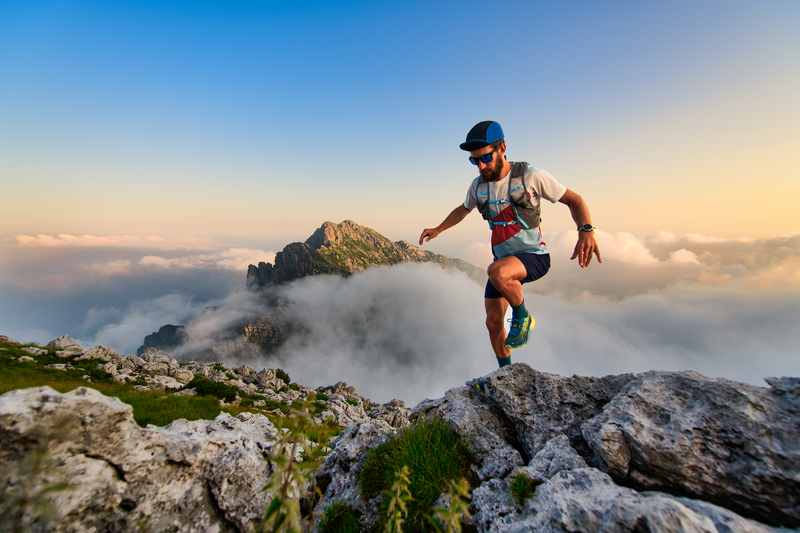
95% of researchers rate our articles as excellent or good
Learn more about the work of our research integrity team to safeguard the quality of each article we publish.
Find out more
ORIGINAL RESEARCH article
Front. Energy Res. , 10 October 2022
Sec. Solar Energy
Volume 10 - 2022 | https://doi.org/10.3389/fenrg.2022.1017293
This article is part of the Research Topic Energy Materials Based Novel Solar Thermal Applications View all 13 articles
The purpose of this study is to investigate the potential of airborne particulate matter (PM10 and PM2.5) and its impact on the performance of the photovoltaic (PV) system installed in the Sargodha region, being affected by the crushing activities in the hills. More than 100 stone crushers are operating in this region. Four stations within this region are selected for taking samples during the summer and winter seasons. Glass–fiber papers are used as a collection medium for particulate matter (PM) in a high-volume sampler. The concentration of PM is found above the permissible limit at all selected sites. The chemical composition, concentration, and the formation of particulate matter (PM10 and PM2.5) layers on the surface of the photovoltaic module varies significantly depending on the site’s location and time. The accumulation of PM layers on the PV module surface is one of the operating environmental factors that cause significant reduction in PV system performance. Consequently, it leads to power loss, reduction of service life, and increase in module temperature. For the PV system’s performance analysis, two PV systems are installed at the site, having higher PM concentration. One system is cleaned regularly, while the other remains dusty. The data of both PV systems are measured and compared for 4 months (2 months for the summer season and 2 months for the winter season). It is found that when the level of suspended particulate matter (PM10 and PM2.5) increases, the energy generation of the dusty PV system (compared to the cleaned one) is reduced by 7.48% in May, 7.342% in June, 10.68% in December, and 8.03% in January. Based on the obtained results, it is recommended that the negative impact of PM on the performance of the PV system should be considered carefully during the decision-making process of setting solar energy generation targets in the regions with a high level of particulate matter.
Solar energy is the most important and primary renewable energy source due to its free availability and environmental friendliness (Thapar, 2019). In the next few years, the benefits and advantages of solar energy, either directly or indirectly, will be observed by everyone globally (Bono, 2018). Solar energy technologies and applications that have been widely used include photovoltaic systems (Chaichan and Kazem, 2018), concentrated solar power stations (Xingping et al., 2019), solar water heating system (Maraj et al., 2019), solar water desalination (Chaichan and Kazem, 2015), Trombe walls (Martín-Consuegra et al., 2021), solar air heating, and space conditioning (Ojike and Okonkwo, 2019). Due to the declining cost and higher electrical efficiency of photovoltaic modules, there has been growing interest maximize the production of solar energy (Schleifer et al., 2022; Tamoor et al., 2022). Hachich et al. (2019) showed that the electrical efficiency of commercial and modern solar cells increases to 20%. Additionally, the photovoltaic modules can also be installed in a number of locations, including the building’s envelope (Middelhauve et al., 2021), plain areas, mountains, oceans, and deserts. They can also operate as standalone or hybrid with other systems (such as wind, diesel generators, and batteries) (Kazem et al., 2017; Jain and Sawle, 2021; Chen and Zhu, 2022; Nkuriyingoma et al., 2022). Among the various practical applications of renewable energy sources, the photovoltaic (PV) system has attracted attention and is widely used in the field of energy generation due to its low operation, maintenance costs, and relatively high energy conversion efficiency (Gil et al., 2020; Soomar et al., 2022). In many countries, photovoltaic conversion of solar energy has begun to make a significant contribution to energy generation, and more than 91% of the global photovoltaic market relies on crystalline silicon-based solar cells. Crystalline silicon solar cells have a current efficiency record of 26.7%, against an intrinsic limit of 29% (Andreani et al., 2019). Many developing and developed countries are planning to shift to renewable energy resources to meet the energy demands of growing populations and improve the operation of national utility grids (Tamoor et al., 2020; Tamoor et al., 2021; Miran et al., 2022; Tamoor et al., 2022; Tamoor et al., 2022; Tamoor et al., 2022). However, the performance of all types of photovoltaic modules is affected by a large number of environmental factors (air temperature, wind speed, the angle of incident irradiation, solar radiation intensity, solar radiation spectrum, air pollution, snow, aging, dirt, and shadows) (Hassan et al., 2016; Kazem et al., 2017; Chaichan and Kazem, 2018). Fouad et al. (2017) proposed that the performance of photovoltaic modules can be affected by more than 30 factors, which are mainly related to the environment, including solar irradiation, dust accumulation, PV module temperature, and shading. One of the most important factors that affect the energy conversion of photovoltaic modules in many regions is dust accumulation (Chaichan et al., 2015). Because dust particles lower and disperse the intensity of the solar irradiation impacting on the photovoltaic modules, as a result, the conversion efficiency is reduced by accumulated dust. It was observed that the rate of dust accumulation on photovoltaic modules depends on a number of factors, like local weather conditions, airborne particle concentration, type and size of particle distribution, composition, shape, and density (Javed e t al., 2017; Cui et al., 2021). Dust accumulation on the surface of any photovoltaic module will depend on the dust type, weather, surrounding environment, PV module characteristics, and installation design (Konyu et al., 2020). The efficiency and output energy of a PV module would decline as the rate of dust deposition on the module surface increases. In desert regions, dust accumulation reduces the total output energy of photovoltaic modules by an average of almost 40% within a year (Ahmed and Massier, 2019). The solar energy incident on the surface of Earth in 1 hour is nearly equal to the total consumption of Earth in 1 year (Tomin et al., 2022). When solar irradiation passes through the atmosphere, it is absorbed by solid particles and droplets in the atmosphere and reflected by water vapor and air molecules, resulting in significant loss of energy. However, solar radiation is absorbed by dust and other pollutants and scattered backward, resulting in a decrease in direct solar radiation and an increase in diffuse solar radiation. Hence, compared to clean air in non-industrial rural areas, cities and polluted areas generally receive less total solar irradiation (Darwish et al., 2018). Another important aspect that greatly affects the rate of dust deposition on the PV module is how close it is to an area where dust is most likely to be airborne (Cheema et al., 2021). The particle size distribution of dust deposited on the surface of photovoltaic modules and its chemical and physical properties have a major impact on the degradation in the performance of the photovoltaic module (Kaldellis and Kapsali, 2011). The size of deposited solid particles has a significant impact on the absorption and scattering of incident radiation on the photovoltaic module, resulting in a decrease in the efficiency of photovoltaic modules. Larger particles are more likely to resuspend with the airflow, promoting the deposition of smaller particles. Compared with large particles with the same quality and volume of the deposited dust, fine/small particles have a larger specific surface area and have a greater impact in degradation of the photovoltaic cell