- 1Swedish Rural Economy and Agricultural Society, Länghem, Sweden
- 2Department of Molecular Sciences, Biocenter, Swedish University of Agricultural Sciences, Uppsala, Sweden
Biogas production from manure is of particular value in regard of lowering greenhouse gas emissions and enhancing nutrient re-circulation. However, the relatively low energy content and the characteristics of manure often result in low degradation efficiency, and the development of operating strategies is required to improve the biogas yield and the economic benefits. In this study, the potential to enhance the performance of two full-scale biogas plants operating with cattle manure, in mono-digestion or combined with poultry manure, was investigated. Four continuously fed laboratory-scale reactors were operated in sets of two, in which the temperature in one reactor in each set was increased from 37–42°C to 52°C. The potential to increase the capacity was thereafter assessed by increasing the organic loading rate (OLR), from ca 3 to 5 kg volatile solids (VS)/ m3 and day. The processes were evaluated with both chemical and microbiological parameters, and in addition, the residual methane potential (RMP) was measured to evaluate the risk of increased methane emissions from the digestate. The results showed that both processes could be changed from mesophilic to themophilic temperature without major problems and with a similar shift in the microbial community profile to a typical thermophilic community, e.g., an increase in the relative abundance of the phylum Firmicutes. However, the temperature increase in the reactor co-digesting cattle and poultry manure caused a slight accumulation of fatty acids (2 g/l) and reduced the specific methane production, most likely due to ammonia inhibition (0.4–0.7 g NH3/l). Still, during operation at higher OLR, thermophilic as compared to mesophilic temperature slightly increased the methane yield and specific methane production, in both investigated processes. However, the higher OLR decreased the overall degree of degradation in all processes, and this showed a positive correlation with increased RMP values. Chemical analyses suggested that high RMP values (40–98 Nml gVS−1) were related to the degradation of cellulose, hemicellulose, and volatile fatty acid enriched in the digestate. Conclusively, increased temperature and load can increase the methane yield from manure but can result in less efficient degradation and increased risks for methane emissions during storage and handling of the digestate.
1 Introduction
Manure, if poorly managed, represent a source of air and water pollution; however, if well integrated into the management chain, manure can be instead be a resource (Burg et al., 2018). Using manure for biogas production has various positive effects, such as production of fossil-free energy, recycling of nutrients, and reduction of greenhouse gas emissions from agriculture (Holm-Nielsen et al., 2009; Hou et al., 2017). The potential for biogas production from cattle manure is generally high in Europe, and in Sweden the potential is estimated to ca 3–6 TWh yearly (Achinas et al., 2020; Swedish Energy Agency, 2020a). The domestic biogas production from manure in 2019 was however only ca 60 GWh in Sweden, responding to approximately in total 1,140,674 tons of manure (SOU, 2019). The production of biogas from manure is slowly increasing, but the development is slow due to difficulties in profitability (Ahlberg-Eliasson, 2018). Cattle manure has high water and fiber contents, resulting in low biogas yields and degradation efficiency, which hamper an increased use of the biogas technology in agriculture (Ahlberg-Eliasson, 2018). Moreover, manure might contain pathogens, potentially limiting the use of digested manure as fertilizer [summarized in Nolan et al. (2018)]. To reach economically feasible biogas production from manure, it is essential to find ways to improve the degradation and methane yield as well as reduce risks for pathogen spreading.
Possible ways to enhance the methane yield in manure-based biogas plants include, for example, increased operating temperature and loading rates. A common organic loading rate (OLR) in anaerobic digestion is 2–5 kg volatile solids (VS) per cubic meter active digester volume and day (Schnürer et al., 2017). Biogas plants running with liquid cattle manure typically operate at the lower level of this range, as higher loads are somewhat limited by the high water content of the manure (Ahlberg-Eliasson, 2018). However, co-digestion of manure with complementary and more energy-dense materials allows increased organic load and can improve both methane production and degradation efficiency, without significantly changing the hydraulic retention time (HRT) (Mata-Alvarez et al., 2014; Sondergaard et al., 2015; Ahlberg-Eliasson et al., 2017; Ma et al., 2020). Today, many farm-scale biogas plants in Sweden mainly operate under mesophilic conditions, i.e., 38°C–40°C (Ahlberg-Eliasson et al., 2017). However, operation at thermophilic (∼52°C) compared with mesophilic conditions has been shown to increase the degradation rate and methane yield and thus allow higher OLR and shorter HRT (Ward et al., 2008). High operational temperatures can provide additional benefits such as lower viscosity and in situ hygienization, reducing the levels of pathogens, if present (Watcharasukarn et al., 2009; Baudez et al., 2013). For biogas production from specifically cattle manure, higher methane yield has also been obtained in thermophilic compared with mesophilic conditions as well as lower residual methane production (Moset et al., 2015). Moreover, the feasibility of reaching higher OLR at thermophilic compared with mesophilic conditions has been demonstrated for cattle manure in co-digestion (Lehtomaki, 2006; Lindorfer et al., 2008a); however, it is less well investigated for mono-digestion of manure.
An issue to consider when selecting operating conditions, in addition to gas yield, is the impact on the quality of the digestate. For farmers operating biogas plants, the nutrient concentration of the digestate is highly important. In general, anaerobic digestion increases the fertilizer value compared with undigested manure, and selection of a suitable co-substrate can improve digestate nutrient levels even further (Insam et al., 2015; Monlau et al., 2015). In this regard, co-digestion with a substrate containing high levels of protein is positive, as it results in a higher ammonium–nitrogen (NH4+–N) level compared with mono-digestion of manure (Ahlberg-Eliasson et al., 2017). However, when using co-substrates with high levels of nitrogen, such as swine and poultry manure, it is important to consider the risk of ammonia inhibition, particularly during thermophilic digestion (Hansen et al., 1998; Fuchs et al., 2018). Free ammonia–nitrogen is in equilibrium with ammonium released during degradation of proteins, and this equilibrium is shifted towards ammonia with increasing temperature and pH. Inhibition of the biogas process by ammonia results in decreased degradation rates, accumulation of fatty acids, lower methane yields, and sometimes even process failure (Hansen et al., 1998; Rajagopal et al., 2013). The overall decrease in degradation rate at high ammonia levels also implies a need for longer HRT and consequently lower OLR (Westerholm et al., 2016a). The degradation efficiency of the biogas process is an important parameter determinative also of the residual methane potential (RMP) in the digestate. Previous studies investigating manure in co-digestion have shown increased RMP when the HRT decreases as a result of increased OLR (Rico et al., 2015; Ruile et al., 2015). Digestate from a well-digested manure with a low RMP produces less methane during storage than undigested manure. Production of methane from the digestate is thus a key parameter determining the greenhouse gas emissions associated with farm-scale biogas production (Amon et al., 2006; Elsgaard et al., 2016).
