketers with small price differences. The tenders will then expire after a short period. If counterparties accept larger amounts of buy bids than sell bids during this time, then the marketer will reduce the prices of the next bids to lower net positive positions (or raise the prices in net negative positions). The energy markets will be driven toward equilibrium through the iterative process. Some activities of the transaction process in TES are shown in Table 3.
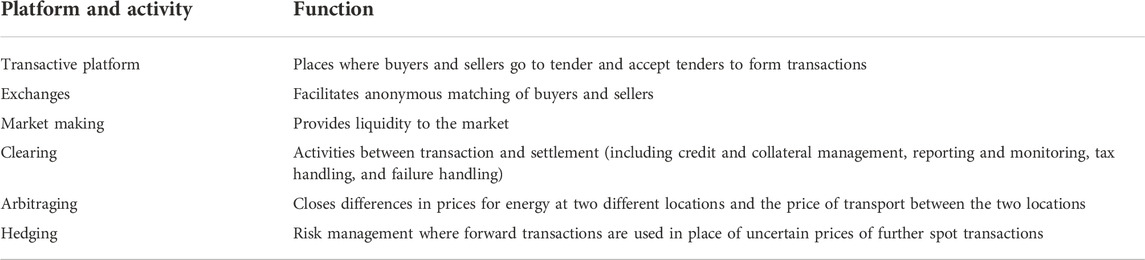
TABLE 3. Activities in the transactive platform (Barrager and Cazalet, 2014).
In addition to providing market mobility, marketers also reduce transaction costs and facilitate trade through price differentials in markets. The marketers should be independent parties that are licensed and regulated. They are not allowed to manipulate the market, either for their own profit or for others’ profits.
3.4 Regulation system
Regulators will always play an important role in maintaining power system security, competition fairness, and protecting the health of the markets. Regulators are also responsible for ensuring rules in TES and fighting against economic abuses. TES can reduce the workload of economic regulators and the requirement for oversight on price setting. Market prices for customers are impartial thanks to forward and spot transaction processes under the supervision of a regulation system. Consequently, regulation ensures that TES is essentially fair and transparent.
The regulation system of TES requires three regulatory framework elements (Kristov et al., 2016). The first key element is to ensure open access and TES principles that are applied to the interconnection process, infrastructure projects, operating procedures, and market transactions. Second, states should be allowed to manage markets for distribution system operators (DSOs) and DSO operations within their jurisdiction. The purpose of this element is to regulate energy markets by each state, thereby facilitating widespread adoption across the country. The last key element is the establishment of reliable shared responsibilities between the transmission system operator (TSO) and DSO. The regulation for DSO needs to assign responsibility and accountability for reliable service to end users in ways that go beyond reliable distribution services (which may vary from state to state).
3.5 Operation modes and value realization
3.5.1 Operation modes
In TES, different operation parties can operate in four modes as transactive agents (Rahimi et al., 2016), as follows: 1) operating autonomously based on the preferences of customers, 2) responding to bilateral transactive bids and offers, 3) responding to market price signals made by marketers, and 4) responding to operator instructions. The first two modes have the least constraint on prosumers but may influence the reliability of the power system. The schedule is not needed to be known before the operation in the first mode, while it is determined in other modes. The operation mode can be transformed from one to another depending on the situation of entity status, time, environment, or system conditions. The autonomous operation, bilateral transactive response, and market price response would prevail in the TES configuration.
In the DSO-based operation, the DSO will finalize a bilateral transaction as an operational schedule to be conveyed to the transacting parties. The DSO’s instructions may replace the operation mode of some devices in some emergency conditions (e.g., priority plans established through the electronic tag). Sometimes, the DSO dispatches operation license signals to a subset of registered transactive agents, depending on the state of the grid or the electricity price (Moazeni and Defourny, 2017). Whether the ability of the prosumer to transition from one mode to another will be restricted or not depends on the TES protocols that are made by relevant local, state, or other regulatory entities. The interactions among different agents— including the DSO, consumers, prosumers, storages, utility distribution companies (UDCs), microgrids, DER providers, regional renewable providers, and the bulk-power markets—are shown in Figure 3.
3.5.2 Value realization
3.5.2.1 Value streams
With growing access to information, customers have higher requirements for reliability and quality of energy service, as well as eco-friendly choices and lower costs. In addition, customers can gain new revenue opportunities and value streams by providing services to the grid through innovative market design and regulatory policies. Sometimes, TE also can be seen as valve-based energy management (Makhmalbaf et al., 2017). Melton (2015) shows that 30 value streams (which include both buck-power and distribution systems) can be considered by different parties to provide energy services and make their own objections.
3.5.2.2 Alignment of value streams
TES proposes an opportunity for utilities and third parties to offer value-added services to energy customers, and also extend them beyond regulatory coverage. However, viable transactive approaches need to introduce opportunities to build and target value streams for all of the participants. In addition, the value achieved by the grid or customer utility should not be at the cost of end users, TSOs, or generators. The alignment of value streams makes a great contribution to establishing multi-objective optimization models that combine both operational and economic factors. Although they may not support the value creation and alignment by current business models, they can be adjusted when necessary and new business models may emerge during the process.
4 Transaction mechanism
Transactive markets allow different entities to transact with each other, as far as the regulatory policy allows. Both generators and customers can take the double role of energy selling and consumption compared to a simple buyer or seller role in the past.
4.1 Transaction classification
4.1.1 Forward transaction and spot transaction
Two kinds of decisions are made in the electricity ecosystem: investment and operation. Forward transactions, which can be made by bilateral contracts or on exchanges, are used to coordinate investment decisions and manage risk. Spot transactions, which can be built on an ISO/DSO platform, are used to coordinate operating decisions and mitigate risk.