performance (Weber et al., 2014). The size of dust particles gathered on the surface is divided into three different ranges: small-sized particles (up to 5.0 μm in diameter) that come from large-spaced areas, medium particles (20.0 μm–40.0 μm in diameter) that contain dust deposits from regional sources, and large-sized particles (50.0 μm–70 μm) that come from automobiles, humans, and livestock. Gravity or other forces related to heat transfer and fluid flow causes solid particles or droplets to accumulate on a PV module surface (Jiang and Lu, 2015). The performance of photovoltaic modules is reduced by solid particles. This causes power losses, which reduces the system efficiency and increases temperature, which further reduces system performance and service life. The chemical composition of deposited dust, its concentration, and the creation of a dust layer on the photovoltaic surface vary greatly depending on location and time (Styszko et al., 2019). In addition, after the East Asian monsoon, PM concentrations vary seasonally due to major fluctuations in rainfall and regional circulation (Lou et al., 2019). The impact of dust deposition on the performance of PV modules is observable, but the dust composition might be different depending on the location, so the degree of reduction in the photovoltaic module efficiency may vary from location to location (Andrea et al., 2019). However, the solar panel manufacturer typically guarantees 80% of the nominal module power for up to 25 years, and the output power is highly dependent on local environmental conditions and ambient intensity. Dust deposition has no effect on the open circuit voltage of a photovoltaic module, but it has a significant impact on the short circuit current, resulting in a drop in output current and, as a result, power generation is decreased. The dust deposition represents a massive loss in energy production and an economic loss for a photovoltaic power plant (Mustafa et al., 2020). Several research studies have examined the detrimental effects of dust accumulation on the surface of photovoltaic modules. Salimi et al. (2019) investigated the impact of relative humidity and wind speed on a performance ratio called the cleanness index (CI) that compares the performance of clean photovoltaic modules to that of dirty photovoltaic modules as a result of dust precipitation. According to the study, the cleanness index was positive when measuring the effects of wind speed, and the cleanness index was negative when measuring the effects of dust concentration and relative humidity. Dust deposits on photovoltaic modules cause their performance to decrease by 8%–12% per month. Kazem and Chaichan (2016) investigated that the physical properties and characteristics of dust are crucial in stimulating the final outcome of photovoltaic modules, while Alnasser et al. (2020) highlighted that dust composition and its physical and chemical properties are the factors that determine its detrimental effect on photovoltaic module performance. Jiang, Lu, and Sun (2011) pointed out that fine dust particles caused a more significant decrease in photovoltaic performance than coarse dust particles. Small dust particles are blown far away by the wind, and their accumulation on the surface of photovoltaic modules prevents the passage of radiation and increases the scattering of light because it adheres more clearly to the surface of photovoltaic modules than larger particles (Tanesab et al., 2015). Lu and Zhao (2019) investigated that ground-mounted photovoltaic systems had higher dust accumulation rates than roof-mounted systems, especially when the station was close to traffic. Furthermore, photovoltaic modules installed at a fixed tilt angle have more dust accumulations on the surface than photovoltaic modules installed with Sun-tracking systems (whether single or dual axis). Table 1 presents a comparative analysis of this proposed research study and previous research studies on the effects of dust on photovoltaic systems. Manju et al (2018) investigated various methods of dust removal from photovoltaic modules, including dry cleaning with clothes, cleaning with water, and the use of compressed air, mechanical brushes, vibration, electrostatic electricity, and ultrasound. Zorrilla-Casanova et al. (2011) showed that utilizing water to clean photovoltaic modules is more efficient than using dry cleaning, but using this technique in water-scarce deserts is an expensive process. Chaichan et al. (2019) explained that the techniques that must be followed for cleaning photovoltaic modules depend on the primary dust constituents and their chemical properties. The location of the photovoltaic modules and the type of dust accumulation due to the topography of the area directly affect the selection of appropriate cleaning techniques. In the agricultural area, dust particles consist of organic pollen, leaves, bird droppings, and soil. In the coastal area, salt can accumulate on photovoltaic modules. In the urban area, dust particles are primarily composed of carbon that comes from automotive exhaust. Dust particles in desert regions are primarily made of minerals (sand, quartz, silica, etc.) (Middleton, 2017; Chen et al., 2020; Fan et al., 2021). The stone crushing sector supplying construction materials is crucial for both employment and the economy in developing countries. These sectors are generally labor-intensive and small-scale. Stone crushing has a significant impact on the socio-economic environment of the local population. The different processes related to the breaking, size reduction, and transferring of stones can release dust (Ganguly and Das, 2020). Stone crushers also produce dust as a by-product due to mechanical reduction and screening operations (Von Schneidemesser et al., 2020). Due to the crushing activity in the crushing zone, both the natural environment of the hills and air quality of the surrounding area are endangered. This pollutant air (airborne pollutants) also affects photovoltaic energy system performance. Taking into consideration the dust produced as a by-product in the stone crushing sector, the efficiency and output energy of a photovoltaic energy generation installed in the area near to the crushing zone would decline as the rate of dust accumulation on the PV module surface increases. In order to optimize the output energy of the PV system in stone crushing zones, a comprehensive analysis of the impact of dust accumulation is crucial. To the best of the author’s knowledge, no such research study has been conducted; hence, a comprehensive methodology is definitely needed, especially in severe environments like stone crushing zones. A research study is presented to analyze the effects of dust accumulation on the PV energy generation system in crushing zones. With the guidance of this study, commercial and residential PV system installers could more precisely plan their systems in stone crushing zones, which should ultimately result in higher output energy from PV systems. The aim of the study is to evaluate and assess air pollution (particulate matter) produced by stone crushers in the crushing zone and the impact of airborne pollutants on the performance of photovoltaic energy systems.
TABLE 1. Comparative analysis of the proposed research study and previous research studies on the effects of dust on photovoltaic systems.
An outdoor station, consisting of a wind vane, beaker, and thermo hygrometer, was installed outside on the roof of the tall building near the crushing zone. Wind speed and direction were continuously monitored every hour to determine the wind speed and wind direction for the air quality monitoring. It has been observed and recorded that the wind was blowing from the west to the east in the Sargodha region. During the experiment, the wind direction is very important to achieve a suitable result. A higher precipitation pattern was also observed. Four sampling sites were selected for the study of PM concentration in this region. Stone crushing activities were carried out at these four sampling sites located on both sides of the Grand Trunk (GT) road as shown in Figure 1.