To promote use of manure in biogas systems and reduce greenhouse gas emissions from agricultural production, in 2014 the Swedish Government introduced a subsidy for manure-based biogas production, in line with both European Union regulations and national goals (Swedish Government, 2010; Swedish Board of Agriculture, 2013). The subsidy currently amounts to ca 40 €/MWh raw biogas produced from manure, and the amount of manure in the Swedish biogas production systems has increased since the introduction of this subsidy (Niemi Hjulfors and Edström, 2017). Estimation of methane losses from the technical equipment installed (e.g., reactor, pipes, and combined heat and power plant) is mandatory under the subsidy. However, the efficiency of the biogas utilization process with regard to the level of organic matter degraded or RMP from digestate is at present not taken into account for the subsidy (Swedish Board of Agriculture, 2015).
The overall aim of the present study was to examine the importance of operational temperature and organic load for overall anaerobic degradation of cattle manure in terms of process peformance, methane yield, digestate composition, and RMP. The hypothesis tested was that operating temperature and organic load determine both methane yield and RMP during anaerobic digestion of cattle manure, and by considering these parameters, the performance of farm-based biogas production can be improved. To test the hypothesis, we used four laboratory-scale reactors mimicking two farm-scale biogas plants co-digesting cattle and poultry manure (A) or mono-digesting cattle manure (B). In line with full-scale operation, the lab reactors were in initially operated at mesophilic temperature and in a later phase changed to thermophilic temperature. Transition from mesophilic to thermophilic temperature has been investigated before and shown possible, but so far, only a few previous studies have investigated the effects of operating temperature for manure substrates, with somewhat contradictory results (Risberg et al., 2013; Moset et al., 2015; Hupfauf et al., 2020). During reactor operation, gas yield, reduction in organic matter, RMP, and digestate composition were investigated. Moreover, the microbial community, both bacteria and methanogens, was assessed in order to also evaluate the influence of the changed operating parameters on the biological process.
2 Materials and Methods
2.1 Full-Scale Biogas Systems
Substrates and inoculum used in the experiments were collected from two biogas plants located in south-west Sweden. Biogas plant A had an active digester volume of 1,000 m3 and was running at 38°C, and the substrate used was a mixture of cattle manure, solid manure, including bedding material (i.e., straw), and poultry manure. The average OLR for plant A was 2.7 kg VS/m3 and day and the HRT was 30 days. Plant B operated at approx. 42°C, and the substrate consisted of liquid and solid cattle manure with a high dry matter content, including straw used as bedding material. Biogas plant B had a total active volume of 1,300 m3. The average OLR for the plant was 3.5 kg VS/m3 and day and the HRT was 22 days. Substrates A and B were both supplemented with an iron-rich residue [Fe concentration approx. 30% of total solids (TS)], with the main aim of reducing the level of H2S in the raw biogas by precipitation to FeS (Persson et al., 2017). For substrate A, the Fe supplement was added in the mixing tank with the liquid manure, and for substrate B, this Fe supplement was applied to the solid substrate fraction (Supplementary Tables S1–S3).
2.2 Experimental Set-Up of Laboratory-Scale Anaerobic Reactor Operation
Liquid substrates were collected directly from the mixing tank at the plant sites and from the mixing wagon for solid substrate used at plant B. The substrates were frozen at −18°C and stored for further use. After completing the second phase of the experiment (Table 1), a new batch of substrates was collected from the farm plants.
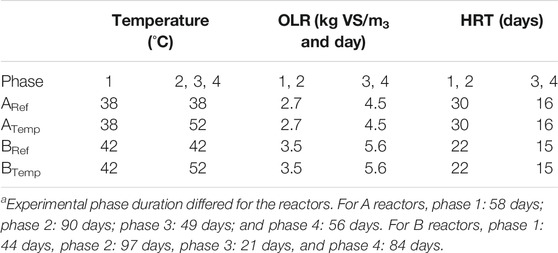
TABLE 1. Operating parameters of the continuously stirred tank reactors (CSTRs) ARef, ATemp, BRef, and BTemp during the four operating phasesa.
Four 10-L laboratory-scale continuous stirred tank reactors (CSTR) (Dolly, Belach Bioteknik AB, Sweden) were started with 5 L each of fresh inoculum from the two farm-scale plants A and B, with parallel reactors with each inoculum. For each of the reactors started with the same inoculum, one was used as a reference (ARef and BRef) and one as an experimental reactor (ATemp and BTemp). The reactors were fed once a day and for practical reasons 6 days a week and agitated continuously at 90 rpm. The average daily load corresponded to the same load as the full-scale plant, and temperature and organic load were also set to simulate the conditions in the corresponding full-scale plant (Table 1). After a 7-day start-up period to confirm process similarity between parallel digesters, the first experimental period (phase 1, 58 days in A reactors and 44 days in B reactors) was initiated, and the temperature in ATemp and BTemp was gradually increased by approximately 4°C per week until it reached 52°C. During the same period, the control reactors (ARef and BRef) were left unchanged, and the temperature remained at 38°C and 42°C, respectively. In the second phase (phase 2, 90 days in A reactors and 97 days in B reactors), all reactors were operated without any changes. In the third phase (phase 3, 49 days in A reactors and 21 days in B reactors), the OLR was increased in all four reactors by approximately 0.5 g VS/l per week until an OLR of 4.5 and 5.6 g VS/L and day was reached for reactors A and B, respectively. After reaching the final OLR, the reactors were operated at constant conditions in phase 4 (56 days in A reactors and 84 days in B reactors). The different experimental phases and their respective conditions are summarized in Table 1.