Forward transactions in TES are used to coordinate investments throughout the electricity ecosystem. Consumers, prosumers, and DERs owners use transactive platforms to develop forward transactions in a fair competitive environment. Investors can manage price and cost risk using forward transactions. Long-term contracts (a form of forwarding transactions) are used by power producers to reduce their current risk. Customers in retail markets may install assets such as PV panels or other efficient appliances to manage the risks of investments.
Spot transactions in TES are employed to coordinate operating decisions. All of the parties have access to the same spot transaction exchanges in the same game rules. However, in the current electricity market, spot transactions are often developed by large wholesale or industrial customers to coordinate system operations, and few are used by small customers. Investment and operation are autonomous in spot transactions. Participants decide what to invest in and how to operate it, and their autonomous decisions are coordinated on the same transactive platforms.
4.1.2 Wholesale transaction and retail transaction
The wholesale market relies on bids and offers to issue delivery orders. When the system constraint is not satisfied by economic bids and offers, then administrative measures will be taken to reject certain transactions or arrange resources outside the market to deal with system constraints (Rahimi and Albuyeh, 2016). The TES paradigm extends the current wholesale market to retail markets, including consumers, prosumers, DERs owners, and various smart devices.
DSO is created (not only) for the following two motivations: the first is to integrate DERs in a market environment to obtain economic benefits, and the second is to establish a market environment to incentivize customers, utilities, and third parties to invest in DERs. In both cases, it is necessary to organize markets and manage system reliability, in addition to transactive with wholesale markets. The presentations on motivation for DSOs specify a significant difference between the wholesale markets (at the ISO level) and the retail markets (at the DSO level) (Masiello and Aguero, 2016).
The retail market is not a new concept, and has been demonstrated and applied in many countries, such as Europe and the United States (Nair and Nair, 2016). There is a tendency to deregulate the electricity markets in many countries. However, the lesson learned from organizational insolvency in California shows that over-deregulation of the retail market may lead to higher electricity costs for customers and improper commercial intervention by competing service operators. TES proposes and encourages distributed control approaches rather than traditional centralized optimized control. TES is an effective correction for the deregulated market. The energy is extended to be traded by the retail market on account of the distributed control mechanism in TES. As a result, small-scale energy resources in DSM can be effectively used and uncertain renewables can be greatly consumed. The relationships between the wholesale market and the retail market are shown in Figure 4 (supported by TeMIX).
4.1.3 Bilateral auction
To make a bilateral auction, buyers submit their bids while sellers send their offers at the same time. They then each compete to get the best price. In this kind of auction, the ideal transaction price is the equilibrium price corresponding to the value at the intersection point of the supply curve and the demand curve (Xavier et al., 2017). The bilateral auction is one of the most popular models studied in the electricity market. It is also widely used in day-ahead and hourly auctions, which can be seen in the UK or the US electricity markets.
A bilateral auction is a feasible solution to handle resource distribution problems in different regions. In addition, an auction can be seen as a fair, effective, and eco-driven scheme. Consequently, several research efforts have been devoted to considering auction behaviors in the resource distribution of the grids (Li et al., 2017). By taking advantage of the potentialities of blockchain technologies, energy exchanges and auctions can be operated automatically in the transactive market (Kounelis et al., 2017).
4.2 Local market designs
This section introduces two local market designs: the first is the direct peer-to-peer (P2P) market and the second is the closed-order book market. The closed-order book market is slow in transaction speed and takes a long time to confirm the order, which is suitable for larger time scales. In comparison, transactions in the P2Pmarket are faster and more flexible, and therefore, the P2P market can be used in shorter time scales.
4.2.1 Order book market
The order book market is designed as a double-auction market with discrete market closing times (Mengelkamp et al., 2017). An energy order book is an electronic list of buying and selling orders for energy transactions, and is dynamic and continually updated. In the order book market, the clearing price is the uniform settlement price for each trading period. The bid and offer bills of customers are gathered and ordered. The bids are sorted by descending order and the offers are sorted by ascending order. When the energy supply is given, the clearing price in the market is influenced by all of the bid/offer prices, even the lowest bid price. In an order book market, customers who successfully booked their energy orders pay the bills for the procured electricity at the market clearing price.
4.2.2 Peer-to-peer market
The P2P network uses a decentralized and distributed network architecture. The individual nodes in the P2P network perform as both suppliers and consumers of energy resources. In contrast to the centralized client-server model, client nodes request access to resources provided by central servers (Barrager and Cazalet, 2014). P2P trading represents direct energy transactions between peers; for example, a direct transaction between consumers and/or prosumers is a simple example of a P2P transaction (Zhang et al., 2018). In the P2P market, customers transact with each other in a randomized based on a payment order. At each session, each buyer is paired with sellers randomly and iteratively until all of the electricity is procured or all of the potential sellers have been matched. A prosumer is paired with the consumer when the bid price of the consumer is higher or equal to the offer price. The matching amount is determined to be the minimum amount offered by the seller and the buyer. The P2P market with intelligent agents may seem to be the best market mechanism because it has the lowest average electricity price of all transactions and the highest participation enthusiasm of end users (Mengelkamp et al., 2017).
4.3 Price mechanism
There are two types of market price: the first is the local marginal price (LMP) and the second is the uniform market price (UMP) (Albadi and El-Saadany, 2008). In a TE environment, distribution locational marginal price (DLMP) is the basis for energy pricing among transactive market participants (Sajjadi et al., 2016). Long-term DLMPs are mainly used to stimulate investments, while short-term DLMPs are used for market clearing and energy balancing in real-time markets or day-ahead markets (Rahimi et al., 2016). The communication mechanism that sends market price signals to smart devices of end users can help to transfer wholesale LMPs to DLMPs considering the transmission losses and operation costs. In a transactive market, the market signals are sent to end users through software nodes, and each node is responded with a prospective energy requirement based on the market signal (Chandler et al., 2014).
