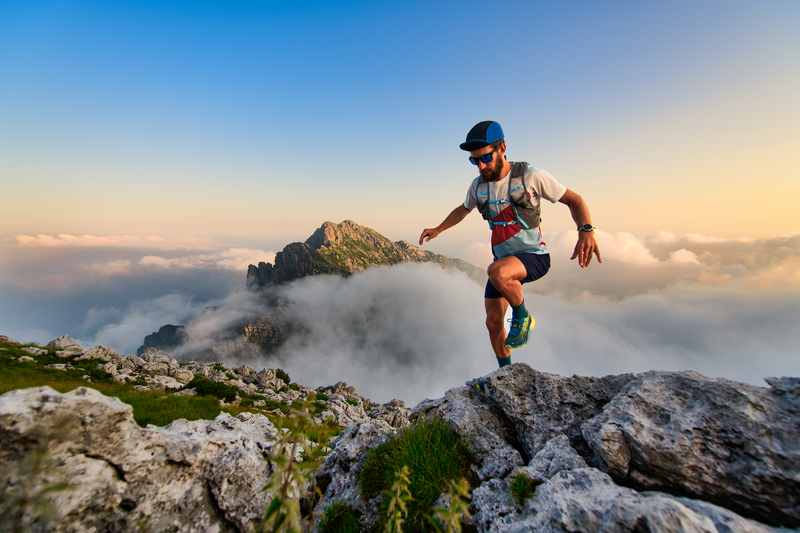
95% of researchers rate our articles as excellent or good
Learn more about the work of our research integrity team to safeguard the quality of each article we publish.
Find out more
ORIGINAL RESEARCH article
Front. Energy Res. , 09 February 2022
Sec. Process and Energy Systems Engineering
Volume 9 - 2021 | https://doi.org/10.3389/fenrg.2021.765221
This article is part of the Research Topic Emerging Sustainable and Energy-Efficient Technologies in Heat Pumps for Residential Heating View all 9 articles
In this paper, a novel direct expansion air-source heat pump heating system with a gravity-driven radiator as a heating terminal was developed, for the purpose of reducing the energy consumption and improving the thermal comfort of indoor personnel. The thermal response speed of the system at the start-up stage was experimentally studied firstly. The results showed that the thermal response speed of the developed system was slightly lower than that of a traditional air-source heat pump heating system with a convective heating terminal, but largely higher than that of an air-source heat pump floor radiant heating system. When the outdoor air temperature fluctuated between −5.2
The statistical data show that the energy used in building a heating system accounts for a large proportion of the whole building energy consumption. In 2018, urban heating energy consumption in northern China was as high as 212 million tec, accounting for 21% of the total building energy consumption in China (Building energy efficiency research center of Tsinghua University, 2020), which also led to a series of environmental pollution problems. Thus, air-source heat pump heating system has been widely used in the past few years in northern China because of its significant advantages in energy saving and environmental protection (Nishimura, 2002; Qu et al., 2012). According to the different heating media used, the air-source heat pump system can be distinguished into two forms: indirect expansion and direct expansion. The former uses hot water to transfer heat to the room, while the latter transfers heat directly to the room through the refrigerant. Depanding on the different heating terminal devices, the air-source heat pump system can also be distinguished into two types. One is the fan-coil heating terminal, in which the heat transfer is mainly dominated by convection. While the other one is the radiative heating terminal, including the traditional radiator and floor heating system, in which most of the heat is transferred by radiation. Researchers have conducted extensive research on air-source heat pump heating systems during the past decades, from the view point of energy efficiecy, thermal comfort, etc. Previous studies revealed that the forced convection heat transfer has the advantage of fast response speed and strong ability to deal with heat load, but the thermal comfort of heating is relatively poor. By using air-source heat pump heating systems with a fan-coil heating terminal, excessive vertical temperature difference and draft feeling of human body often occurs because of the large air supply speed (Wang, 2002). In addition, hot air supplied from a high height of the room will also lead to an increase in temperature at the upper zone, and reduce the utilization rate of hot air energy in the occupied zone, which eventually results in the waste of energy (Li, 2010). In contrast, the radiation heat transfer is characterized by high comfort and good stability, but the response time of indoor air temperature rise is relatively long. Imanari’s experimental result shows that radiant heating can improve thermal comfort by creating an environment with a small vertical temperature difference (Imanari et al., 1999). Through the research on the radiator heating system of air-source heat pump in an office building in Shanghai, Zhou found that the system can mitigate the human discomfort caused by cold radiation, but it is not suitable for intermittent heating due to the low indoor air temperature rise rate (Zhou et al., 2013). Through the comparison of the differences between various radiating end heating forms, Wang found that floor radiant heating is not suitable for intermittent heating mode, and the intermittent heating performance of a radiator is better than that of floor radiant heating (Wang et al., 2018).