OLR and HRT were calculated according to Schnürer et al., (2017). The reduction in VS, i.e., degree of degradation (DD), was obtained as follows:
The efficiency of the biogas reactor was calculated according to Rico et al. (2015):
where MP_Vactive is the volumetric methane production from the active reactor volume (l CH4/lactive digester volume and day), HRT is the hydraulic retention time (days), and RMPtot is the total residual methane production (l CH4/ldigestate).
2.2.1 Biochemical Methane Potential
The biochemical methane potential (BMP) of the substrates was determined using the commercial system AMPTS II (Bioprocess Control, Sweden), and all samples were analyzed in triplicate. Inoculum from all reactors in the experiment was collected at the end of phase 2. The inoculum/substrate ratio was set to 3:1 (VS basis), and the organic load was 3 g VS/l. Dilution was made with tap water to final volume of 400 ml (flask volume 500 ml). Incubation temperature corresponded to the operating temperature in phase 2 of the respective reactor. The two different substrates used in reactors A and B were also analyzed using inoculum from a wastewater treatment plant (WWTP), at the same OLR and inoculum/substrate ratio, and here, the incubation temperature was set to 38°C (Table 2). The inoculum from WWTP had a TS content of 3.1% of wet weight and a VS content of 2.0%. The experiment ended when daily methane production fell below 1% of the accumulated methane production on a volume basis. The gas volumes produced were normalized to 1.01325 bar and a temperature of 273.2 K.
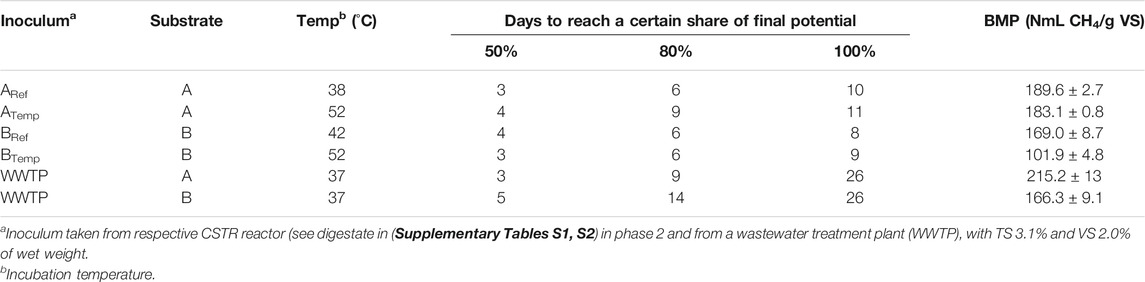
TABLE 2. Experimental set-up, biochemical methane potential (BMP), and the time taken to reach 50, 80, and 100% of the potential in anaerobic degradation of cattle and poultry manure (A) and cattle manure (B) used as substrates in the present study.
2.2.2 Residual Methane Production
Residual methane production from the digestate of all four reactors was measured at the end of phase 2 (day 140) and phase 4 (day 247). Digestate (300 ml) was added to 500-ml flasks, using the same AMPTS system as for the BMP test, but no inoculum, substrate, or water was added. All samples used for analysis were taken prior to daily feeding of the CSTRs. The incubation temperature was set to agree with the corresponding CSTR system, and the incubation was terminated after 90 days or when no more methane production was detected. For comparison, digestate samples from the end of phase 2 (day 140) were also incubated at 20°C for 200 days. This tempetature was set to evaluate the highest possible temperature in real-life post digestion under climate Swedish conditions.
2.2.3 Analytical Methods
The gas volumes from each reactor were calibrated by collecting the gas produced in bags and determining the volume using a drum meter (TG 0.5) from Ritter, Germany. The content of carbon dioxide in the raw biogas was determined 6 days a week by measuring the liquid displacement in a saccharometer filled with 7 M sodium hydroxide. Hydrogen sulfide (ppm H2S in raw biogas) and methane content (% CH4 in raw biogas) were measured weekly using the gas analyzer Biogas 5000 (Geotechnical Instruments, United Kingdom). Methane was also analyzed weekly by gas chromatography according to Westerholm et al. (2012a). All volumetric gas values in the BMP tests and from the CSTRs were converted to standard conditions at pressure 1.01325 bar and a temperature of 273.2 K.
After every HRT, a 500-ml digestate sample was taken from the CSTRs for chemical analysis. The chemical composition of substrate and digestate was analyzed in the laboratory of the Department of Animal Nutrition and Management, Swedish University of Agricultural Sciences, Uppsala, Sweden. Concentration of neutral detergent fiber was analyzed according to Chai and Uden (1998). Acid detergent fiber and acid detergent lignin were determined according to Van Soest et al. (1991). The concentrations of hemicellulose and cellulose were calculated from the analyzed values of neutral detergent fiber, acid detergent fiber, and acid detergent lignin according to Van Soest et al. (1991). Starch and water-soluble sugars were analyzed by an enzymatic method according to Larsson and Bengtsson (1983), originally based on Mannheim (1984). Free glucose was subtracted and not included in the starch content. Minerals [phosphorus (P), calcium (Ca), magnesium (Mg), sodium (Na), sulfur (S), iron (Fe), copper (Cu), manganese (Mg), and zinc (Zn)] were analyzed according to Balsberg-Påhlsson (1990) using the spectrophotometer Spectro Blue FMS 26 (Spectro Analytical Instruments, Kleve, Germany). Digestate samples for TS and VS analysis were taken once in the beginning of the experiment and then once every HRT. The analysis was performed by drying according to APHA (1998). Short-chain volatile fatty acids (VFA) in the digestate from the four CSTRs were quantified on a weekly basis (except for ARef, BRef, and BTemp in weeks 15, 19–23, 27, 30, and 33 and ATemp in week 24). One biological sample per time point was analyzed by ion-exclusion chromatography according to Westerholm et al. (2012a). The pH in the CSTRs was monitored over the whole experimental period by weekly measurements using a bench pH-meter (3510 pH meter, Jenway, United Kingdom).