Experiments were conducted in two seasons: winter and summer. December and January were selected for the winter season and May and June for the summer season because the weather conditions of the crushing area change throughout the year. A three-day experiment was performed at four sampling sites, and then the average of these values was calculated to estimate the exact PM concentration. In this way, experimental values for 12 days were calculated for each season. Sampling of ambient air was performed for 24 h. For experiments, a large volume air sampler was used for air sampling. The recovery medium used in these experiments was Whatman glass microfiber filter paper. The dust concentration was calculated from the weight difference of the filter paper before and after sampling. After compiling the experimental results, all parameters were analyzed according to the National Ambient Air Quality Standard (NAAQS) methods prescribed by the Environmental Protection Agency (EPA), Pakistan.
First, PM concentration was analyzed on all four selected sites. After PM concentration analyses, the HelioScope simulation model of the photovoltaic power generation was developed by taking different parameters under consideration, like the architectural design of the building, photovoltaic power system specifications, and the shading conditions. HelioScope is one of the finest simulation software for modeling and analyzing the PV system (Goel and Sharma, 2021). To enable energy system designers to use a single software program to create a whole PV system design, HelioScope simulation software combines all the features of PVSyst with AutoCAD design features. HelioScope makes it possible to create 3D model designs and estimate energy production (kWh) using a same simulation tool. From small to large size, and for every kind of surface, including flat or uneven surfaces, it is capable of designing photovoltaic systems. It has the benefit of being able to provide a very accurate estimate for the PV system size that would be installed at the proposed area, monthly and annual energy production (kWh), system performance ratio (PR), expected energy production (kWh/kWp), photovoltaic system losses, photovoltaic plant layout, number of PV modules and inverters, single line diagram (SLD), shading analysis, electrical wiring selection, total collector irradiation, annual global horizontal irradiation, SketchUp, and CAD integration (Tamoor et al., 2022). The comparison of HelioScope with other PV system simulation software is shown in Table 2.
TABLE 2. Comparison of HelioScope with other PV system simulation software (Tamoor et al., 2022).
Later, two 10.20-kW grid systems are designed and installed at the site, which have higher PM concentration to analyze the effect of PM concentration on the performance of the photovoltaic energy generation system. For the photovoltaic system performance analysis, one system is cleaned on a regular basis while the other is not cleaned at all and remains dusty.
In both systems, the Jinko Solar mono crystalline (JKM510M-7TL4-V) type photovoltaic module was used. For the photovoltaic array size, the PV array design factor of 1.35 is used. A 10.20 kW the grid system consisting of 20 W × 510 W Jinko Solar PV modules has a maximum power voltage of 41.400 V and open circuit voltage of 48.940 V. The specification of 510 W mono crystalline Jinko Solar is given in Table 3. Both photovoltaic systems are installed at 18° tilt angle and at an azimuth angle of 180°. A manually movable structure with a 2 up x 5 wide frame size is used for installation and orientation of the PV modules.
A Solis three phase inverter, model number Solis-3P10K-4G, is used in the installation of the test system. Table 4 shows the detailed specifications of the Solis three-phase inverter. They convert the DC power produced by photovoltaic modules into three-phase AC power at the frequency of 50.0 Hz and synchronize the output power with the utility distribution system. The Solis-3P10K-4G inverter only operates when connected to the utility AC grid and cannot be operated as a standalone mode.
In order to more accurately study the impact of dust accumulation on photovoltaic modules, an experimental setup is installed. The schematic diagram of the experimental process is shown in Figure 2. The experimental design process and data measurement are divided into two parts, Part-1: air quality monitoring and assessment and Part-2: PV system analysis. Air quality monitoring and assessment includes selection of sampling sites in the stone crushing zone, and air quality monitoring at all selected sites uses different apparatuses like wind vane, thermo hygrometer, high-volume air sampler, glass–fiber filter papers, and analytical balance in the summer and winter seasons. The dust concentration was calculated from the weight difference of the filter paper before and after sampling. PV system analysis includes the selection of PV system components like PV modules, inverts, and electrical wiring. After selection, the PV system is designed. HelioScope simulation software is used to perform the simulation study of the designed PV system. Finally, the effect of dust on the performance of the photovoltaic system is found.
The calculated PM concentrations on all four stations as compared to National Ambient Air Quality Standard in the winter season are shown in Figure 3. The calculated concentration at site station 1 is 520.236 μg/m3, at site station 2 is 525.76 μg/m3, at site station 3 is 316.087 μg/m3, and at site station 4 is 182.34 μg/m3. From the experimental study, it is found that the concentration of PM at site station 1, site station 2, site station 3, and site station 4, which is the residential area, is above the NAAQS values. A very high difference is observed between the experimental value and NAAQS, which leads to an adverse impact on photovoltaic power generation.
The calculated PM concentrations on all four stations as compared to national ambient air quality standard in the summer season are shown in Figure 4. The calculated concentration at site station 1 is 416.049 μg/m3, at site station 2 is 439.177 μg/m3, at site station 3 is 228.98 μg/m3, and at site station 4 is 155.45 μg/m3. From the experimental study, it is found that the concentration of PM at site station 1, site station 2, site station 3 and site station 4, which is residential area, is above the NAAQS values. A very high difference is observed between the experimental value and NAAQS, which leads to an adverse impact on photovoltaic power generation.
The simulation model of the photovoltaic power generation is developed by taking different parameters under consideration like the architectural design of the building, photovoltaic power system specifications, and the shading conditions. The physical characteristics of the photovoltaic power system (like the size of the system, azimuth and tilt angle of the system, orientation, height, and type of PV modules and inverters) are modeled in HelioScope simulation software. Photovoltaic modules and building blocks are simplified using a parameterized model that takes into account the real size and shape of the components. In the parameter setting column, detailed photovoltaic module parameters (such as PV cell pattern, tilt and azimuth angle, and distance between PV modules and rows) are imported. The weather data are based on the current weather conditions at the chosen location. Shading modeling is simulated automatically.
In the simulation, the overall energy performance is evaluated on a monthly basis by using a photovoltaic monitoring system that receives real-time data directly from the meter. The complete monthly power generation data from January 2020 to December 2020 have been extracted. The simulation results of a designed photovoltaic system showed that the annual energy generation is 15.79 MWh, the performance ratio (PR) is 88.1%, and the power generation (kWh/kWp) is 1548.2. Figure 5 shows the photovoltaic system’s monthly power production.
Figure 5 shows that the maximum produced power is 1,708.2 kWh in May, and minimum produced power is 841.1 kWh in December. For solar energy applications, accurate and precise measurements of global horizontal irradiance (GHI) are crucial. It is necessary to use GHI to forecast the output of solar thermal collectors or photovoltaic system. Thus, understanding GHI is crucial for activities like resource assessment during photovoltaic installation planning and optimizing photovoltaic plant operation (Nouri et al., 2020; Toledo et al., 2020). The global horizontal irradiance of the site is 204.4 kWh/m2 in May and 79.7 kWh/m2 in December. The annual global horizontal irradiance is shown in Figure 6.
Determining the irradiance incident on the plane of the array (POA) as a function of time is a crucial step in calculating photovoltaic system performance. Several factors influence the plane of the array irradiance like orientation of the PV modules, shading, Sun position, latitude, longitude, and weather conditions (Nour et al., 2019; Meyers, 2021; Blum et al., 2022). The plane of array irradiance of the site is 206.3 kWh/m2 in May and 99.7 kWh/m2 in the February. The plane of the array irradiance is shown in Figure 7.