To overcome the shortcomings of the two heat transfer modes, and maximize their respective advantages, the optimization method of integrated coupling of the two heat transfer modes can be selected. At present, many related studies have been carried out on the combination of fan coil and floor radiation, wall radiation, or radiator (Jin et al., 2017; Zhao et al., 2017). To solve the above problems, this study performs an experimental analysis on the gravity-driven radiator, and to further accelerate the heat transfer speed of gravity-driven radiator, a new gravity-driven radiator based on the coupling heat transfer of radiant-convective is proposed.
Different from the conventional air-source heat pump hot water heating system, the direct expansion air conditioning source heat pump uses liquid refrigerant as heat medium, which can effectively save the energy consumption due to the transportation of hot water. In the meantime, it can reduce the heat loss in the middle heat exchange process, and the operation of the system is shown in Figure 1A. The gravity-driven radiator proposed in this paper is a radiator heating terminal based on thermosyphon heat pipe technology, in which the working medium is alkyl dichloride and the size is 1,020
FIGURE 1. Schematic diagram of direct expansion air-source heat pump system coupled with gravity-driven radiator. (A) Direct expansion air-source heat pump coupled gravity-driven radiator heating system. (B) Vertical view and lateral view of a gravity-driven radiator.
To study the response speed of the gravity-driven radiator of the air-source heat pump coupled gravity-driven radiator system during heating in winter, an experimental platform was established in an office building in Taiyuan, Shanxi Province of China. There are two office rooms with the same size and layout, namely, A and B; both rooms have dimensions of 8
K-type thermocouples were used to measure the indoor air temperature. As shown in Figure 2, there are six measuring points arranged in horizontal direction in each room, with three heights of 0.1
The thermal performance of the gravity-driven radiator is one of the main factors influencing the thermal comfort of air-source heat pump heating systems. Figure 3 presents the surface temperature variations of the gravity-driven radiator during the start-up stage, with different outdoor temperatures. It can be found that the stable surface temperature of the gravity-driven radiator is about 45
Figure 4 compares the variations of indoor air temperature in Room A and Room B, from 08:00 to 21:00 during a typical day. It can be found that the indoor air temperature of both rooms are relatively stable within the range of 22–23.5
In the stable operation stage of the heating system, the indoor air temperature of Room A is maintained in the range of 22–22.6
It can also be found that the indoor air temperature decreasing rate in Room B is faster than that in Room A, when the heating systems are turned off. As shown in Figure 4, the indoor air temperature in Room B decreased from 22.9
To further improve the response speed of the gravity-driven radiator and meet the demand of intermittent heating, Figure 5 presents a new gravity-driven radiator with radiation and convection coupled heat transfer. Specifically, the air is sucked from the lower air outlet of the radiator by the crossflow fan, and discharged after being heated by the heat pipe wall in the ventilation channel; an adjustable deflector is equipped at the front end of the air supply outlet for the adjustment of the air supply angle; Figure 6 illustrates the geometric model of the radiator at different angles.
In this research, the authors optimize the gravity-driven radiator by CFD. Geometric modeling has to meet the requirements of geometric similarity, physical similarity, and condition similarity to get the results close to the real situation. This simulation makes a reasonable simplified calculation of the model. The length of the room is only half of the actual length, and the space dimensions of different heating systems are 4
Considering the air supply speed on the thermal comfort will also have an impact; after verifying the reliability of the model, this paper studies the indoor air temperature response speed and thermal comfort of the new gravity-driven radiator with the air-source heat pump heating system under different air supply angles and air supply speeds; a total of 12 working conditions are simulated in this study, as shown in Table 2.