2.3 Statistical Treatment
The biogas production, specific methane potential, VFA content, degree of degradation, H2S, CH4, and pH data were statistically evaluated pair-wise for the reactors and between the experimental periods, using the t-test procedure in R version 3.4.0 (2017-04-21). The p-values was presented as significant at ***p < 0.001, **p < 0.01, and *p < 0.05) and not significant (ns) at p > 0.10).
2.4 Illumina Sequencing and Quantitative Polymerase Chain Reaction Analyses
Reactor samples (15 ml) from all four CSTR were collected at six different occasions, to create a time series corresponding to the different phases of operation. Then, 200 µl were used for DNA extraction according to the manufacturer’s protocol (60 µl elution volume) with some minor modifications (Danielsson et al., 2017). Construction of 16S rRNA gene amplicon libraries was done, and Illumina MiSeq sequencing was carried out on triplicate samples from each sampling point using primers 515′F and 805R as described previously (Westerholm et al., 2020). Data analyses and data organization were conducted using the software package Divisive Amplicon Denoising Algorithm 2 (DADA2) version 1.6.0; the phyloseq package as described in Westerholm et al. (2020) was used for processing; the forward and reverse reads were truncated at positions 250 and 200, respectively; and taxonomy classification was conducted using the Silva training set v. 132. The graphic was produced using the plot_bar function [ggplot2 package, (Wickham, 2016)] in R Studio software (Team, 2016). For ordination plot, the Bray–Curtis metric was used for calculation of beta diversity with the ordinate function, while a non-metric multidimensional scaling (NMDS) plot was constructed with plot_ordination functions (phyloseq package). Reads were assigned at species level using the BLAST algorithm (Altschul et al., 1990) provided by the National Center for Biotechnology Information (NCBI http://www.ncbi.nlm.nih.gov). Raw sequences were submitted to the NCBI Sequence Read Archive (SRA) under study accession number PRJNA638826.
For quantitative polymerase chain reaction (qPCR) analyses, extracted DNA was diluted 10x, 50x, 100x, and 500x and used for an initial inhibition test. For the actual quantification assay, technical triplicates of the 50x and the 100x dilutions were chosen for all DNA samples, and qPCR analyses were carried out on the CFX Connect™ Real-Time System (Bio-Rad, California, United States). The methanogenic orders Methanobacteriales and Methanomicrobiales and the families Methanosarcinaceae and Methanosaetaceae were analyzed in all four reactors using the primer sets MBT, MMB, Msc, and Mst, respectively (Yu et al., 2005). The reference strains used for creation of the MBT, MMB, Msc, and MSt standard curves were Methanobacterium bryantii (DSM 10113), Methanoculleus bourgenis strain MAB1, Methanosarcina bakeri (DSM 800), and Methanosaeta concilii (DSM 2139), respectively. In the assays, the PCR efficiency varied between 86% and 97%, and a linear correlation coefficient (r2) between 0.996 and 0.998 was obtained. The qPCR reaction mixtures and thermal cycle programs used were in accordance with Westerholm et al. (2012b).
3 Results and Discussion
3.1 Substrate Characteristics
Chemical analysis revealed that the concentrations of cellulose, hemicellulose, and lignin were higher in the substrate mix used in biogas plant B compared with plant A (Supplementary Tables S1–S3). The higher content of these components in substrate mix B was most likely due to the fraction of solid manure. However, the levels were still in line with those reported in other studies evaluating liquid cattle manure (Labatut et al., 2014). Another difference in substrate characteristic was the higher protein and ammonium concentrations in substrate A, 30% of TS and 2.4 g NH4+–N/l (wet weight), respectively, compared with 23% of TS and 1.7 g/l, respectively, in substrate B. Poultry manure was included in substrate A, which probably explains the higher ammonium and crude protein concentrations compared with substrate B. Poultry manure contains a relatively high amount of organic nitrogen, which is mineralized to ammonium in the manure during storage (Chadwick et al., 2011; Yenigün and Demirel, 2013). For substrate mix B, an analysis of the liquid and solid manures was also performed separately, and in this case, the results showed that the liquid manure supplied the substrate mix with most of the nutrients and trace metals (Supplementary Table S3).
3.2 Substrate Methane Production Potential
The BMP test for the substrates showed values between ca 100 and 200 ml CH4/g VS, depending on inoculum used in the test (Table 2). Substrate B showed values in line with previous research on cattle manure, showing a wide span of values (∼150–250 ml CH4/g VS) (Labatut et al., 2011; Moller et al., 2004; Triolo et al., 2011). Substrate A had a slightly higher BMP value as compared to substrate B (Table 2), which is in line with the content of poultry manure. Compared with cattle manure, poultry manure have been reported to have somewhat higher BMP values (180–280 ml CH4/g VS) (Huang and Dhih, 1981; Pratt et al., 2014; Ruile et al., 2015). The BMP test of the substrate mixes A and B, using inoculum from the reference or the experimental reactor, revealed no influence by temperature on the degradation of substrate mix A, whereas for substrate B, the BMP was significantly (p < 0.05) higher at 42°C than at 52°C. The substrates were also digested using an inoculum taken from a WWTP. In this test, substrates A and B both gave higher BMP values than in the test using inoculum from the laboratory reactors. However, the time to reach the final potential was considerably longer when using this inlucoum as compared to the other tests (Table 2).
Although several norms and guidelines for BMP tests exist, interlaboratory tests regularly show high variability in BMP for a particular substrate (Holliger et al., 2016). This variation depends in part not only on differences in the composition of the substrates evaluated but also on the experimental set-up. In this study, the test was set-up according to guidelines for BMP tests, using a substrate/inoculum ratio of 1:3 on a VS basis and an organic load of 3 g VS/l day (Schnürer et al., 2017). However, using different inocula still gave different results, with lower values for the tests started with inoculum from the reactors compared to inoculum from WWTP. The importance of inoculum source for the results in batch tests has been reported previously and most likely depends on differences in microbial composition/activity and functional diversity (Raposo et al., 2009; Cordoba et al., 2016; Sun et al., 2016; Liu et al., 2017). Interestingly and of relevance to the present work is that several studies have shown lower BMP values of manure when determined in tests initiated with inoculum taken from a process before as compared to after operation with manure, respectively (Risberg et al., 2013; Liu et al., 2017). This suggests that the BMP of manure will be low when determined using inoculum from a manure-based process, as found in the present study. It also suggests that manure may include compounds that accumulate over time and act as inhibitors of degradation. Moreover, previous studies using inoculum from WWTP in BMP tests have shown good efficiency for degradation of specifically lignocellulose (Sun et al., 2016; Liu et al., 2017). This could explain the larger difference in the test using different inoculum for substrate B, with a fraction of solid manure with high fiber content, compared with substrate A (Table 2).