4.4 Stakeholder responsibilities
The clear definition of the stakeholder responsibilities is one of the key elements to realize the bulk-power recombination in the past (Rahimi et al., 2016). It is also an indispensable step to establish a novel structural framework for all of the transaction and operation parties to clear the responsibilities of each stakeholder. For instance, the system operators are responsible for balancing energy between supply and demand, managing transmission congestion, and reducing (or stopping) transactions in urgent situations. They also have the right to get transmission and scheduling information in their jurisdiction. The main responsibility of marketers/retailers is to manage energy transactions. Marketers or retailers make suitable market prices, both for forward transactions and spot transactions, and send market signals to the end users’ devices (or show them on the Internet). Together with the regulators, they are responsible to enable a healthy and effective market ambience. The customers should be responsible for the transactions that they made and obey the transaction formal process and rules (a breach of contract may be punished).
4.5 Transactive control
4.5.1 Category of EMS
As shown in Table 4, energy management approaches can be classified into four main categories (Kok and Widergren, 2016): top-down switching, centralized optimization, price reaction, and transactive control in a distribution-level energy management system.
Top-down switching, like direct load control (DLC) (Xie et al., 2018), is the earliest and most straightforward energy management approach of DR. Although recently implemented in different countries, it does not take full advantage of the end users’ responses. Price reaction (PR) uses price signals that are sent by marketers to change the end users’ electricity consumption. However, the customers are not allowed to send their preferences to marketers or system operators because it is one-way communication. Although the centralized optimization (CO) approach is also a centralized control approach, it takes a large amount of information (both power system and energy customers) into account through two-way communications. However, the communication and optimization times grow nonlinearly as the number of end users increases. Price reaction and centralized optimization can realize the full use of demand response potential. However, the two approaches also have some constraints. For the former, the consumer’s reaction to each price signal is uncertain due to the lack of user’s preferences and the state of the device. The latter one has privacy issues because the exchanged information involves the details of devices and individual behaviors of the end users.
Transactive control (TC) is a distributed control strategy that uses self-interested responsive loads to provide energy services to the grid based on market mechanisms (Hao et al., 2017). The energy quantities and market price signals are the entire information need to be exchanged. Therefore, compared to PR, TC is much more efficient in market mechanisms with no privacy problems. Moreover, TC displays much higher scalability than the CO approach, due to its distributed structure. In short, the TE approach realizes the full use of the potential of flexible devices, provides greater certainty about the instantaneous system response, gives appropriate incentives to achieve effective markets, and respects the end users’ privacy, preference, and free will.
4.5.2 Transactive control strategies
Transactive control will provide a means to coordinate the response of smart devices at all levels of the power system (Melton and Hammerstrom, 2012). Based on TC, each stakeholder is allowed to have their own set of optimal control strategies based on the market mechanism and are accountable for their standards of performance. The application of TE has been studied in renewables integration, EV management and integration, efficient and intelligent management for microgrids, commercial buildings and smart houses, and other aspects. These TC strategies and modeling may look unique on different occasions but in fact the difference primly lies in the diversification of objective function and constraint—the core is still the optimization control strategy under the market mechanism using price and energy quantities as key parameters.
4.5.2.1 Renewables
The use of renewable energy resources is increasing, due to the strong support of many governments. For example, the European Union has a renewable portfolio standard (RPS) of 20% by 2020 and the United States 20% by 2030 (Sahin and Shereck, 2014). As the proportion of renewable energy generation grows, which replaces more traditional energy resources, concerns about the reliability and security of the grid increase. More approaches are needed to properly account for integrating renewables on the grid—and TC is a suitable solution. Kiani and Annaswamy (2012) and Kiani Bejestani et al. (2014) present a hierarchical TC architecture, which considers market transactions at both the lower levels (unit-level control) and the higher levels (inter-area control). A hierarchical control framework is described in Hansen et al. (2017), which is used to coordinate DERs and demand response.
4.5.2.2 Electric vehicles and storage
The use of EVs has become more popular over the past few years because they are environmentally friendly, cost effective, and highly efficient. However, the reliability of the power system is greatly challenged by large-scale access of EVs, especially in power distribution systems (Galvan et al., 2016). This issue can be subtly solved by transactive control, which is an advanced control approach that is supported by an increasing amount of smart devices that use ICT (Lopes et al., 2011; Hu et al., 2015; Behboodi et al., 2016; Hu et al., 2017a; Divshali et al., 2017). Although storage cost has been reduced considerably, storage technology is still expensive. Therefore, there are still risks in investing in storage equipment (Parandehgheibi et al., 2017). Building distributed, small-scale energy storage is one way to reduce investment risk. Therefore, approaches and mechanisms to aggregate small energy storage need to be studied.
4.5.2.3 Microgrids
In the past few decades, extensive research has been conducted to develop energy efficient operation strategies for microgrids, which can be classified into two categories: the first is individual microgrid operation (Amin et al., 2016; Patterson and Geary, 2016; Prinsloo et al., 2016; Prinsloo et al., 2017; Yao and Zhang, 2017) and the second is multiple interconnected microgrid operation (as microgrid clusters) (Chen and Hu, 2016; Baron-Prada et al., 2017; Nunna and Srinivasan, 2017; EduardoCeseña et al., 2018). Microgrid clusters and individual operation both have advantages and constraints. Microgrid clusters can significantly reduce energy costs and improve resilience ability. However, most of the existing operation models for microgrid clusters have difficulty ensuring individual interests because they focus on collective interests (Yang, 2018).