Figure 8 represents major photovoltaic system losses, like temperature losses, irradiance losses, mismatch losses, soiling losses, electrical wiring losses, reflection losses, clipping losses, shading losses, inverters losses, and AC system losses. The temperature losses are 6.2%, irradiance losses are 0.4%, mismatch losses are 4.0%, soiling losses are 2.0%, electrical wiring losses are 0.3%, reflection losses are 3.2%, clipping losses are 0.0%, shading losses are 0.4%, inverter losses are 3.0% and AC system losses are 0.5%.
The concept “soiling losses” refers to a reduction in power or yield as a result of accumulation of dust, sand, snowfall, and other falling particles on the photosensitive surface of the photovoltaic module. By concentrating on dust soiling, a thin layer of paper-sized dust particles with a diameter of less than 10 microns covers the surface of the PV module. The continuously accumulating dust increasing the soiling impact will result in reducing the energy of the photovoltaic system from daily to annual rates. Generally, dust characteristics (e.g., chemical composition and size of particle), local environment condition (e.g., humidity, temperature, wind speed, and direction), and photovoltaic module installation (e.g., irradiation level, tilt angle, and azimuth angle) influence the accumulation impact of dust (particulate matter) on the performance of the photovoltaic system. The impact of soiling losses is shown in Table 5.
The accumulation of a particulate matter layer on the photovoltaic module surface is one of the operating environmental factors that cause significant reduction in PV system performance, which further leads to power loss, reduces service life, reduces irradiance, and increases module temperature.
The normal operating temperature for photovoltaic modules is 25°C. The performance of photovoltaic modules is most significantly impacted by temperature. However, because the selected site for the PV system is in a hot climate region, the ambient temperature (Ta) of the photovoltaic modules has increased excessively. The PV module temperature is calculated as follows (Kim et al., 2021):
Here,
•
•
•
•
•
•
By using Equation no. 1 for PV module temperature, 6.2% losses are calculated. It is found that the efficiency of the photovoltaic module decreases as the temperature of the photovoltaic module increases. In a simulation study, power is analyzed at different irradiance levels with temperature variation as shown in Table 6.
From Table 6, it is observed that at normal operating temperature 25°C and irradiance level 1000 W/m2, the output power of the PV module is 510.3 W, and at 50°C operating temperature and irradiance level 100 W/m2, the power output of the PV module is 43.6 W. From the simulation result, it is found that dust accumulation has a significant impact on the performance of the PV system because it increases module temperature and reduces the amount of irradiance that reaches the surface of the modules.
To examine the power production and efficiency of both cleaned 10.20-kW PV systems installed near the stone crushing zone, readings of power production from both PV systems are taken for 10 days in April. The power production from both photovoltaic systems is shown in Figure 9.
Figure 9 shows that the maximum power produced by the installed photovoltaic system 1 and system 2 in 1 day is 7,463 and 7491 kWh, respectively. The power produced by both systems is almost the same because PV systems having same specification are installed near the crushing zone. The photovoltaic systems produced lower power due to bad weather condition, i.e., it rained at day 9.
For the photovoltaic system performance analysis, one system is cleaned on a regular basis, while the other is not cleaned at all and remains dusty. The experimental setup of the installed clean and dusty photovoltaic energy generation systems is shown in Figure 10.
The average data of both systems are calculated and compared for 4 months (2 months for the summer season and 2 month for the winter season). In the summer season, the comparison between both systems is performed in May and June, and in the winter season, the comparison between both systems is performed in January and December. Table 7 shows the parameters of both photovoltaic systems for May.
Table 7 shows that for May, the average power produced by the cleaned PV system is 7451.85 W and the average power produced by the dusty PV system is 6893.8 W. So, 7.48% power is reduced due to PM concentration on the surface of the photovoltaic modules. The I–V curve of cleaned and dusty PV systems to compare system performance in May is shown in Figure 11.
Table 8 shows that for June, the average power produced by the cleaned PV system is 7430.91 W and the average power produced by the dusty PV system is 6885.3 W. So, 7.342% power is reduced due to PM concentration on the surface of the photovoltaic modules. The I–V curve of cleaned and dusty PV systems to compare system performance in June is shown in Figure 12.
Table 9 shows that for December, the average power produced by the cleaned PV system is 6892.06 W and the average power produced by the dusty PV system is 6155.6 W. So, 10.68% power is reduced due to PM concentration on the surface of the photovoltaic modules. The I–V curve of cleaned and dusty PV systems to compare system performance in December is shown in Figure 13.
Table 10 shows that for January, the average power produced by the cleaned PV system is 6892.06 W and the average power produced by the dusty PV system is 6155.6 W. So, 8.03% power is reduced due to PM concentration on the surface of the photovoltaic modules. The I–V curve of cleaned and dusty PV systems to compare system performance in January is shown in Figure 14.
This study investigates the effects of particulate matter (PM2.5 and PM10) on the photovoltaic energy generation system by analyzing actual energy data from two PV systems installed near the Sargodha stone crushing zone in the winter and summer seasons. The methodologies used in this research are the air quality assessment at four selected sites, designing and simulation study of the PV system, analyzing actual energy data and system losses of two PV systems installed near the stone crushing zone, and other meteorological parameters (like GHI and POA) that are different from those in previous assessments, which typically focus on PM influences on solar irradiance and simulated photovoltaic energy output (Peters et al., 2018; Son et al., 2020).
Air quality experiments were conducted in two seasons: winter and summer. December and January were selected for the winter season and May and June for the summer season because the weather conditions of the crushing area change throughout the year. A three-day experiment was performed at four sampling sites, and then the average of these values was calculated to estimate the exact PM concentration. In this way, experimental values for 12 days were calculated for each season. After compiling the experimental results, all parameters were analyzed according to the National Ambient Air Quality Standard (NAAQS) standard methods prescribed by the Environmental Protection Agency (EPA), Pakistan. In the winter season, the calculated PM concentration at site station 1 is 520.236 μg/m3, at site station 2 is 525.76 μg/m3, at site station 3 is 316.087 μg/m3, and at site station 4 is 182.34 μg/m3. In the summer season, the calculated PM concentration at site station 1 is 416.049 μg/m3, at site station 2 is 439.177 μg/m3, at site station 3 is 228.98 μg/m3, and at site station 4 is 155.45 μg/m3. From the experimental study, it is found that the concentration of PM at site station 1, site station 2, site station 3, and site station 4, which is residential area, is above the NAAQS values. A very high difference is observed between the experimental value and NAAQS, which leads to an adverse impact on photovoltaic power generation.
The simulation model of the photovoltaic power generation is developed by taking different parameters under consideration. The simulation results of a designed photovoltaic system showed that the annual energy generation is 15.79 MWh and the performance ratio (PR) is 88.1%, the performance ratio in this research is high as compared to the research study (Navothna and Thotakura, 2022), and the power generation (kWh/kWp) is 1548.2. The maximum monthly energy production is 1,708.2 kWh, the maximum global horizontal irradiance (GHI) is 204.4 kWh/m2, and the maximum plane of array irradiance (POA) is 206.3 kWh/m2 in May. Soiling losses due to the accumulation of dust influence the performance of the PV system. The simulation results show that when the soiling losses are 2%, the annual energy generation of the photovoltaic system is 15.79 MWh, and the performance ratio is 88.1%. The annual energy generation and performance ratio of the PV system declines as the percentage of soiling losses increases. The annual energy generation and performance ratio of the PV system declines as the percentage of soiling losses increases. The performance of photovoltaic modules is most significantly impacted by temperature. At normal operating temperature 25°C and 1000 W/m2 irradiance level, the output power of the PV module is 510.3 W, and at 50°C operating temperature and 100 W/m2 irradiance level, the power output of the PV module is 43.6 W.