3.3 Impact of Increased Temperature on Gas Yields and Process Performance
3.3.1 Gas Production and Stability
Before the temperature increase started, all reactors showed a similar performance with regard to gas production, gas quality, and low VFA values (>0.5 g/l) (Figure 1; Supplementary Figure S1; Table 3). In line with the BMP values for the substates, A reactors showed higher gas production compared to B reactors (Figure 1; Table 3). The change in temperature in phase 1, e.g., gradually increased to 52°C in ATemp and BTemp, did not influence the processes, and both reference and experimental reactors showed no signifcant difference in gas production or degree of degradation (Figure 1; Table 3). However, in phase 2, when the experimental reactors were operated at a higher temperature, some difference could be seen, with Atemp showing a significantly higher level of VFA and lower gas production as compared to the mesophilic reference reactor (Supplementary Figure S1; Table 3). The temperature in biogas digesters can be increased in several steps, as in this study, or in one step (Bousková et al., 2005; Chae et al., 2008; Tian et al., 2015; Westerholm et al., 2018). Stepwise increases are recommended as a suitable approach, as this can allow the organic load to be maintained during the temperature shift period (Lindorfer et al., 2008b; Risberg et al., 2013). However, previous research has also shown a drop in biogas production around 42°C–44°C when performing a stepwise temperature increase (Bousková et al., 2005; Lindorfer et al., 2008b; Westerholm et al., 2018). This effect could not be statistically confirmed in the reactors evaluated in this study (Table 3). The result was instead more in line with Hupfauf et al. (2020), demonstrating similar biogas production from a mixture of liquid cattle manure and wheat straw during operation at both 37°C and 45°C or 55°C.
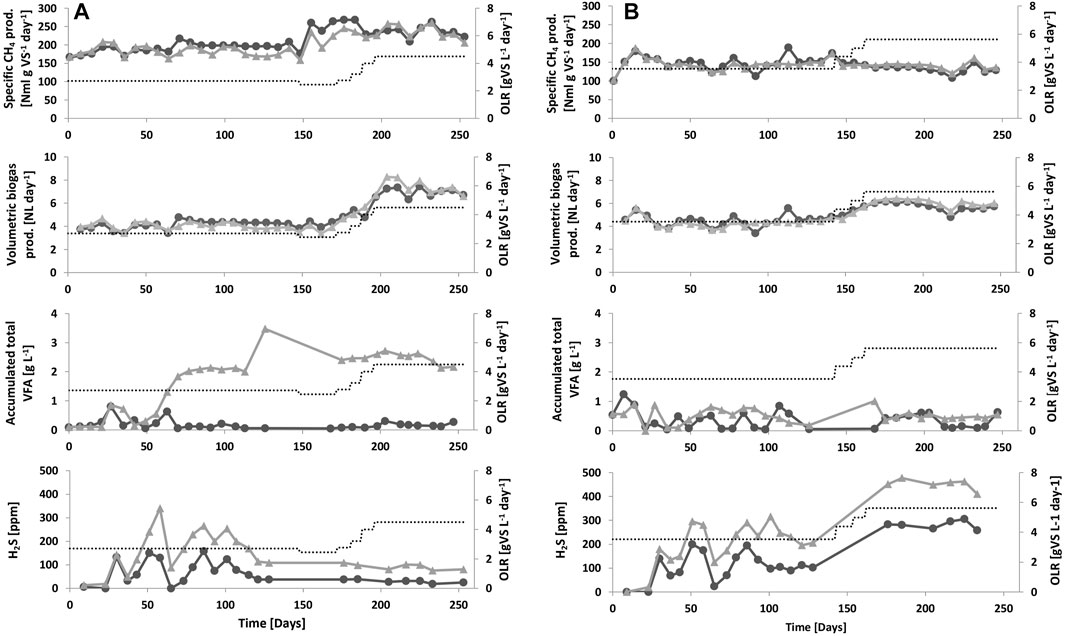
FIGURE 1. Performance of the investigated reactors, e.g., specific methane (CH4) production, volumetric biogas production, total volatile fatty acids (VFA), and hydrogen sulfide (H2S) in raw biogas. Organic load (OLR) is represented by a dotted line. (A) Reactors ARef (black line) and ATemp (gray line). Phase 1: days 1–58, phase 2: days 59–148, phase 3: days 149–197, and phase 4: days 198–253. (B) Reactors BRef (black line) and BTemp (gray line). Phase 1: days 1–44, phase 2: days 45–141, phase 3: days 142–162, and phase 4: days 163–246.