4.5.2.4 Smart buildings
The energy consumption of buildings has accounted for more than 40% of whole consumption in the United States since 2010, which is higher than the transportation industry and industrial sector by 44% and 36%, respectively (Chen and Hu, 2016). Recently, the growth of smart building development has provided an opportunity for transactive systems to provide grid services. In addition, the potential value of TES for smart buildings extends beyond the grid services (and may include other energy services, not only electricity services) (Hammerstrom et al., 2016b). The transactive behavior of buildings can be classified into the following categories: interior of the building, different buildings, and building to the grid. Aggregation and coordination of commercial buildings loads (Rahimi and Ipakchi, 2016; Ramdaspalli et al., 2016; Hao et al., 2017) and smart house (residential) loads (Mohsenian-Rad and Leon-Garcia, 2010; Widergren et al., 2014; Dai et al., 2015; Adhikari et al., 2016; Pratt et al., 2016; Siano and Sarno, 2016; Zhou et al., 2016; Ji and Zhang, 2017; Yue et al., 2017; Rayati and Ranjbar, 2018) for TC have drawn attention, and modeling and control strategies have started to emerge.
4.5.2.5 Distributed multi-energy system
Distributed multi-energy systems can flexibly provide a broad range of energy services (EduardoCeseña et al., 2018). A multi-energy system is commonly designed with one or more forms of energy utilization (except electricity), such as gas boilers, combined heat and power (CHP) units, thermal energy storages, and battery energy storages (BES). Oikonomou et al. (2018) presents a comprehensive architecture to improve the participation of water distribution system operators (W-DSOs) in DR and frequency services to gain profit opportunities. A transactive approach has been proposed to the optimal scheduling for prosumers in coupled energy systems (Qiu et al., 2018), where DERs are operated in coordination in the form of a virtual power plant (VPP) (Qiu et al., 2017), which actively take part in the electricity market and the wholesale gas market. Hu et al. (2017b) proposed a network-constrained transactive energy method to schedule EVs and heat pumps in a retailer’s aggregation.
5 Impacts and future directions
5.1 Benefits
5.1.1 Power system benefits
First, TES uses advanced automation and control, which increases the flexibility of microgrids and enhances the local and regional resilience of electric networks (Transactive Energy Infographics, 2022). Second, TES increases interoperability between regional and local markets that coordinates the use of energy resources to improve efficiency and reliability. Third, the strength of the TES lies in the innovative mechanisms that combine the economics of managing buck-power systems with the objectives of coordinated control (Nair and Nair, 2016). Finally, the high cost of system operation, which requires a considerable amount of reserve generation to safeguard against fluctuations caused by intermittent renewable generations (O’Connell et al., 2014), can be significantly reduced by TES through load curtailment and shifting.
5.1.2 Customer benefits
First, customers are offered more choices in the TES. They can freely produce and sell energy and services, as well as purchase energy from multiple sources. Second, opportunities for new services to customers are unlocked by wider information exchange in TES. Therefore, customers are free to choose and enjoy their favorite services. Finally, TES will save customers’ costs over time and reduce the volatility of their bills, which will increase their economic benefits.
5.1.3 Market benefits
First, TES is conducive to attracting market investment. It has significant efficiency profits, both in the forward markets and in the spot markets. Second, market impartiality is regulated and guaranteed by the TES, which provides an efficient, fair, and transparent platform for all participants. Finally, by having more active participation in the market, a significant enhancement in market efficiency may be realized (Siano, 2014). A further benefit is that it may reduce average wholesale prices, as well as the volatility of peak prices.
5.2 Challenges
TES is a complex system that can provide functional and extendible ways to integrate the self-directed resources of participants to achieve the objective of coordinating the interests of the single-user and region. A reasonable solution requires the following issues to be addressed (Kok and Widergren, 2016): 1) privacy and security; 2) standards-formulating; 3) feasible transition; and 4) reliable, stable, and automatic operation.
In addition to these concerns, one of the most challenging for grid related control systems is the shift from a highly centralized control system to a more distributed control system under the TES configuration. The demand side will take a more important role in the power system and diversified distributed control strategies may emerge, both for energy suppliers and energy consumers. As a result, the coordination between the distributed control of end users and the centralized control of the grid is a critical issue that urgently needs to be addressed. There are currently many control methods for communication speed and distributed control. For example, the distributed consensus control algorithm is a high-speed communication method, which is oriented to fully distributed control (Sun et al., 2015; Wang et al., 2015; Qin et al., 2019). In addition, the effectiveness of distributed control algorithm based on the back-and-forth communication framework has also been verified (Yu et al., 2021).
Realizing effective and high-speed communications is a significant challenge for smart meters. This issue may be difficult considering the varying environments and locations of smart meters, especially in remote areas. This situation may be very different between rural areas and urban areas. So far, there are a large number of solutions, such as the use of cell and pager networks, satellite, radios, power line communication, and Internet-related networks (e.g., fixed wireless, mesh network, and Wi-Fi). However, no single solution seems to be optimal for all applications.
5.3 Future directions
New market structures will need to be developed to deal with the uncertainty and intermittent of renewable energy resources, both on a large-scale and distributed level. A cost-effective, high-efficient, fair, and transparent market operation mechanism will need to be built for customers to make their transactions more free, safe, and trusting. In addition, an active supplier community (or technology ecosystem) will need to emerge to provide a healthy supply of transactional energy products and services for customers. Meanwhile, new business and regulatory models should be established in the TES configuration, which will guarantee a fair and transparent transaction environment for all of the participants.
Considering the periodicity of the users’ energy consumption and the uncertainty of new energy output, future research should include a combined multi-time scale transaction control mechanism. This will help to explore the potential of the TES in the construction and development of power market and distributed trading market, and promote the consumption of renewable energy. The emergence of prosumers and distributed renewable energy will bring a large amount of controllable distributed energy to the demand side. Therefore, future research in TES should focus on improving the interaction between flexible resources. In addition, the development of smart cities and smart energy has improved the level of intelligent control of equipment, which will provide a foundation for TES to build a clean, efficient, interactive, and intelligent energy trading community.
