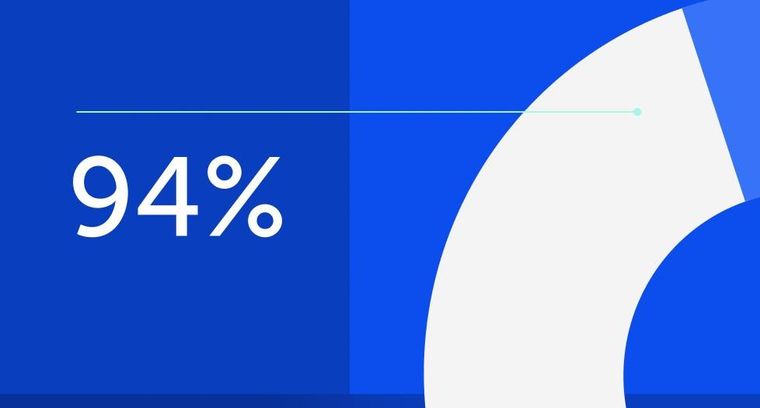
94% of researchers rate our articles as excellent or good
Learn more about the work of our research integrity team to safeguard the quality of each article we publish.
Find out more
ORIGINAL RESEARCH article
Front. Energy Res., 21 September 2021
Sec. Nuclear Energy
Volume 9 - 2021 | https://doi.org/10.3389/fenrg.2021.756106
This article is part of the Research TopicReactor Physics: Methods and ApplicationsView all 27 articles
In the design of a nuclear reactor, improving fuel utilization and extending burnup are two of the most important goals. A concept design of spectral-shift control rods is presented to extend cycle length and fuel utilization. First, a small lead-based reactor, SLBR-50, is preliminarily designed, and the design rationality is proved. Next, the concept design of spectral-shift control rods is presented and analyzed. Finally, numerical results of the small reactor design show that the burnup depth is extended by 73.3% and the fuel utilization rate for 235U and 238U is improved by 66.6 and 68.4%. All results are calculated using a Monte-Carlo code RMC. These results show advantages of the concept design for the spectral-shift control rod.
Improving fuel utilization, extending burnup depth, and improving the nuclear plant economy are several important reactor design targets (Zhang et al., 2020a; Zhang et al., 2020b). It is still an open question when it comes to extending burnup depth with certain fuel weights. The spectral-shift effect is one of the solutions for the problem.
The spectral-shift effect was studied and the spectral-shift control concept was first proposed in 1961 in the Babcock and Wilcox company report (Mars and Gans, 1961). After that, the spectral-shift effect was studied in the pressurized water reactor design (Ronen and Galperin, 1980; Ronen and Fahima, 1984; Martin, 1988) and extended to the boiling water reactor design (Yokomizo et al., 1993). In the 21st century, the spectral-shift effect research was applied in the new-type advanced reactor. In the ABWR-II core design, spectral-shift rods were adopted and analyzed (Anegawa et al., 2001; Moriwaki et al., 2004). Results show that the average discharge burnup was improved by 5% and uranium weight was saved by 6∼7%. Recently, the spectral-shift control design was applied in other advanced reactors, such as SmAHTR (Greene, 2010; Ilas et al., 2014; Kotlyar et al., 2017; Mehta and Kotlyar, 2019), small modular reactors (Lindley and Parks, 2016), and molten salt reactors (Betzler et al., 2018). SmAHTR is a small advanced high-temperature graphite-moderator reactor designed by Oak-Ridge. The cycle length can be extended by up to 20% or coated particle (TRISO) fuel can be reduced by 15% while maintaining the cycle length with the spectral-shift effect (Kotlyar et al., 2017; Mehta and Kotlyar, 2019). In these new-type advanced reactor analyses, a reactor design with spectral-shift effect shows good improvements of the fuel utilization rate and cycle length extension. However, few research studies have been carried out on spectral-shift lead-based reactors (LBRs) or the spectral-shift for extending cycle length and the fuel utilization rate.