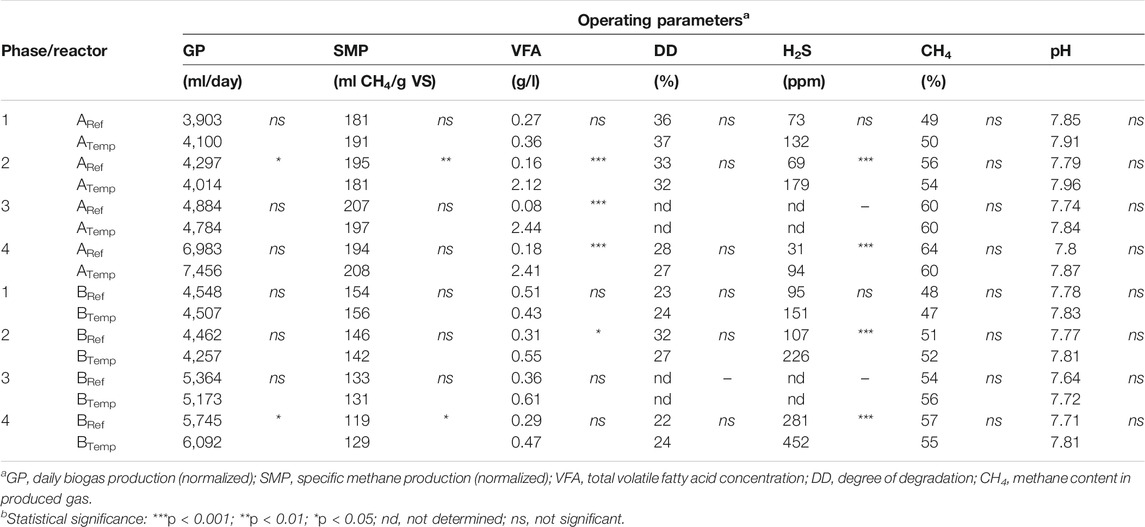
TABLE 3. Biogas reactor performancea parameters during the four phases of operation involving increased temperature to 52°C in ATemp and BTemp (phase 1), adaptation to new temperature conditions (phase 2), increased OLR (phase 3), and the final operation at constant parameters (phase 4). In each phase the experimental and reference reactor was statistically comparedb.
Previous studies that have investigated changing operational temperature during biogas production from cattle manure have either shown small effects or increased specific methane potential with increasing temperature (Moset et al., 2015; Risberg et al., 2013). In the present study, the reactors operating with cattle manure only showed no effect of the temperature change (B reactors, Table 3). However, contradictory results were obtained for A reactors, fed with a combination of cattle and poultry manure. In this reactor, the increase in temperature resulted in accumulation of acetate and propionate and a decrease in the specific methane potential (Figure 1; Table 3), suggesting a slight disturbance (Marchaim and Krause, 1993). This difference between the A and B reactors was likely caused by differences in substrate composition and the high nitrogen concentration in the substrate mixture used in the A-system (Supplementary Table S1). Earlier studies have shown that biogas processes operating with poultry manure typically are characterized by high ammonia concentrations, originating from degradation of protein and from hydrolysis of urea (Rajagopal et al., 2013; Tian et al., 2015). Calculations on the level of free ammonia in the investigated reactors according to the formula by Hansen et al. (1998) showed values of 0.7 and 0.2 g NH3/l for ATemp and ARef, respectively. The levels in the thermophilic process were clearly above those previously reported to cause inhibition (0.4–0.7 g NH3/l) and are likely the explanation to the observed decreased specific methane potential as compared to the mesophilic process (Rajagopal et al., 2013; Westerholm et al., 2016a). The B reactors, receiving only cattle manure, showed moderate ammonia levels (<0.3 g NH3/l), and, in line with this, there was also no difference in daily biogas production and specific methane potential between the reactors operating at different temperatures (Table 3; Supplementary Table S5). As a consequence of high ammonia concentration, an imbalance might occur between the different degradation steps and consequently VFA can accumulate. This was also clearly seen in ATemp where VFA accumulated to 2 g/l during the temperature increase, which was not seen in BTemp (Figure 1; Supplementary Figure S1). The VFA in ATemp mainly consisted of acetate and propionate, with an initially increasing ratio of propionate to acetate stabilizing at the end of phase 3 (Supplementary Figure S1), which can be an early sign of risk of process failure (Marchaim and Krause, 1993).
3.3.2 Gas Composition
There was no significant change in the methane content of the biogas in response to the change in temperarature (Table 3), and levels were as expected for manure-based biogas production (Alhgren-Eliasson et al., 2017). The concentration of H2S in the raw biogas was also in line with reported values for manure-based biogas systems (Table 3; Figure 1) (Weiland, 2010). However, it is notable that for both the A and B processes, the concentration of H2S in the raw biogas was significantly higher for the systems running under thermophilic conditions (Table 3), likely due to less H2S being dissolved in the liquid phase at thermophilic compared with mesophilic temperatures. Another alternative explanation could be increased activity of sulfate-reducing bacteria, producing H2S as end product of their metabolism, at the higher temperature. However, a previous survey of different biogas plants illustrated the opposite, e.g., slightly lower levels of sulfate-reducing bacteria at thermophilic compared to mesophilic conditions, which appears not a likely explanation (Moestedt et al., 2013). High levels of H2S can result in disturbance in the biogas process directly, or indirectly, by precipitation of important trace metals and inhibition of the microbial community (Chen et al., 2008; Rasi et al., 2011; Choong et al., 2016). In addition, H2S in the raw biogas can cause corrosion problems in the technical equipment at the biogas plant, such as heat and power plant units (Rasi et al., 2011). Therefore, it is highly important to reduce the levels of H2S in the biogas produced. In this study, both substrate mixes were complemented with a ferrous-rich sludge material in order to reduce H2S levels (Persson et al., 2017). Addition of iron in excess reduces the levels of H2S by precipitation of FeS and releases trace metals otherwise trapped as metal sulfides. Trace metals, in available forms, are essential for microbial activity and have been shown in a number of studies to boost biogas production and VFA degradation from various substrates, including manure (Rasi et al., 2011; Choong et al., 2016; Zhang et al., 2016). The iron added to the substrate used in this study was sufficient to keep the H2S levels low in the raw biogas, but there were still higher concentrations in the gas produced from the reactors running at thermophilic conditions (Table 3; Figure 1). A higher dosage of Fe and trace metals might have improved the performance of the thermophilic process, in particular the A process operating with poultry manure.
3.3.3 Degree of Degradation
Even though ATemp showed lower gas production compared to ARef in phase 2, no differences between these reactors were observed for the degree of degradation (Table 3). All A and B reactors showed values of around 30% degradation of organic matter, which is in line with previous findings for mesophilic full-scale biogas plants digesting manure (Fontana et al., 2016; Ahlberg-Eliasson et al., 2017). The overall chemical composition of the digestate for the respective reactors operating at different temperatures was also relatively similar (Supplementary Tables S1, S2). However, comparative analyses of substrate and digestate suggested a more efficient conversion of carbohydrates as compared to protein in all processes, and the mineralization of organic nitrogen to ammonium was only around 2%.