For 4 months, Figure 15 displays the impact of PM10 and PM2.5 concentrations on the rate of reduction of photovoltaic energy generation. The findings reveal that the amount of photovoltaic energy generation decreases with the deterioration of air quality. The reduction rate of photovoltaic energy generation due to PM10 and PM2.5 is higher in the dusty photovoltaic energy generation system than in the cleaned photovoltaic energy generation system. In May, the average power produced by the cleaned PV system is 7451.85 W and the average power produced by the dusty PV system is 6893.8 W. In June, the average power produced by the cleaned PV system is 7430.91 W and the average power produced by the dusty PV system is 6885.3 W. In December, the average power produced by the cleaned PV system is 6892.06 W and the average power produced by the dusty PV system is 6155.6 W. In January, the average power produced by the cleaned PV system is 6892.06 W and the average power produced by the dusty PV system is 6155.6 W. It was found that when the level of suspended particulate matter (PM10 and PM2.5) increases, the energy generation of the dusty PV system is reduced by 7.48% in May, 7.342% in June, 10.68% in December, and 8.03% in January under bad air quality, 439.177 μg/m3 and 535.76 μg/m3 in the summer and winter seasons, respectively, as compared to the photovoltaic energy generation systems that are regularly cleaned.
Based on the aforementioned results, it can be predicated that a large amount of suspended particulate matter (PM10 and PM2.5) reduces the energy generation of the photovoltaic system. It is important to note that these high levels of particulate matter (PM10 and PM2.5) are damaging to photovoltaic generation because the higher the concentration of PM10 and PM2.5, the greater the adverse impact on photovoltaic power generation. The limitations of this study are that it investigated the impact of suspended particulate matter on the performance of the photovoltaic energy generation system. These suspended particulate matters (PM2.5 and PM10) are produced during the stone crushing process. Based on a comparative analysis of the proposed research study and previous research studies on the effects of dust on photovoltaic systems, Guan et al. (2017) performed a similar study for 8 days only, Hachicha et al. (2019) performed laboratory tests, and Al-Badra et al. (2020) performed a similar study for 6 weeks, proposed a research which provides good result because this research is performed in all seasons of the year, and investigated all environmental impacts produced due to the change in weather.
Many environmental factors influence photovoltaic energy system production, and particulate matter (PM10 and PM2.5) can be one of the main causes of the reduction of photovoltaic energy. Particulate matter (PM10 and PM2.5) is a mixture of different pollutants and varies significantly depending on site location and time. This study aims to investigate the process of particulate matter (PM10 and PM2.5) accumulation on the photovoltaic system installed near the stone crushing zone in the Sargodha region, one of the most polluted crushing zones in Pakistan. The four sampling stations were selected for sampling during the summer and winter seasons. December and January were selected for the winter season and May and June for the summer season because the weather conditions of the crushing area change throughout the year. Glass–fiber filter papers are used as a collection medium for particulate matter in a high-volume sampler. A three-day experiment was performed at four sampling sites, and then the average of these values was calculated to estimate the exact PM concentration. In this way, experimental values for 12 days were calculated for each season. After compiling the experimental results, all parameters were analyzed according to the National Ambient Air Quality Standard (NAAQS) methods prescribed by the Environmental Protection Agency (EPA), Pakistan. In the winter season, the calculated PM concentration at site station 1 is 520.236 μg/m3, at site station 2 is 525.76 μg/m3, at site station 3 is 316.087 μg/m3, and at site station 4 is 182.34 μg/m3. In the summer season, the calculated PM concentration at site station 1 is 416.049 μg/m3, at site station 2 is 439.177 μg/m3, at site station 3 is 228.98 μg/m3, and at site station 4 is 155.45 μg/m3.
For the photovoltaic system performance analysis, two PV systems installed at the site have higher PM concentrations, where one system is cleaned on a regular basis while the other is not cleaned at all and remains dusty. The HelioScope simulation model of the photovoltaic power generation is developed by taking different parameters under consideration like the architectural design of the building, photovoltaic power system specifications, and shading conditions. Simulation results show that the annual energy generation is 15.79 MWh and the performance ratio (PR) is 88.1%; the performance ratio in this research is high as compared to that of the research study (Navothna and Thotakura, 2022). The power generation (kWh/kWp) is 1548.2, the maximum produced power is 1,708.2 kWh in May, and the minimum produced power is 841.1 kWh in December. The global horizontal irradiance (GHI) of the site is 204.4 kWh/m2 in May and 79.7 kWh/m2 in December. The plane of array irradiance (POA) of the site is 206.3 kWh/m2 in May and 99.7 kWh/m2 in February. Major losses of PV systems are also calculated using simulation software. The temperature losses are 6.2%, irradiance losses are 0.4%, mismatch losses are 4.0%, soiling losses are 2.0%, electrical wiring losses are 0.3%, reflection losses are 3.2%, clipping losses are 0.0%, shading losses are 0.4%, inverter losses are 3.0%, and AC system losses are 0.5%. The losses in this research are lower than losses in the research study (Navothna and Thotakura, 2022). The annual energy generation and performance ratio of the PV system decline as the percentage of soiling losses and temperature of the site increases.
The data of both PV systems are measured and compared for 4 months (2 months for the summer season and 2 months for the winter season). The average power produced by the cleaned PV system is 7451.85, 7430.91, 6892.06, and 6892.06 W, and the average power produced by the dusty PV system is 6893.8, 6885.3, 6155.6, and 6155.6 W May, June, December, and January, respectively. It was found that when the level of suspended particulate matter (PM10 and PM2.5) increases, the energy generation of the dusty PV system is reduced by 7.48% in May, 7.342% in June, 10.68% in December, and 8.03% in January under bad air quality, 439.177 μg/m3 and 535.76 μg/m3 in the summer and winter seasons, respectively, as compared to the photovoltaic energy generation system that is regularly cleaned. Based on the obtained results, it is recommended that the negative impact of PM on the performance of the PV system should be considered carefully during the decision-making process of setting solar energy generation targets in the regions with a high level of particulate matter.
The original contributions presented in the study are included in the article/Supplementary Material; further inquiries can be directed to the corresponding author.
Conceptualization, MT, AB, and SM; methodology, MT, MH, AB, and SM; software, MT, SM, WA, TK, MH, and GL; validation, MT, AB, SM, and GL; formal analysis, MT, MH, GL, and TK; investigation, MT, AB, and SM; resources, MT, SM, and MH; writing—original draft preparation, MT, AB, SM, and TK; writing—review and editing, MT, AB, SS, MH, and GL; visualization, MT, AB, SM, TK, and WA; supervision, AB, SS, MH, and GL; project administration, MT, AB, SM, MH, and GL; funding acquisition, MH and GL. All authors have read and agreed to the published version of the manuscript.
This work was supported by the National Research Foundation of Korea (NRF) grant funded by the Korea government (MSIT) (No. 2022R1F1A1062793); Korea Institute of Planning and Evaluation for Technology in Food, Agriculture and Forestry (IPET) through Technology Commercialization Support Program, funded by Ministry of Agriculture, Food and Rural Affairs (MAFRA) (No. 821048-3).
The authors gladly acknowledge the continued support of the Department of Electrical Engineering and Technology, GC University Faisalabad.