In this study, a 50-MWt small lead-based fast reactor, SLBR-50, is conceptually designed at the Nuclear Power Institute of China (NPIC) for research. Besides, detailed parameters of the SLBR-50 are introduced. Based on the SLBR-50, the concept design of control rods with the spectral-shift effect are proposed and analyzed, including the spectral-shift effect analyses and reactor performance improvement.
A continuous-energy Monte-Carlo reactor physics code, RMC (She et al., 2013; Wang et al., 2015; Liu et al., 2017), is adopted for the small reactor design. RMC has been developed by the Department of Engineering Physics, Tsinghua University, from 2010 as a tool for a reactor core analysis platform. It has several functions, such as complicated geometry modeling, criticality calculation, burnup calculation, and critical position searching calculation. Until now, RMC has already been used for reactor design and validation. In this study, all geometry modeling and calculation results are provided using RMC.
The SLBR-50 is conceptually designed for research. Several basic parameters of the reactor are shown in Table 1. Thermal power of the SLBR-50 is 50 MW. The hot-condition average temperature is 700 K. As for materials, 19.95% enrichment UO2 is applied as the fuel. The uranium total weight is 3835.3 kg in the SLBR-50. B4C is chosen as the control rod absorber material. In each pin cell, stainless steel is conducted as the clad material. Outside of the active core, BeO is applied as the reflector and stainless steel is conducted as the barrel. Heavy metal lead is adopted as the coolant in the lead-based reactor SLBR-50. Other parameters will be introduced in geometry modeling.
The geometry modeling of the SLBR-50 is divided into the pin cell, the lattice, and the whole core. The basic pin cell geometry is shown in Figure 1. The radius of the fuel region is 4.0 mm. The thickness of the air gap between the fuel and the clad is 0.1 mm, which is hard to show in the figure. The 0.6-mm-thick clad lies outside of the pin cell.
FIGURE 1. Pin cell geometry of the SLBR-50; (A) fuel assembly, (B) reflector assembly, and (C) control rod assembly.
The hexagonal assembly geometry of the fuel, reflector, and control rod lattice are shown in Figure 2. The basic geometry is totally the same for these assemblies. 61 rods are arranged in regular matrix form. The thickness of the stainless steel assembly box is 2 mm. The assembly inner and outer distances are 88.0 and 92.0 mm separately. The assembly center distance is 93.5 mm.
The radial cut of SLBR-50 whole-core modeling is shown in Figure 3, and axial cuts of all rods out (ARO) and all rods in (ARI) cases are shown in Figure 4. The whole core consists of 144 fuel assemblies, 48 reflector assemblies, 18 control rod assemblies, and center guide tube assembly. Control rod assemblies are divided into three groups, including compensation, safety, and power adjustment. Besides, reflector assemblies are located outside of the active core. The barrel is explicitly modeled and the diameter of the barrel is 82 cm. In the axial cut, the height of the active core is 95 cm. On the top and the bottom of the active core, a 20-cm-high coolant reflector is arranged.
Nuclear design calculations for the power distribution and control rod worth were conducted using the Monte-Carlo code RMC. In the calculation, the ENDF-VII.0 library was adopted. 10 layers were divided in the axial direction of the active core. To obtain the detailed power distribution results, a relatively refined calculation condition was applied in the calculation. 8 billion active particles were used (800 generations consisting of 10 million neutrons per generation, of which 300 generations were skipped). Normalized radial assembly power and axial power distribution results of the ARO case are shown in Figures 5, 6. In the radial assembly power result, the maximum assembly power is 1.46, which lies in the center of the reactor. In the axial power distribution, the maximum power is 1.268.
In the control rod worth calculation, 60 million active particles are used (600 generations consisting of 100 thousand neutrons per generation, of which 200 generations are skipped). The control rod worth results are shown in Table 2. Three sets of control rods are calculated. Integral rod worth (IRW) results are 6,915, 4,114, and 16,022 pcm for compensation, power adjustment, and safety control rod sets separately. Both differential rod worth (DRW) and integral rod worth (IRW) results show the shutdown depth is adequate for reactor control.