3.4 The Effect of Increased Organic Loading Rate on Process Performance
3.4.1 Gas Production and Stability
During the OLR increase and following stabilization phase (phases 3–4), the daily biogas production for both the A and B reactors increased compared to the previous period (Table 3). The increase was most pronounced for the A reactors reaching 40%–50% higher yields as compared to the period with lower OLR (Table 3). The thermophilic reactor showed slightly higher values as compred to mesophilic operation, but the difference was not significant (Table 3). For the B reactors, the increase in daily biogas production in response to increased OLR was comparably lower (10%), but here, gas production (GP) was significantly (p < 0.05) higher at the thermophlic temperature (Table 3). Moreover, the specific methane potential was significantly higher for BTemp compared with BRef, suggesting a higher degradation rate at the higher temperature (Table 3; Supplementary Table S5). No VFA accumulation was seen in the B reactors or Aref, and in the ATemp reactor, the VFA accumulation did not escalate further as compared to before the OLR increase (Table 3). The increase in volumetric biogas production in response to higher organic load is in line with previous studies of pig manure and cow manure as substrates for biogas production, in mono- or co-digestion and using mesophilic and/or thermophilic operational temperatures (Lindorfer et al., 2008a; Ning et al., 2019). This clearly illustrates that an increase in OLR represents a great potential for a significant capacity increase for manure-based biogas plants. However, to avoid increased risk for methane emissions from digestates, it is important to secure that the overall degradation is not decreased due to the shortening of HRT in response to the OLR increase. In previous studies, the measured specific methane potential in response to increase in load varies between studies, with slightly higher values or no influence at all (Lindorfer et al., 2008a; Li et al., 2015; Moset et al., 2015) or decreasing values (Adebayo et al., 2018; Ning et al., 2019; Hupfauf et al., 2020). The discrepancy in results is likely depending on several different parameters, such as OLR, HRT, and substrate composition as well as operational temperature.
3.4.2 Degree of Degradation and Gas Composition
In the present study, the B reactors showed significantly lower specific methane potential at the higher OLR as well as degree of degradation in the case of Bref (Table 3). In contrast, the A reactors did not shown a decrease in specific methane potential with the increase in OLR (Table 3). Still, a significantly (p < 0.01) lower degree of degradation was shown for Atemp in phase 4 as compared to phase 2. The non-significant difference in the specific methane potential, despite the lower degree of degradation, can be explained by a slight increase of methane content in the gas at the higer organic load (Table 3). Considering all reactors, the overall degree of degradation showed a negative correlation with OLR (k = −0.75) (Supplementary Table S5). A similar negative correlation between OLR and the degree of degradation was also seen during an evaluation of 31 full-scale farm reactors in Sweden (Ahlberg-Eliasson et al., 2017).
Furthermore, comparing digestate composition from phases 2 and phase 4 indicated that the observed decrease in the degree of degradation in Bref in response to the increase in OLR was caused by a less effeicent degradation of cellulose and hemicellulose (Supplementary Table S2). For the A system, the decrease in degradation efficiency was instead likely caused by a slight decrease in digestibility of proteins as indicated by a lower concentration of NH4+–N in the digestate in phase 4 compared to phase 2 (Supplementary Table S1). A decreased degree of degradation of proteins, in response to the higher load in the A reactors was also suggested by the concetration of H2S in the gas, which was significantly lower for reactor A in phase 4 compared with phase 2 (Table 3). On the contrary, the H2S level and the concentration of NH4+–N in reactor B was similar or slightly higher in phase 4 as compared to phase 2. In line with the results for the A system, decreasing TAN levels in response to increasing OLR (3.5–4.4 g VS/l day) and decreasing HRT (from 20 to 16 days) were also observed by Moset et al. (2015), at both mesophilic and thermophilic temperatures, using cattle manure as substrate. The same results were also obtained during biogas production from cow manure mixed with dog food, but here, a difference was seen between temperatures, with a comparably higher degradation rate of proteins under thermophilic conditions during the OLR increase (Labatut et al., 2014). A temperature effect could not be demonstrated in the present study, even though both ATemp and BTemp showed slightly higher values of NH4+–N g/l compared with the reference reactors for both phases 2 and 4 (Supplementary Tables S1, S2).
3.5 Residual Methane Potential and Efficiency
3.5.1 Residual Methane Potential in the Different Operational Phases
The residual methane potential for the different reactors spanned between 40 and 98 Nml gVS−1, which was within the range measured in previous studies on manure-based processes, showing values from 20 to 240 ml CH4/g VS (Monalu et al., 2015; Moset et al., 2015; Ruile et al., 2015). The observed variations may be explained not only by differences in digestate composition and chosen operational conditions but also by different methods used to determine RMP, with variations in, e.g., incubation temperature and time, and the use of inoculum or not in the incubations (Monalu et al., 2015; Thygesen et al., 2014). The RMP in the present study varied between reactors A and B and with different results depending on operating temperature, OLR, and also incubation temperature during the test. In A reactors, the higher operating temperature, at both OLR, resulted in a comparably higher RPM, irrespective of incubation temperature during the test. On contrary, the RMP levels for the B reactors were similar or slightly lower for BTemp than for BRef, independent of ORL and incubation temperature (Table 4). These results were contradictory to a study by Moset et al. (2015) where a higher residual methane production was found for a mesophilic compared to a thermophilic process operating with cattle manure and when incubation was made at respective reactor temperatures, e.g., at 35°C and 50°C (Moset et al., 2015). The authors of that study proposed that the resulting digestate from the mesophilic digestion contained a comparably higher amount of undigested biodegradable VS, representing a source for residual methane production. This was apparently not the case in the present study. Previous studies have also shown that residual methane production is decreasing at high ammonium–nitrogen level (>2.7 g/l N–NH4+), tentatively due to inhibition of methanogenesis [reviewed in Monlau et al. (2015)]. However, in the present study, the RMP levels were similar in A and B reactors, even though the ammonium–nitrogen level was comparably higher in the A reactors. For both reactors, the RMP from phase 4 was slightly higher than that from phase 2, likely as a result of the lower degradation in the reactors as a consequence of the increase and decrease in OLR and HRT, respectively (Supplementary Figure S1; Table 3). Indeed, a positive correlation between OLR and RMP and a negative correlation between HRT, as well as efficiency value, and RMP were observed (Supplementary Figure S1; Supplementary Table S5). This result was also in line with previous studies evaluating full-scale biogas plants with cattle manure and maize silage as main substrates (Lindorfer et al., 2008a; Ruile et al., 2015). Calculating the efficiency values by considering both the gas production and the RMP according to Rico et al. (2015) showed slightly higher values for the A system compared with the B system. Also, for all reactors, the efficiency value decreased in phase 4 compared with phase 2, indicating a decrease in degradation efficiency after the OLR increase (Table 4). Thus, increasing OLR increased the risk for methane emission during post storage in all reactors (Table 4). However, when RMP was determined at 20°C, which is the highest temperature likely to be reached in a storage under Swedish climate conditions, the RMP for the B reactors decreased (Table 4). Still, the values obtained for the A reactors at this temperature were similar to those determined during incubation at 38°C and 52°C, showing that a lower temperature does not always reduce the methanogenic activity. This result was in line with a recent modelling study, which suggested that CH4 emissions cannot be predicted from VS and temperature alone and that the methanogenic potential changes during storage (Baral et al., 2018).