The physical model is consistent with the experimental platform, and the simulation results are compared with the measured data at 14:00 on a typical day. The root mean square error (RMSE) is selected as the evaluation index. The comparison between the simulated and experimental values of the air temperature distribution at the vertical height of indoor air temperature measuring Point one and measuring Point four is shown in Figure 8, which shows that the maximum temperature difference between the simulated and measured air temperature values is no more than 1
Since the thermal sensation of the head greatly influences the overall thermal sensation of the human body (Duanmu, 2007), the 1.1-m height plane, which is the height of the head at the sitting position (hereinafter referred to as the 1.1-m height plane), will be used as the main standard for the comparison of response time and thermal comfort under different optimization schemes. Figure 9 illustrates the average temperature change curve of the 1.1-m height plane under different air angles and air supply speeds. As can be seen from the figure, when the new gravity-driven radiator is working, as shown in Figure 5, the indoor air temperature rises rapidly, and the air temperature rise rate tends to be flat after heating for a certain time. GB/T5 0785–2012 stipulates that the standard for Class II comfort of thermal environment of heating is as follows: the air speed is not lower than 0.2
The indoor air temperature changes with time under different air supply speeds and different air supply angles, as shown in Figure 9. It can be seen from Figures 1–9 that at the air speed of 0.2
It can be found that when the air supply speed remains unchanged, with the air supply angle decreasing from 90
Because the thermal environment at the terminal of the gravity-driven radiator is relatively even, there is no obvious air temperature stratification, and the layout position of radiators in the room is uniform and symmetrical. Moreover, the effects of radiation asymmetry and air speed asymmetry can almost be ignored, and the thermal environment using the gravity-driven radiator can be regarded as an ideal uniform environment. Therefore, when further analyzing the thermal comfort of indoor environment under different air supply angles and air speeds, according to the provisions of ISO7730 (International Standard, 1984), the PMV, PDD, and DR (blowing feeling index) will be taken as the evaluation indexes. The indoor Level II thermal comfort environment requires that PMV be −0.5–0.5, PDD < 10%, and DR < 20%. This paper studies the sitting posture of the human body; therefore, the three indoor height planes of 0.1
The thermal comfort evaluation results of indoor environment under different air supply angles and air supply speeds are shown in Table 3. As seen from Table 3, although the changes in air supply angle and air speed have an impact on the DR of 0.1
1) It is experimentally found that during the gravity-driven system start-up stage, the average indoor air temperature rise rate of 10.8
2) In this paper, a convection and radiation coupled gravity-driven radiator was proposed. By installing a cross-flow fan, the radiation and convection coupled heat transfer can be realized at the gravity-driven radiator, thereby significantly speeding up the response speed of indoor temperature during heating. The simulation study found that the increase in air supply speed can greatly reduce the required time, and the reduction range of the required time decreases gradually with the increase in air supply speed when the air supply angle remains unchanged.
3) Without affecting thermal comfort, the new gravity-driven radiator can be optimized with an air supply angle of −45
The original contributions presented in the study are included in the article/supplementary material, Further inquiries can be directed to the corresponding author.
HW was in charge of experimental results analysis and the writing of the paper; DW contributed to the data analysis; YC was in charge of the whole research; JJ, ZA, WP and JY participated in the experimental work; YC and PD contributed to the revision of the paper.
The authors declare that the research was conducted in the absence of any commercial or financial relationships that could be construed as a potential conflict of interest.
All claims expressed in this article are solely those of the authors and do not necessarily represent those of their affiliated organizations, or those of the publisher, the editors, and the reviewers. Any product that may be evaluated in this article, or claim that may be made by its manufacturer, is not guaranteed or endorsed by the publisher.
The authors appreciate the financial supports from the National Key Research and Development Program of China (No. 2018YFD1100701-05), the National Natural Science Foundation of China (No. 51808372), Key Research and Development (R and D) Projects of Shanxi Province (Nos. 201903D321043 and 201903D121037), the Open Foundation Program of State Key Laboratory of Green Building in Western China (No. LSKF202011), and the Postgraduate Innovation Project of Shanxi Education Department (No. 2021Y235).
Building energy efficiency research center of Tsinghua University (2020). Annual Report on China Building Energy Efficiency [R] (In Chinese). Beijing, China: China Architecture & Building Press 2020 (4).
Cheng, H., Nian, M., Wang, G., Tang, G., and Zhang, J. (2018). Measurement and Analysis of Temperature Intermittent Heating in Hefei Area[J]. Building energy efficiency 46 (8), 108–112. doi:10.3969/j.issn.1673-7237.2018.08.021
Duanmu, L. (2007). The Study on thermal Environment and comfort for Desktop-Based Task-Ambient Air conditioning[D]. Dalian, China: Dalian University of Technology.
GB/T5 0785-2012 (2012). Evaluation Standard for Indoor thermal Environment in Civil buildings[S]. Beijing: China Architecture & Building Press.