Rationality of the primary design for the SLBR-50 is confirmed by these nuclear design results. Apparently, parameters in the reactor design need to be researched to improve the reactor characteristic. In this study, the spectral-shift effect is researched and analyzed based on the SLBR-50.
The basis of the spectral-shift effect is the difference of the fission absorption capacity in different energy ranges. 235U and 239Pu are dominant fission nuclides. As is shown in Figure 7, fission cross-sections of 235U and 239Pu show a similar pattern; the cross-section in the thermal energy region is larger than that in the fast energy region. Besides, 238U can absorb fast neutrons and convert to 239Pu. In this way, fuel breeding happens in the fast-spectrum reactor. Based on the fuel nuclides analysis of the different energy ranges, it can be concluded that the spectral-shift effect can balance the fuel breeding in the fast energy range and fission absorption in the thermal energy range. As a result, the fuel utilization rate will increase by the spectral-shift effect.
FIGURE 7. Diagram of the fission cross-sections for typical fuel nuclides. (A)235U fission cross-section. (B)239Pu fission cross-section.
In this study, a concept design of a control rod with the spectral-shift effect is analyzed to realize the spectral-shift effect in the small lead-based reactor SLBR-50. The concept design of spectral-shift control rods is shown in Figure 8. Three sections are divided axially, including the control rod absorber, coolant, and moderator from the top to the bottom. Compared to the traditional control rod, the spectral-shift rod adds coolant and moderator sections. In the beginning of the spectral-shift scheme, the absorber section is inserted into the core active region. Spectral-shift control rods are withdrawn in the burnup procedure. With all absorbers withdrawn outside of the active core and the coolant section raised into the core, the fast-spectral operation procedure is finished. In the fast-spectrum operation, the reactor is operated in the fast spectrum to realize fuel proliferation. After that, spectral-shift rods continue withdrawing and moderators are moving to the core. In the spectrum-shift operation, neutrons will be moderated and the energy spectrum is softened. In this way, fuel utilization can be improved with the spectral-shift effect. The control rod moving procedure is shown in Figure 9. In the SLBR-50, YH2 is applied as the moderator material.
The burnup depth and critical rod position results for the small reactor design are shown in Table 3. Three sets of control rods are withdrawn in the order of safety rods, power adjustment rods, and compensation rods. The initial rod position is −95 cm, where the control rod absorbers are totally inserted. The fast-spectrum operation is from the beginning of life to 1500 EFPD. In 1500 EFPD, all control rod absorbers are withdrawn and the spectrum-shift operation begins. The spectral-shift effect can extend the cycle length from 1500 EFPD to 2600 EFPD, which has a 73.3% improvement for the cycle length with the same fuel weight. Besides, the spectral-shift design has the same shutdown margin as the fast-spectrum design.
The kernel of the spectral-shift effect is the energy spectrum shift. Therefore, the energy spectrums are analyzed in typical burnup steps, shown in Figure 10. Energy sections are divided by an order of magnitude, from 1.0e-9 to 10 MeV. Each line shows the energy spectrum along all energy sections for the certain burnup. The energy spectrum analyses need to match with the spectral-shift rod withdrawal procedure. Besides, the thermal-spectrum result is provided in the beginning of life with all moderator rods inserted. The thermal-spectrum result can be shown as a reference in the spectrum-shift analysis. In the fast-spectrum operation from 0 EFPD to 1500 EFPD, energy spectrums are similar. Control absorber insertion has little influence on the energy spectrum. In the spectral-shift operation from 1500 EFPD to 2600 EFPD, moderators are inserted, and the spectrum-shift phenomenon can be observed in the energy spectrum analyses. The energy spectrum distribution of 2600 EFPD is similar to the thermal spectrum. It can be concluded that the spectrum-shift control rod design takes advantage of the spectrum-shift effect sufficiently in the SLBR-50.
Nuclide density variation is an important result for the spectral-shift effect. In the nuclide density analysis, only the spectral-shift scheme is conducted because that is the only scheme influenced by the spectral-shift effect. Considering the breeding of 238U to 239Pu, the fission reaction of 235U, 238U, and 239Pu is the dominant nuclear reaction in the burnup. 235U, 238U, and 239Pu are analyzed as the typical nuclides. The nuclide densities for these three nuclides are listed in Table 4, and the utilization rate variations in the burnup are shown in Figure 11.