The authors declare that the research was conducted in the absence of any commercial or financial relationships that could be construed as a potential conflict of interest.
The reviewer AH and reviewer MF declared a shared affiliation with the author TK at the time of the review.
The handling editor declared a shared affiliation with the author TK, MPS at the time of the review.
All claims expressed in this article are solely those of the authors and do not necessarily represent those of their affiliated organizations, or those of the publisher, the editors, and the reviewers. Any product that may be evaluated in this article, or claim that may be made by its manufacturer, is not guaranteed or endorsed by the publisher.
PV, photovoltaic; W, watt; kW, kilowatt; kWh, kilowatt hour; MWh, megawatt hour; A, ampere; V, volt; SLD, single-line diagram; PR, performance ratio; AC, alternating current; DC, direct current; MPPT, maximum power point tracking; NOCT, nominal operating cell temperature; GHI, global horizontal irradiance; POA, plane of the array; DHI, diffuse horizontal irradiance; DNI, direct normal irradiance; Ta, ambient temperature; Tm, PV module temperature; Ws, wind speed at proposed site; E, solar irradiation on photovoltaic module; PM, particulate matter; NAAQS, National Ambient Air Quality Standard; EPA, Environmental Protection Agency; CI, cleanness index; and ASHRAE, American Society of Heating, Refrigerating, and Air-Conditioning Engineers.
Abderrezek, M., and Fathi, M. (2017). Experimental study of the dust effect on photovoltaic panels’ energy yield. Sol. Energy 142, 308–320. doi:10.1016/j.solener.2016.12.040
Ahmed, A., and Massier, T. (2019). “Impact of climate change and high PV penetration on power factor profile,” in 2019 IEEE industry applications society annual meeting (IEEE), 1–6.
Al-Badra, M. Z., Abd-Elhady, M. S., and Kandil, H. A. (2020). A novel technique for cleaning PV panels using antistatic coating with a mechanical vibrator. Energy Rep. 6, 1633–1637. doi:10.1016/j.egyr.2020.06.020
Alnasser, T. M., Mahdy, A. M., Abass, K. I., Chaichan, M. T., and Kazem, H. A. (2020). Impact of dust ingredient on photovoltaic performance: An experimental study. Sol. Energy 195, 651–659. doi:10.1016/j.solener.2019.12.008
Andrea, Y., Pogrebnaya, T., and Kichonge, B. (2019). Effect of industrial dust deposition on photovoltaic module performance: Experimental measurements in the tropical region. Int. J. Photoenergy, 1–10. doi:10.1155/2019/1892148
Andreani, L. C., Bozzola, A., Kowalczewski, P., Liscidini, M., and Redorici, L. (2019). Silicon solar cells: Toward the efficiency limits. Adv. Phys. X 4 (1), 1548305. doi:10.1080/23746149.2018.1548305
Blum, N. B., Wilbert, S., Nouri, B., Lezaca, J., Huckebrink, D., Kazantzidis, A., et al. (2022). Measurement of diffuse and plane of array irradiance by a combination of a pyranometer and an all-sky imager. Sol. Energy 232, 232–247. doi:10.1016/j.solener.2021.11.064
Chaichan, M. T., Kazem, H. A., Al-Waeli, A. H., and Sopian, K. (2019). The effect of dust components and contaminants on the performance of photovoltaic for the four regions in Iraq: A practical study. Renew. Energy Environ. Sustain. 5, 3. doi:10.1051/rees/2019009
Chaichan, M. T., and Kazem, H. A. (2018). Generating electricity using photovoltaic Solar plants in Iraq. Springer. doi:10.1007/978-3-319-75031-6
Chaichan, M. T., and Kazem, H. A. (2015). Water solar distiller productivity enhancement using concentrating solar water heater and phase change material (PCM). Case Stud. Therm. Eng. 1 (5), 151–159. doi:10.1016/j.csite.2015.03.009
Chaichan, M. T., Mohammed, B. A., and Kazem, H. A. (2015). Effect of pollution and cleaning on photovoltaic performance based on experimental study. Int. J. Sci. Eng. Res. 6 (4), 594–601.
Cheema, A., Shaaban, M. F., and Ismail, M. H. (2021). A novel stochastic dynamic modeling for photovoltaic systems considering dust and cleaning. Appl. Energy 300, 117399. doi:10.1016/j.apenergy.2021.117399
Chen, G., and Zhu, Y. (2022). Advanced control for grid-connected system with coordinated photovoltaic and energy storage. Front. Energy Res. 10, 901354. doi:10.3389/fenrg.2022.901354
Chen, J., Pan, G., Ouyang, J., Ma, J., Fu, L., and Zhang, L. (2020). Study on impacts of dust accumulation and rainfall on PV power reduction in East China. Energy 194, 116915. doi:10.1016/j.energy.2020.116915
Cui, Y. Q., Xiao, J. H., Xiang, J. L., and Sun, J. H. (2021). Characterization of soiling bands on the bottom edges of PV modules. Front. Energy Res. 9, 665411. doi:10.3389/fenrg.2021.665411
Darwish, Z. A., Kazem, H. A., Sopian, K., Alghoul, M. A., and Alawadhi, H. (2018). Experimental investigation of dust pollutants and the impact of environmental parameters on PV performance: An experimental study. Environ. Dev. Sustain. 20 (1), 155–174. doi:10.1007/s10668-016-9875-7
Dhaouadi, R., Al-Othman, A., Aidan, A. A., Tawalbeh, M., and Zannerni, R. (2021). A characterization study for the properties of dust particles collected on photovoltaic (PV) panels in Sharjah, United Arab Emirates. Renew. Energy 171, 133–140. doi:10.1016/j.renene.2021.02.083
Fan, S., Wang, Y., Cao, S., Sun, T., and Liu, P. (2021). A novel method for analyzing the effect of dust accumulation on energy efficiency loss in photovoltaic (PV) system. Energy 234, 121112. doi:10.1016/j.energy.2021.121112
Fouad, M. M., Shihata, L. A., and Morgan, E. I. (2017). An integrated review of factors influencing the perfomance of photovoltaic panels. Renew. Sustain. Energy Rev. 80, 1499–1511. doi:10.1016/j.rser.2017.05.141
Ganguly, K. K., and Das, D. (2020). Analysing the barriers in Indian stone crushing industries: An ISM and fuzzy AHP approach. Int. J. Appl. Manag. Sci. 12 (3), 242–264. doi:10.1504/IJAMS.2020.108072
Gholami, A., Khazaee, I., Eslami, S., Zandi, M., and Akrami, E. (2018). Experimental investigation of dust deposition effects on photo-voltaic output performance. Sol. Energy 159, 346–352. doi:10.1016/j.solener.2017.11.010
Gil, G. M. V., Cunha, R. B. A., Di Santo, S. G., Monaro, R. M., Costa, F. F., and Sguarezi Filho, A. J. (2020). Photovoltaic energy in South America: Current state and grid regulation for large-scale and distributed photovoltaic systems. Renew. Energy 162, 1307–1320. doi:10.1016/j.renene.2020.08.022
Goel, S., and Sharma, R. (2021). Analysis of measured and simulated performance of a grid-connected PV system in eastern India. Environ. Dev. Sustain. 23 (1), 451–476. doi:10.1007/s10668-020-00591-7
Guan, Y., Zhang, H., Xiao, B., Zhou, Z., and Yan, X. (2017). In-situ investigation of the effect of dust deposition on the performance of polycrystalline silicon photovoltaic modules. Renew. energy 101, 1273–1284. doi:10.1016/j.renene.2016.10.009
Hachicha, A. A., Al-Sawafta, I., and Said, Z. (2019). Impact of dust on the performance of solar photovoltaic (PV) systems under United Arab Emirates weather conditions. Renew. Energy 141, 287–297. doi:10.1016/j.renene.2019.04.004
Hachicha, A. A., Al-Sawafta, I., and Said, Z. (2019). Impact of dust on the performance of solar photovoltaic (PV) systems under United Arab Emirates weather conditions. Renew. Energy 141, 287–297. doi:10.1016/j.renene.2019.04.004
Hassan, Q., Jaszczur, M., Przenzak, E., and Abdulateef, J. (2016). The PV cell temperature effect on the energy production and module efficiency. Contemporary Problems of Power Engineering and Environmental Protection (Gliwice, Poland), 33–40.