TESs may also contain more energy forms, turning into integrated energy systems. The new energy system is dominated by electric energy and supplemented by other energy, including natural gas energy, chilling water resources, and thermal energy resources. The Internet of Things (IoT) will be further developed by implementing TES. Finally, an Energy Internet may emerge in the future.
6 Conclusion
TES, combined with both economic and control mechanisms, provides an effective transactive platform for all transacting parties to transact and communicate with each other. This study introduces the basic concepts, system configuration, and transaction mechanism of TES, which can integrate DERs into the transactive market. By using a TES configuration, an optimal control mechanism for integrated energy systems can be established that considers flexible coupling and the complementarity of multi-energy at the end users’ or regional level. In addition, the benign competition mechanism in the TE market improves the efficiency of the market and establishes a reasonable market price. In the future, TE will play an increasingly important role in energy management and service, promote the establishment of new energy system structures, and will also build new business and regulatory models.
Author contributions
HZ conceptualized the study and wrote part of the originaldraft. BL contributed to the construction of the theoretical research framework, manuscript writing—review and editing. XZ and DC contributed to the revision of the manuscript. All authors have read and agreed to the published version of the manuscript.
Conflict of interest
HZ was employed by China Electric Power Research Institute Co., Ltd.
The remaining authors declare that the research was conducted in the absence of any commercial or financial relationships that could be construed as a potential conflict of interest.
Publisher’s note
All claims expressed in this article are solely those of the authors and do not necessarily represent those of their affiliated organizations, or those of the publisher, the editors, and the reviewers. Any product that may be evaluated in this article, or claim that may be made by its manufacturer, is not guaranteed or endorsed by the publisher.
References
Adhikari, R., Pipattanasomporn, M., Kuzlu, M., and Rahman, S. (2016). “Simulation study of transactive control strategies for residential HVAC systems,” in 2016 IEEE PES innovative smart grid technologies conference Europe (ISGT-Europe) (Ljubljana), 1–5.
Albadi, M. H., and El-Saadany, E. F. (2008). A summary of demand response in electricity markets. Electr. Power Syst. Res. 78 (11), 1989–1996. doi:10.1016/j.epsr.2008.04.002
Ambrosio, R. (2016). Transactive energy systems [viewpoint]. IEEE Electrific. Mag. 4 (4), 4–7. doi:10.1109/mele.2016.2614234
Amin, U., Hossain, M. J., Lu, J., and Mahmud, M. A. (2016). “Cost-benefit analysis for proactive consumers in a microgrid for transactive energy management systems,” in 2016 australasian universities power engineering conference (AUPEC) (Brisbane, QLD), 1–6.
Baron-Prada, E., Osorio, E., and Mojica-Nava, E. (2017). “Resilient transactive control in microgrids under dynamic load altering attacks,” in 2017 IEEE 3rd Colombian conference on automatic control (CCAC) (Cartagena, Colombia, 1–5).
Barrager, S., and Cazalet, E. (2014). Transactive energy: A sustainable business and regulatory model for electricity. Francisco: Baker Street Publishing.
Behboodi, S., Chassin, D. P., Crawford, C., and Djilali, N. (2016). “Electric vehicle participation in transactive power systems using real-time retail prices,” in 2016 49th Hawaii international conference on system sciences (HICSS) (Koloa, HI), 2400–2407.
Cazalet, E. G. (2022). An official white paper of the OASIS EMIX Technical Committee. Available at: http://www.oasis-open.org/committees/download.php/37954/TeMIX-20100523.pdf.
Chandler, S. A., Rinaldi, J. H., Bass, R. B., and Beckett, L. (2014). “Smart grid dispatch optimization control techniques for transactive energy systems,” in 2014 IEEE conference on technologies for sustainability (SusTech), Portland, OR (Portland: OR), 51–54.
Chang, L., Wang, X., and Mao, M. (2017). “Transactive energy scheme based on multi-factor evaluation and contract net protocol for distribution network with high penetration of DERs,” in 2017 Chinese Automation Congress (CAC), Jinan, China, October 20–22, 2017 (IEEE), 7139–7144.
Chen, S., and Liu, C. C. (2017). From demand response to transactive energy: State of the art. J. Mod. Power Syst. Clean. Energy 5 (1), 10–19. doi:10.1007/s40565-016-0256-x
Chen, Y., and Hu, M. (2016). Balancing collective and individual interests in transactive energy management of interconnected micro-grid clusters. Energy 108, 1075–1085. doi:10.1016/j.energy.2016.05.052
Dai, Rui, Hu, Mengqi, Dong, Yang, and Chen, Yang (2015). A collaborative operation decision model for distributed building clusters. Energy 84, 759–773. doi:10.1016/j.energy.2015.03.042
Divshali, P. H., Choi, B. J., and Liang, H. (2017). Multi-agent transactive energy management system considering high levels of renewable energy source and electric vehicles IET Gener. Transm. Distrib. 11, 3713–3721. doi:10.1049/iet-gtd.2016.1916
EduardoCeseña, A. Martínez, Good, Nicholas, Syrri, A. L., and Mancarella, P. (2018). Techno-economic and business case assessment of multi-energy microgrids with co-optimization of energy, reserve and reliability services. Appl. Energy 210, 896–913. doi:10.1016/j.apenergy.2017.08.131
Forfia, D., Knight, M., and Melton, R. (2016). The view from the top of the mountain: Building a community of practice with the GridWise transactive energy framework. IEEE Power Energy Mag. 14 (3), 25–33. doi:10.1109/mpe.2016.2524961
Galvan, E., Mandal, P., Velez-Reyes, M., and Kamalasadan, S. (2016). “Transactive control mechanism for efficient management of EVs charging in transactive energy environment,” in 2016 north American power symposium (NAPS) (Denver, CO), 1–6.