As is shown in the table, the nuclide density of 235U and 238U is decreasing both in the fast-spectrum operation and the spectrum-shift operation, and the nuclide density of 239Pu is increasing. The phenomenon is caused by the relatively hard energy spectrum. In the burnup procedure, even though the spectrum-shift effect makes the energy spectrum softer than the original fast spectrum, the spectrum-shift effect is unable to change the harder energy spectrum in the SLBR-50. Therefore, the breeding of 239Pu is stronger than the fission absorption in the burnup procedure. All in all, the spectral-shift improves the fuel utilization of 235U and 238U from 11.5 to 19.1% and from 2.06 to 3.47%.
A 50-MWt small lead-based reactor, SLBR-50, is conceptually designed at the NPIC for research. The detailed design parameters are provided, as well as power distribution and control rod worth calculation results. These results verify the rationality of the preliminary design for the SLBR-50.
Based on the SLBR-50, the concept design of the spectral-shift control rod is analyzed for extending the burnup depth and improving fuel utilization. The basic configuration of the spectral-shift control rod is introduced in detail. Three sections are divided axially, including the control rod absorber section, the coolant section, and the moderator section. In the burnup procedure, the first section is the fast-spectrum operation. Control rod absorbers are withdrawn in this section and it is totally the same as the traditional control rod design. The second section is the spectral-shift operation with moderators inserted into the active core. The spectral-shift effect is realized in this section, and the burnup depth can be extended.
The original contributions presented in the study are included in the article/Supplementary Material; further inquiries can be directed to the corresponding author.
CZ: main researcher in the group and writer of the manuscript. LL: main researcher in the group. XP: leader in the group. BZ: researcher in the group. LW: main adviser and director.
This work is supported by the National Natural Science Foundation of China (grant nos. 12005214 and 11905214) and the China Association for Science and Technology (Young Elite Scientists Sponsorship Program 2019QNRC001).
The authors declare that the research was conducted in the absence of any commercial or financial relationships that could be construed as a potential conflict of interest.
All claims expressed in this article are solely those of the authors and do not necessarily represent those of their affiliated organizations, or those of the publisher, the editors, and the reviewers. Any product that may be evaluated in this article, or claim that may be made by its manufacturer, is not guaranteed or endorsed by the publisher.
Anegawa, T., Aoyama, M., Fennern, L. E., and Koh, F. (2001). “The Status of Development Activities of ABWR-II,” in Proc. 9th Int. Conf. Nuclear Engineering, Nice, France. ICONE-9, 8–12. April.
Betzler, B. R., Robertson, S., Powers, E. E. J. J., Worrall, A., Dewan, L., and Massie, M. (2018). Fuel Cycle and Neutronic Performance of a Spectral Shift Molten Salt Reactor Design. Ann. Nucl. Energ. 119, 396–410. doi:10.1016/j.anucene.2018.04.043
Greene, S. R. (2010). Pre-Conceptual Design of a Fluoride-Salt-Cooled Small Modular Advanced High-Temperature Reactor (SmAHTR). Tennessee: ORNL/TM-201/199.
Ilas, D., Holcomb, D. E., and Gehin, J. C. (2014). SmAHTR-CTC Neutronic Design. Kyoto, Japan: Proc Physor.
Kotlyar, D., Lindley, B. A., and Mohamed, H. (2017). Improving Fuel Utilization in SmAHTR with Spectral Shift Control Design: Proof of Concept. Ann. Nucl. Energ. 104, 53–63. doi:10.1016/j.anucene.2016.12.037
Lindley, B. A., and Parks, G. T. (2016). The Spectral Shift Control Reactor as an Option for Much Improved Uranium Utilisation in Single-Batch SMRs. Nucl. Eng. Des. 309, 75–83. doi:10.1016/j.nucengdes.2016.08.041
Liu, S., Liang, J., Wu, Q., Guo, J., Huang, S., Tang, X., et al. (2017). BEAVRS Full Core Burnup Calculation in Hot Full Power Condition by RMC Code. Ann. Nucl. Energ. 101, 434–446. doi:10.1016/j.anucene.2016.11.033
Mars, D., and Gans, D. (1961). Spectral Shift Control Reactor Design and Economic study[R]. BAW-1241. United States: Babcock and Wilcox Company.