Jain, S., and Sawle, Y. (2021). Optimization and comparative economic analysis of standalone and grid-connected hybrid renewable energy system for remote location. Front. Energy Res. 9, 724162. doi:10.3389/fenrg.2021.724162
Javed, W., Wubulikasimu, Y., Figgis, B., and Guo, B. (2017). Characterization of dust accumulated on photovoltaic panels in Doha, Qatar. Sol. Energy 142, 123–135. doi:10.1016/j.solener.2016.11.053
Jiang, H., Lu, L., and Sun, K. (2011). Experimental investigation of the impact of airborne dust deposition on the performance of solar photovoltaic (PV) modules. Atmos. Environ. 45 (25), 4299–4304. doi:10.1016/j.atmosenv.2011.04.084
Jiang, Y., and Lu, L. (2015). A study of dust accumulating process on solar photovoltaic modules with different surface temperatures. Energy Procedia 75, 337–342. doi:10.1016/j.egypro.2015.07.378
Kaldellis, J. K., and Kapsali, M. (2011). Simulating the dust effect on the energy performance of photovoltaic generators based on experimental measurements. Energy 36 (8), 5154–5161. doi:10.1016/j.energy.2011.06.018
Kazem, H. A., Al-Badi, H. A., Al Busaidi, A. S., and Chaichan, M. T. (2017). Optimum design and evaluation of hybrid solar/wind/diesel power system for Masirah Island. Environ. Dev. Sustain. 19 (5), 1761–1778. doi:10.1007/s10668-016-9828-1
Kazem, H. A., and Chaichan, M. T. (2016). Experimental analysis of the effect of dust’s physical properties on photovoltaic modules in Northern Oman. Sol. Energy 139, 68–80. doi:10.1016/j.solener.2016.09.019
Kazem, H. A., and Chaichan, M. T. (2016). Experimental analysis of the effect of dust’s physical properties on photovoltaic modules in Northern Oman. Sol. Energy 139, 68–80. doi:10.1016/j.solener.2016.09.019
Kim, M., Kim, H., and Jung, J. H. (2021). A study of developing a prediction equation of electricity energy output via photovoltaic modules. Energies 14 (5), 1503. doi:10.3390/en14051503
Konyu, M., Ketjoy, N., and Sirisamphanwong, C. (2020). Effect of dust on the solar spectrum and electricity generation of a photovoltaic module. IET Renew. Power Gener. 14 (14), 2759–2764. doi:10.1049/iet-rpg.2020.0456
Lou, S., Yang, Y., Wang, H., Smith, S. J., Qian, Y., and Rasch, P. J. (2019). Black carbon amplifies haze over the North China Plain by weakening the East Asian winter monsoon. Geophys. Res. Lett. 46 (1), 452–460. doi:10.1029/2018GL080941
Lu, H., and Zhao, W. (2019). CFD prediction of dust pollution and impact on an isolated ground-mounted solar photovoltaic system. Renew. Energy 131, 829–840. doi:10.1016/j.renene.2018.07.112
Manju, B., Bari, A., and Pavan, C. M. (2018). Automatic solar panel cleaning system. Int. J. Adv. Sci. Res. Eng. 4 (7), 26–31. doi:10.31695/IJASRE.2018.32778
Maraj, A., Londo, A., Gebremedhin, A., and Firat, C. (2019). Energy performance analysis of a forced circulation solar water heating system equipped with a heat pipe evacuated tube collector under the Mediterranean climate conditions. Renew. energy 140, 874–883. doi:10.1016/j.renene.2019.03.109
Martín-Consuegra, F., Alonso, C., Pérez, G., Frutos, B., Guerrero, A., and Oteiza, I. (2021). Design, optimization and construction of a prototype for a thermochromic Trombe wall. Adv. Build. Energy Res. 15 (2), 161–178. doi:10.1080/17512549.2019.1684365
Meyers, B. E. (2021). “Identification of best plane-of-array irradiance sensor for PV system performance analytics,” in 2021 IEEE 48th photovoltaic specialists conference (PVSC) (IEEE), 1208–1212.
Middelhauve, L., Girardin, L., Baldi, F., and Maréchal, F. (2021). Potential of photovoltaic panels on building envelopes for decentralized district energy systems. Front. Energy Res. 9 (ARTICLE), 689781. doi:10.3389/fenrg.2021.689781
Middleton, N. J. (2017). Desert dust hazards: A global review. Aeolian Res. 24, 53–63. doi:10.1016/j.aeolia.2016.12.001
Miran, S., Tamoor, M., Kiren, T., Raza, F., Hussain, M. I., and Kim, J. T. (2022). Optimization of standalone photovoltaic drip irrigation system: A simulation study. Sustainability 14 (14), 8515. doi:10.3390/su14148515
Mustafa, R. J., Gomaa, M. R., Al-Dhaifallah, M., and Rezk, H. (2020). Environmental impacts on the performance of solar photovoltaic systems. Sustainability 12 (2), 608. doi:10.3390/su12020608
Navothna, B., and Thotakura, S. (2022). Analysis on large-scale solar PV plant energy performance–loss–degradation in coastal climates of India. Front. Energy Res. 10, 301. doi:10.3389/fenrg.2022.857948
Nkuriyingoma, O., Özdemir, E., and Sezen, S. (2022). Techno-economic analysis of a PV system with a battery energy storage system for small households: A case study in Rwanda. Front. Energy Res. 10, 957564. doi:10.3389/fenrg.2022.957564
Nour, C. A., Migan-Dubois, A., Badosa, J., Bourdin, V., Marchand, C., and Akiki, T. (2019). “Evaluation of the plane of array irradiance for a photovoltaic installation equipped with flat reflectors in different geographical locations,” in 2019 IEEE 46th photovoltaic specialists conference (PVSC) (IEEE), 1572–1577.
Nouri, B., Wilbert, S., Blum, N., Kuhn, P., Schmidt, T., Yasser, Z., et al. (2020). Evaluation of an all sky imager based nowcasting system for distinct conditions and five sites. In AIP Conf. Proc., 2303. Daegu, South Korea: AIP Publishing LLC, 180006.