Imanari, T., Omori, T., and Bogaki, K. (1999). Thermal comfort and Energy Consumption of the Radiant Ceiling Panel System. Comparison with the Conventional All-Airsystem. Energy Build 30 (2), 167–175. doi:10.1016/s0378-7788(98)00084-x
ISO. International Standard (1984). Moderate thermal Environments-Determination of the PMV and PPD Indices and Specification of Conditions for thermal comfort. Geneva: International Standards Organization.
Jin, H., Zhang, Y., and Wu, Q. (2017). A Coupled Air-Conditioning System of Radiation and Convection Comparative Analysis of Indoor Environment[J]. Building Energ. Environ. 6, 25–29. doi:10.3969/j.issn.1003-0344.2017.06.006
Nishimura, T. (2002). Heat Pumps-Status and Trends” in Asia and the Pacific[J]. Int. J. Refrigeration 25 (4), 405–413. doi:10.1016/s0140-7007(01)00031-7
Qu, M., Xia, L., Deng, S., and Jiang, Y. (2012). An Experimental Investigation on Reverse-Cycle Defrosting Performance for an Air Source Heat Pump Using an Electronic Expansion Valve. Appl. Energ. 97, 327–333. doi:10.1016/j.apenergy.2011.11.057
Wang, E., and Tan, H. (2004). Application Study on Floor Heating System with Air Source Heat Pump in Shanghai Area[J]. Building Energ. Environ. 06, 25–29.
Wang, Y. (2002). Measurement and Analysis of thermal Environment of Heated and Heat Pump Air-Conditioned Rooms[J]. J. HV&AC 32 (3), 18–19. doi:10.3969/j.issn.1002-8501.2002.03.006
Wang, Z., Luo, M., Geng, Y., Lin, B., and Zhu, Y. (2018). A Model to Compare Convective and Radiant Heating Systems for Intermittent Space Heating. Appl. Energ. 215, 211–226. doi:10.1016/j.apenergy.2018.01.088
Wei, X., Zeng, Z., Lu, J., Wu, J., and Wei, Z. (2010). Experiment Study on Direct Floor Radiant Heating System of Air Source Heat Pump[J]. J. HV&AC 40 (7), 103–107. doi:10.3969/j.issn.1002-8501.2010.07.026
Zhao, Q., Wang, J., Feng, X., and Li, J. (2017). Influencing Factors Analysis on Cooling Capacity of Fan Coil Unit and Radiant Floor Combined System[J]. Fefrigeration And Air-Conditioning 6, 19–23.
Zhou, B., Tan, H., Wang, L., Chen, S., and Zhuang, Z. (2013). Application Study on Radiator Heating System with Air-Source Heat Pump in Shanghai Area[J]. J. HV&AC 43 (9), 83–86. Available at https://d.wanfangdata.com.cn/periodical/ChlQZXJpb2RpY2FsQ0hJTmV3UzIwMjExMjMwEg1udGt0MjAxMzA5MDE3GghwNDQ5eG85eg%3D%3D.
Keywords: air-source heat pump heating system, gravity-driven radiator, thermal response speed, thermal comfort, radiation and convection coupled heat transfer
Citation: Wang H, Cheng Y, Wang D, Peng W, Ai Z, Jia J, Duan P and Yang J (2022) Experimental and Numerical Study on Thermal Performance of a Novel Direct Expansion Air-Source Heat Pump Heating System. Front. Energy Res. 9:765221. doi: 10.3389/fenrg.2021.765221
Received: 26 August 2021; Accepted: 22 December 2021;
Published: 09 February 2022.
Edited by:
Libor Pekař, Tomas Bata University in Zlín, CzechiaReviewed by:
Wenzhe Wei, Harbin Institute of Technology, ChinaCopyright © 2022 Wang, Cheng, Wang, Peng, Ai, Jia, Duan and Yang. This is an open-access article distributed under the terms of the Creative Commons Attribution License (CC BY). The use, distribution or reproduction in other forums is permitted, provided the original author(s) and the copyright owner(s) are credited and that the original publication in this journal is cited, in accordance with accepted academic practice. No use, distribution or reproduction is permitted which does not comply with these terms.
*Correspondence: Yuanda Cheng, Y2hlbmd5dWFuZGFAdHl1dC5lZHUuY24=
Disclaimer: All claims expressed in this article are solely those of the authors and do not necessarily represent those of their affiliated organizations, or those of the publisher, the editors and the reviewers. Any product that may be evaluated in this article or claim that may be made by its manufacturer is not guaranteed or endorsed by the publisher.
Research integrity at Frontiers
Learn more about the work of our research integrity team to safeguard the quality of each article we publish.