Mehta, V., and Kotlyar, D. (2019). Core Analysis of Spectral Shift Operated SmAHTR. Ann. Nucl. Energ. 123, 46–58. doi:10.1016/j.anucene.2018.09.013
Moriwaki, M., Aoyama, M., Anegawa, T., Okada, H., Sakurada, K., and Tanabe, A. (2004). ABWR-II Core Design with Spectral Shift Rods for Operation with All Control Rods Withdrawn. Nucl. Tech. 145, 247–256. doi:10.13182/nt04-a3474
Ronen, Y., and Fahima, Y. (1984). Combination of Two Spectral Shift Control Methods for Pressurized Water Reactors with Improved Power Utilization. Nucl. Tech. 67 (1), 46–55. doi:10.13182/nt84-a33528
Ronen, Y., and Galperin, A. (1980). A Comparison between Spectral Shift Control Methods for Light Water Reactors [J]. Ann. Nucl. Energ. 7, 55–64. doi:10.1016/0306-4549(80)90007-9
She, D., Liu, Y., Wang, K., Yu, G., Forget, B., Romano, P. K., et al. (2013). Development of Burnup Methods and Capabilities in Monte Carlo Code RMC. Ann. Nucl. Energ. 51, 289–294. doi:10.1016/j.anucene.2012.07.033
Wang, K., Li, Z., She, D., Liang, J. g., Xu, Q., Qiu, Y., et al. (2015). RMC - A Monte Carlo Code for Reactor Core Analysis. Ann. Nucl. Energ. 82, 121–129. doi:10.1016/j.anucene.2014.08.048
Yokomizo, O., Kashiwai, S., Nishida, K., Orii, A., Yamashita, J., and Mochida, T. (1993). Spectral Shift Rod for the Boiling Water Reactor. Nucl. Eng. Des. 144, 223–236. doi:10.1016/0029-5493(93)90139-z
Zhang, T., Liu, X., Xiong, J., and Cheng, X. (2020). Comparisons of Reduced Moderation Small Modular Reactors with Heavy Water Coolant[J]. Front. Energ. Res. 8, 15. doi:10.3389/fenrg.2020.00027
Keywords: spectral-shift effect, SLBR-50, RMC, fuel utilization, extending burnup
Citation: Zhao C, Lou L, Peng X, Zhang B and Wang L (2021) Application of the Spectral-Shift Effect in the Small Lead-Based Reactor SLBR-50. Front. Energy Res. 9:756106. doi: 10.3389/fenrg.2021.756106
Received: 10 August 2021; Accepted: 02 September 2021;
Published: 21 September 2021.
Edited by:
Tengfei Zhang, Shanghai Jiao Tong University, ChinaReviewed by:
Shen Qu, Southwestern Institute of Physics, ChinaCopyright © 2021 Zhao, Lou, Peng, Zhang and Wang. This is an open-access article distributed under the terms of the Creative Commons Attribution License (CC BY). The use, distribution or reproduction in other forums is permitted, provided the original author(s) and the copyright owner(s) are credited and that the original publication in this journal is cited, in accordance with accepted academic practice. No use, distribution or reproduction is permitted which does not comply with these terms.
*Correspondence: Xingjie Peng, cGVuZ3hpbmdqaWV0c0AxMjYuY29t
Disclaimer: All claims expressed in this article are solely those of the authors and do not necessarily represent those of their affiliated organizations, or those of the publisher, the editors and the reviewers. Any product that may be evaluated in this article or claim that may be made by its manufacturer is not guaranteed or endorsed by the publisher.
Research integrity at Frontiers
Learn more about the work of our research integrity team to safeguard the quality of each article we publish.