Hammerstrom, D. J., Corbin, C. D., Fernandez, N., Homer, J. S., Makhmalbaf, A., Pratt, R. G., et al. (2016). Valuation of transactive systems. Richland, WA: Pacific Northwest National Laboratory.
Hammerstrom, D. J., Widergren, S. E., and Irwin, C. (2016). Evaluating transactive systems: Historical and current U.S. DOE research and development activities. IEEE Electrific. Mag. 4 (4), 30–36. doi:10.1109/mele.2016.2614182
Hansen, J., Edgar, T., Daily, J., and Wu, D. (2017). “Evaluating transactive controls of integrated transmission and distribution systems using the Framework for Network Co-Simulation,” in 2017 American control conference (ACC) (Seattle, WA), 4010–4017.
Hao, H., Corbin, C. D., Kalsi, K., and Pratt, R. G. (2017). Transactive control of commercial buildings for demand response. IEEE Trans. Power Syst. 32 (1), 774–783. doi:10.1109/tpwrs.2016.2559485
Hu, J., Yang, G., and Xue, Y. (2017). Economic assessment of network-constrained transactive energy for managing flexible demand in distribution systems. Energies 10, 711. doi:10.3390/en10050711
Hu, J., Yang, G., and Bindner, H. W. (2015). “Network constrained transactive control for electric vehicles integration,” in 2015 IEEE power & energy society general meeting, Denver, CO, 1–5.
Hu, J., Yang, G., Bindner, H. W., and Xue, Y. (2017). Application of network-constrained transactive control to electric vehicle charging for secure grid operation. IEEE Trans. Sustain. Energy 8 (2), 505–515. doi:10.1109/tste.2016.2608840
Huang, P., Kalagnanam, J., Natarajan, R., Sharma, M., Ambrosio, R., Hammerstrom, D., et al. (2010). “Analytics and transactive control design for the Pacific Northwest smart grid demonstration project,” in 2010 first IEEE international conference on smart grid communications, Gaithersburg, MD, 449–454.
Ji, M., and Zhang, P. (2017). “Transactive control and coordination of multiple integrated energy systems,” in 2017 IEEE conference on energy Internet and energy system integration (EI2) (Beijing, 1–6).
Jin, D., Zhang, X., and Ghosh, S. (2012). “Simulation models for evaluation of network design and hierarchical transactive control mechanisms in Smart Grids,” in 2012 IEEE PES Innovative Smart Grid Technologies (ISGT), Washington, DC, January 16–20, 2012 (IEEE), 1–8.
Kiani, A., and Annaswamy, A. (2012). “A hierarchical transactive control architecture for renewables integration in Smart Grids,” in 2012 IEEE 51st IEEE conference on decision and control (CDC) (Maui, HI, 4985–4990).
Kiani Bejestani, A., Annaswamy, A., and Samad, T. (2014). A hierarchical transactive control architecture for renewables integration in smart grids: Analytical modeling and stability. IEEE Trans. Smart Grid 5 (4), 2054–2065. doi:10.1109/tsg.2014.2325575
Kok, K., and Widergren, S. (2016). A society of devices: Integrating intelligent distributed resources with transactive energy. IEEE Power Energy Mag. 14 (3), 34–45. doi:10.1109/mpe.2016.2524962
Kounelis, I., Steri, G., Giuliani, R., Geneiatakis, D., Neisse, R., and Nai-Fovino, I. (2017). “Fostering consumers' energy market through smart contracts,” in 2017 international conference in energy and sustainability in small developing economies (ES2DE) (New York, NY: IEEE), 1–6.
Kristov, L., De Martini, P., and Taft, J. D. (2016). A tale of two visions: Designing a decentralized transactive electric system. IEEE Power Energy Mag. 14 (3), 63–69. doi:10.1109/mpe.2016.2524964
Lezama, F., Soares, J., Hernandez-Leal, P., Kaisers, M., Pinto, T., and Vale, Z. (2019). Local energy markets: Paving the path toward fully transactive energy systems. IEEE Trans. Power Syst. 34 (5), 4081–4088. doi:10.1109/tpwrs.2018.2833959
Li, D., Yang, Q., Yu, W., An, D., Yang, X., and Zhao, W. (2017). “A strategy-proof privacy-preserving double auction mechanism for electrical vehicles demand response in microgrids,” in 2017 IEEE 36th international performance computing and communications conference (IPCCC) (San Diego, CA, USA, 1–8).
Lopes, J. A. P., Soares, F. J., and Almeida, P. M. R. (2011). Integration of electric vehicles in the electric power system. Proc. IEEE 99 (1), 168–183. doi:10.1109/jproc.2010.2066250
Makhmalbaf, A., Hammerstrom, D. J., Huang, Q., and Tang, Y. (2017). “Valuation diagramming and accounting of transactive energy systems,” in 2017 IEEE conference on technologies for sustainability (Phoenix, AZ: SusTech), 1–7.
Masiello, R., and Aguero, J. R. (2016). Sharing the ride of power: Understanding transactive energy in the ecosystem of energy economics. IEEE Power Energy Mag. 14 (3), 70–78. doi:10.1109/mpe.2016.2524965
Melton, R. B., and Hammerstrom, D. J. (2012). “Transactive control: A technique for widespread coordination of responsive smart grid assetsin 2012 IEEE PES innovative smart grid technologies (ISGT), (Washington, DC), 1.
Melton, R. B. (2015). “Gridwise transactive energy framework version 1,” Grid-Wise Archit. Council. Richland, WA, USA: Tech. Rep. PNNL-22946.