Ojike, O., and Okonkwo, W. I. (2019). Study of a passive solar air heater using palm oil and paraffin as storage media. Case Stud. Therm. Eng. 14, 100454. doi:10.1016/j.csite.2019.100454
Peters, I. M., Karthik, S., Liu, H., Buonassisi, T., and Nobre, A. (2018). Urban haze and photovoltaics. Energy Environ. Sci. 11 (10), 3043–3054. doi:10.1039/c8ee01100a
Salimi, H., Mirabdolah Lavasani, A., Ahmadi-Danesh-Ashtiani, H., and Fazaeli, R. (2019). Effect of dust concentration, wind speed, and relative humidity on the performance of photovoltaic panels in Tehran. Energy Sources, Part A Recovery, Util. Environ. Eff., 1–11. doi:10.1080/15567036.2019.1677811
Schleifer, A. H., Murphy, C. A., Cole, W. J., and Denholm, P. (2022). Exploring the design space of PV-plus-battery system configurations under evolving grid conditions. Appl. Energy 308, 118339. doi:10.1016/j.apenergy.2021.118339
Shah, A. H., Hassan, A., Laghari, M. S., and Alraeesi, A. (2020). The influence of cleaning frequency of photovoltaic modules on power losses in the desert climate. Sustainability 12 (22), 9750. doi:10.3390/su12229750
Son, J., Jeong, S., Park, H., and Park, C. E. (2020). The effect of particulate matter on solar photovoltaic power generation over the Republic of Korea. Environ. Res. Lett. 15 (8), 084004. doi:10.1088/1748-9326/ab905b
Soomar, A. M., Hakeem, A., Messaoudi, M., Musznicki, P., Iqbal, A., and Czapp, S. (2022). Solar photovoltaic energy optimization and challenges. Front. Energy Res. 10. doi:10.3389/fenrg.2022.879985
Styszko, K., Jaszczur, M., Teneta, J., Hassan, Q., Burzyńska, P., Marcinek, E., et al. (2019). An analysis of the dust deposition on solar photovoltaic modules. Environ. Sci. Pollut. Res. 26 (9), 8393–8401. doi:10.1007/s11356-018-1847-z
Tamoor, M., Abu Bakar Tahir, M., Zaka, M. A., and Iqtidar, E. (2022). Photovoltaic distributed generation integrated electrical distribution system for development of sustainable energy using reliability assessment indices and levelized cost of electricity. Environ. Prog. Sustain. Energy 41 (4), e13815. doi:10.1002/ep.13815
Tamoor, M., Bhatti, A. R., Farhan, M., and Miran, S. (2022). Design of on-grid photovoltaic system considering optimized sizing of photovoltaic modules for enhancing output energy. Eng. Proc. 19 (1), 2.
Tamoor, M., Bhatti, A. R., Farhan, M., Miran, S., Raza, F., and Zaka, M. A. (2021). Designing of a hybrid photovoltaic structure for an energy-efficient street lightning system using PVsyst software. Eng. Proc. 12 (1), 45.
Tamoor, M., Habib, S., Bhatti, A. R., Butt, A. D., Awan, A. B., and Ahmed, E. M. (2022). Designing and energy estimation of photovoltaic energy generation system and prediction of plant performance with the variation of tilt angle and interrow spacing. Sustainability 14 (2), 627. doi:10.3390/su14020627
Tamoor, M., Tahir, M. S., Sagir, M., Tahir, M. B., Iqbal, S., and Nawaz, T. (2020). Design of 3 kW integrated power generation system from solar and biogas. Int. J. Hydrogen Energy 45 (23), 12711–12720. doi:10.1016/j.ijhydene.2020.02.207
Tanesab, J., Parlevliet, D., Whale, J., Urmee, T., and Pryor, T. (2015). The contribution of dust to performance degradation of PV modules in a temperate climate zone. Sol. Energy 120, 147–157. doi:10.1016/j.solener.2015.06.052
Thapar, V. (2019). A revisit to solar radiation estimations using sunshine duration: Analysis of impact of these estimations on energy yield of a PV generating system. Energy Sources, Part A Recovery, Util. Environ. Eff., 1–25. 1–25. doi:10.1080/15567036.2019.1677819
Toledo, C., Gracia Amillo, A. M., Bardizza, G., Abad, J., and Urbina, A. (2020). Evaluation of solar radiation transposition models for passive energy management and building integrated photovoltaics. Energies 13 (3), 702. doi:10.3390/en13030702
Tomin, N., Shakirov, V., Kozlov, A., Sidorov, D., Kurbatsky, V., Rehtanz, C., et al. (2022). Design and optimal energy management of community microgrids with flexible renewable energy sources. Renew. Energy 183, 903–921. doi:10.1016/j.renene.2021.11.024
Von Schneidemesser, E., Driscoll, C., Rieder, H. E., and Schiferl, L. D. (2020). How will air quality effects on human health, crops and ecosystems change in the future? Phil. Trans. R. Soc. A 378 (2183), 20190330. doi:10.1098/rsta.2019.0330
Weber, B., Quiñones, A., Almanza, R., and Duran, M. D. (2014). Performance reduction of PV systems by dust deposition. Energy Procedia 57, 99–108. doi:10.1016/j.egypro.2014.10.013
Xingping, Z., Xiaoying, Z., Yuanbo, Y., Kun, W., Wei, C., and Xiaolan, W. (2019). “Operation mode and economic analysis of concentrating solar power station,” in 2019 4th international conference on intelligent green Building and smart grid (IGBSG) (IEEE), 751–755.
Yazdani, H., and Yaghoubi, M. (2022). Dust deposition effect on photovoltaic modules performance and optimization of cleaning period: A combined experimental–numerical study. Sustain. Energy Technol. Assessments 51, 101946. doi:10.1016/j.seta.2021.101946
Zorrilla-Casanova, J., Philiougine, M., Carretero, J., Bernaola, P., Carpena, P., Mora-López, L., et al. (2011). “Analysis of dust losses in photovoltaic modules,” in World renewable energy congress-Sweden; 8-13 may; 2011 (Linköping, Sweden: Linköping University Electronic Press), 2985–2992. No. 057. doi:10.3384/ecp110572985
Keywords: particulate matter, photovoltaic system (PV system), solar irradiation, ambient air quality, PM10–2.5
Citation: Tamoor M, Hussain MI, Bhatti AR, Miran S, Arif W, Kiren T and Lee GH (2022) Investigation of dust pollutants and the impact of suspended particulate matter on the performance of photovoltaic systems. Front. Energy Res. 10:1017293. doi: 10.3389/fenrg.2022.1017293
Received: 11 August 2022; Accepted: 23 September 2022;
Published: 10 October 2022.
Edited by:
Muhammad Amjad, University of Engineering and Technology, Lahore, PakistanReviewed by:
Amjad Hussain, University of Engineering and Technology, Lahore, PakistanCopyright © 2022 Tamoor, Hussain, Bhatti, Miran, Arif, Kiren and Lee. This is an open-access article distributed under the terms of the Creative Commons Attribution License (CC BY). The use, distribution or reproduction in other forums is permitted, provided the original author(s) and the copyright owner(s) are credited and that the original publication in this journal is cited, in accordance with accepted academic practice. No use, distribution or reproduction is permitted which does not comply with these terms.
*Correspondence: Gwi Hyun Lee, Z2hsZWVAa2FuZ3dvbi5hYy5rcg==
Disclaimer: All claims expressed in this article are solely those of the authors and do not necessarily represent those of their affiliated organizations, or those of the publisher, the editors and the reviewers. Any product that may be evaluated in this article or claim that may be made by its manufacturer is not guaranteed or endorsed by the publisher.
Research integrity at Frontiers
Learn more about the work of our research integrity team to safeguard the quality of each article we publish.