Mengelkamp, E., Staudt, P., Garttner, J., and Weinhardt, C. (2017). “Trading on local energy markets: A comparison of market designs and bidding strategies,” in 2017 14th international conference on the European energy market (EEM) (Dresden, 1–6).
Moazeni, S., and Defourny, B. (2017). “Distribution system controls assessment in a nonbinding transactive energy market,” in 2017 north American power symposium, Morgantown, WV, 1–6.
Mohsenian-Rad, A. H., and Leon-Garcia, A. (2010). Optimal residential load control with price prediction in real-time electricity pricing environments. IEEE Trans. Smart Grid 1 (2), 120–133. doi:10.1109/tsg.2010.2055903
Mohsenian-Rad, A. H., Wong, V. W. S., Jatskevich, J., Schober, R., and Leon-Garcia, A. (2010). Autonomous demand-side management based on game-theoretic energy consumption scheduling for the future smart grid. IEEE Trans. Smart Grid 1 (3), 320–331. doi:10.1109/tsg.2010.2089069
Moslehi, K., and Kumar, R. (2010). A reliability perspective of the smart grid. IEEE Trans. Smart Grid 1 (1), 57–64. doi:10.1109/tsg.2010.2046346
Nair, V. V., and Nair, U. (2016). “Distributed energy integration and transactive energy framework for a developing economy,” in 2016 IEEE International Symposium on Technology and Society (ISTAS), Trivandrum, India, October 20–22, 2016 (IEEE).
Nunna, H. S. V. S. K., and Srinivasan, D. (2017). Multiagent-based transactive energy framework for distribution systems with smart microgrids. IEEE Trans. Ind. Inf. 13 (5), 2241–2250, Oct. doi:10.1109/tii.2017.2679808
O'Connell, N., Pierre, P., Madsen, H., and O׳Malley, M. (2014). Benefits and challenges of electrical demand response: A critical review. Renew. Sustain. Energy Rev. 39, 686–699. doi:10.1016/j.rser.2014.07.098
Oikonomou, K., Parvania, M., and Khatami, R. (2018). Optimal demand response scheduling for water distribution systems. IEEE Trans. Ind. Inf. 14 (11), 5112–5122, Nov. doi:10.1109/tii.2018.2801334
Olken, M. (2016). Transactive energy: Providing an enabling environment [from the editor]. IEEE Power Energy Mag. 14 (3), 4. doi:10.1109/mpe.2016.2525878
Palensky, P., and Dietrich, D. (2011). Demand side management: Demand response, intelligent energy systems, and smart loads. IEEE Trans. Ind. Inf. 7 (3), 381–388. doi:10.1109/tii.2011.2158841
Parandehgheibi, M., Pourmousavi, S. A., Nakayama, K., and Sharma, R. K. (2017). A two-layer incentive-based controller for aggregating BTM storage devices based on transactive energy framework. Chicago, IL, USA: IEEE Power & Energy Society General Meeting, 1–5.
Patterson, B. T., and Geary, D. E. (2016). “Real-Time transactional power management in a microgrid mesh network: The enernet,” in 2016 IEEE international telecommunications energy conference (INTELEC) (Austin, TX, 1–7).
Pratt, A., Krishnamurthy, D., Ruth, M., Wu, H., Lunacek, M., and Vaynshenk, P. (2016). Transactive home energy management systems: The impact of their proliferation on the electric grid. IEEE Electrific. Mag. 4 (4), 8–14. doi:10.1109/mele.2016.2614188
Pratt, R. (2012). Transactive control with real-time prices and a double-auction feeder market. In 2012 IEEE PES innovative smart grid technologies (ISGT) (Washington, DC, 1).
Prinsloo, Gerro, Mammoli, Andrea, and Dobson, Robert (2017). Customer domain supply and load coordination: A case for smart villages and transactive control in rural off-grid microgrids. Energy 135, 430–441. doi:10.1016/j.energy.2017.06.106
Prinsloo, G., Mammoli, A., and Dobson, R. (2016). “Participatory smartgrid control and transactive energy management in community shared solar cogeneration systems for isolated rural villages,” in 2016 IEEE global humanitarian technology conference (GHTC) (Seattle, WA, 352–359).
Qin, J., Wan, Y., Yu, X., Li, F., and Li, C. (2019). Consensus-based distributed coordination between economic dispatch and demand response. IEEE Trans. Smart Grid 10 (4), 3709–3719. doi:10.1109/tsg.2018.2834368
Qiu, J., Meng, K., Zheng, Y., and Dong, Z. Y. (2017). Optimal scheduling of distributed energy resources as a virtual power plant in a transactive energy framework. IET Gener. Transm. &. Distrib. 11 (13), 3417–3427. doi:10.1049/iet-gtd.2017.0268,
Qiu, J., Zhao, J., Yang, H., and Dong, Z. Y. (2018). Optimal scheduling for prosumers in coupled transactive power and gas systems. IEEE Trans. Power Syst. 33 (2), 1970–1980. doi:10.1109/tpwrs.2017.2715983
Rahimi, F., and Albuyeh, F. (2016). “Applying lessons learned from transmission open access to distribution and grid-edge Transactive Energy systems,” in 2016 IEEE Power & Energy Society Innovative Smart Grid Technologies Conference (ISGT), (Minneapolis, MN), 1–5.
Rahimi, F., Ipakchi, A., and Fletcher, F. (2016). The changing electrical landscape: End-to-End power system operation under the transactive energy paradigm. IEEE Power Energy Mag. 14 (3), 52–62. doi:10.1109/mpe.2016.2524966
Rahimi, F., and Ipakchi, A. (2016). Using a transactive energy framework: Providing grid services from smart buildings. IEEE Electrific. Mag. 4 (4), 23–29. doi:10.1109/mele.2016.2614181
Ramdaspalli, S., Pipattanasomporn, M., Kuzlu, M., and Rahman, S. (2016). “Transactive control for efficient operation of commercial buildings,” in 2016 IEEE PES innovative smart grid technologies conference Europe (ISGT-Europe), Ljubljana, 1–5.
Rayati, M., and Ranjbar, A. M. (2018). Resilient transactive control for systems with high wind penetration based on Cloud computing. IEEE Trans. Ind. Inf. 14 (3), 1286–1296. doi:10.1109/tii.2017.2759223
Sahin, T., and Shereck, D. (2014). “Renewable energy sources in a transactive energy market,” in The 2014 2nd international conference on systems and informatics (ICSAI 2014), Shanghai, 202–208.
Sajjadi, S. M., Mandal, P., Tseng, T. L. B., and Velez-Reyes, M. (2016). “Transactive energy market in distribution systems: A case study of energy trading between transactive nodes,” in 2016 north American power symposium (NAPS) (Denver, CO, 1–6).
Siano, P. (2014). Demand response and smart grids - a survey. Renew. Sustain. Energy Rev. 30, 461–478. doi:10.1016/j.rser.2013.10.022
Siano, Pierluigi, and Sarno, Debora (2016). Assessing the benefits of residential demand response in a real time distribution energy market. Appl. Energy 161, 533–551. doi:10.1016/j.apenergy.2015.10.017
Sun, Q., Han, R., Zhang, H., Zhou, J., and Guerrero, J. M. (2015). A multiagent-based consensus algorithm for distributed coordinated control of distributed generators in the energy Internet. IEEE Trans. Smart Grid 6 (6), 3006–3019, Nov. doi:10.1109/tsg.2015.2412779
TeMIX, E. G. C. (2010). A foundation for transactive energy in a smart grid world. Grid-Interop Forum.
Transactive Energy Infographics (2022). The grid-wise architecture Council. Washington, DC, USA: US Department of Energy. Available at: http://www.gridwiseac.org.
Transactive Energy Principles (V1.0) (2022). The grid-wise architecture Council. Washington, DC, USA: US Department of Energy. Available at: http://www.gridwiseac.org.
Wang, Y., Yemula, P., and Bose, A. (2015). Decentralized communication and control systems for power system operation. IEEE Trans. Smart Grid 6 (2), 885–893. doi:10.1109/tsg.2014.2363192
Widergren, S., Fuller, J., Marinovici, C., and Somani, A. (2014). Residential transactive control demonstration. Washington, DC: ISGT 2014, 1–5.
Xavier, E. B., Goncalves, D. A. V., Dias, B. H., and Borba, B. S. M. C. (2017). Electricity auction simulation platform for learning competitive energy markets. IEEE Potentials 36 (5), 32–36. doi:10.1109/mpot.2016.2563179
Xie, D. J., Hui, H. X., Ding, Y., and Lin, Z. Z. (2018). Operating reserve capacity evaluation of aggregated heterogeneous TCLs with price signals. Appl. Energy 216, 338–347. doi:10.1016/j.apenergy.2018.02.010
Yan, M., Shahidehpour, M., Paaso, A., Zhang, L., Alabdulwahab, A., and Abusorrah, A. (2021). Distribution network-constrained optimization of peer-to-peer transactive energy trading among multi-microgrids. IEEE Trans. Smart Grid 12 (2), 1033–1047. doi:10.1109/tsg.2020.3032889
Yang, C. (2018). Uncertainty-aware transactive operation decisions for grid-friendly building clusters. Chicago, IL: University of Illinois at Chicago.
Yao, Y., and Zhang, P. (2017). “Transactive control of air conditioning loads for mitigating microgrid tie-line power fluctuations,” in 2017 IEEE power & energy society general meeting (Chicago, IL, USA, 1–5).
Yu, P., Wan, C., Song, Y., and Jiang, Y. (2021). Distributed control of multi-energy storage systems for voltage regulation in distribution networks: A back-and-forth communication framework. IEEE Trans. Smart Grid 12 (3), 1964–1977. doi:10.1109/tsg.2020.3026930
Yue, J., Hu, Z., Li, C., Vasquez, J. C., and Guerrero, J. M. (2017). Economic power schedule and transactive energy through an intelligent centralized energy management system for a DC residential distribution system. Energies 10, 916–945. doi:10.3390/en10070916
Zhang, Chenghua, Wu, Jianzhong, Zhou, Yue, Cheng, Meng, and Long, Chao (2018). Peer-to-Peer energy trading in a Microgrid. Appl. Energy 220, pp1–12. doi:10.1016/j.apenergy.2018.03.010
Keywords: transactive energy, distributed energy resources, transactive energy market, transaction mechanism, transactive control
Citation: Zhou H, Li B, Zong X and Chen D (2023) Transactive energy system: Concept, configuration, and mechanism. Front. Energy Res. 10:1057106. doi: 10.3389/fenrg.2022.1057106
Received: 29 September 2022; Accepted: 20 October 2022;
Published: 12 January 2023.
Edited by:
Xue Lyu, University of Wisconsin-Madison, United StatesReviewed by:
Jiayong Li, Hunan University, ChinaYing Wang, Southeast University, China
Hongjun Gao, Sichuan University, China
Copyright © 2023 Zhou, Li, Zong and Chen. This is an open-access article distributed under the terms of the Creative Commons Attribution License (CC BY). The use, distribution or reproduction in other forums is permitted, provided the original author(s) and the copyright owner(s) are credited and that the original publication in this journal is cited, in accordance with accepted academic practice. No use, distribution or reproduction is permitted which does not comply with these terms.
*Correspondence: Biao Li, Ymlhb2xpQHpqdS5lZHUuY24=