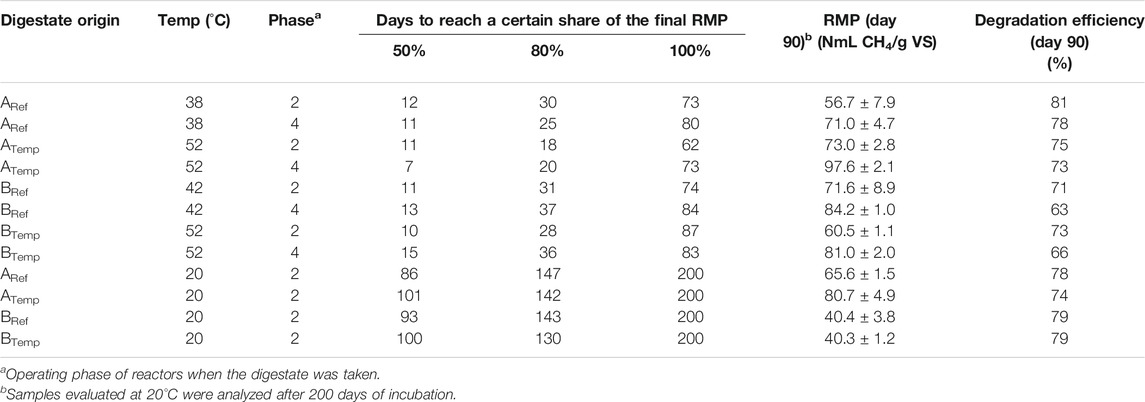
TABLE 4. Experimental set-up and results of analyses of digestate residual methane potential (RMP) and the incubation time to reach 50, 80, and 100% of this potential in digestate taken during operating phases 2 and 4.
3.5.2 Origin of Residual Methane Potential
Considering all reactors, the RMP was highest in ATemp, and according to chemical analyses, this seemed to arise mainly from degradation of cellulose and hemicellulose (Supplementary Table S6). The protein concentration as a percentage of TS instead increased during the incubation, suggesting that the degradation of protein was comparably less efficient. Still, proteins were degraded to some extent as levels of NH4+–N increased slightly during the RMP test incubation (Supplementary Tables S1, S6). Gas was probably also produced from degradation of VFA, accumulated in the ATemp reactor. For the B reactors, the RMP was generally higher for phase 4 and appeared independent of the initial operational temperature of the reactors. During the inoculation of digestate from these reactors, no degradation of proteins occurred, and in addition, the fraction of hemicellulose also seems to be stable. From this digestates, the major gas production seems to arise mainly from the degradation of cellulose (Supplementary Table S6). The recalcitrance of protein structures as shown in the present study was in line with the findings of a recent study investigating the residual particulate organic matter in nine full-scale anaerobic digesters (Yekta et al., 2019a). By comparing the structural compositions of substrate and digestate in this study, an enrichment of protein structures relative to the carbohydrates was observed in most cases, suggesting a preferential degradation of the carbohydrates over proteins, alternatively an increase of microbial biomass during the process.
3.5.3 Efficiency
The levels of RMP are important to consider when evaluating the risk of greenhouse gas emissions from digestate. Earlier research has discussed the importance of sufficient retention time and post digestion time in full-scale biogas plants to reduce the risk of methane production from digestate (Angelidaki et al., 2005; Bacenetti et al., 2016). However, it is clear from the results in this study and those of others that RMP values can vary depending on the method used for the measurment. Further complicating matters, the storage conditions, i.e., crust, temperature, and volume, of digestate before field application strongly affect the greenhouse gas emissions from the digestate and have to be taken into account before practical use of the RMP values (Rhode et al., 2012; Lebuhn et al., 2014). The ways in which different operating parameters, substrates, and storage conditions affect the RMP need to be determined more accurately, in order to fully understand and calculate the risk of methane leakage. Calculation of efficiency values according to Rico et al. (2015) seems likely to be a promising tool to describe and assess the performance of different biogas processes. This calculation agreed well with the results for the reactors in the present study, e.g., the higher daily biogas production, specific methane potential, and degree of degradation in the A system compared with the B system (Table 3).
3.6 The Impact of Increased Temperature and Organic Loading Rate on Microbial Communities
3.6.1 Initial Microbial Community Profile and the Effect of Down-Scaling
The amplicon sequencing of 16S rRNA genes of the inoculums used to seed the reactors showed community profiles in line with previous studies on manure-based biogas processes (Sun et al., 2015; Ozbayram et al., 2018; Hupfauf et al., 2020). Both inoculums were dominated by phylum Firmicutes and Bacteroidetes, still with some variation on lower taxonomic rank. In addition, inoculum B contained high relative abundance of phylum Candidatus Cloacimonetes. On family level, inoculum for the A reactors demonstrated dominance of the families Ruminococcaceae, Caldicoprobacteraceae (phylum Firmicutes), and uncultivated members of the order Sphingobacteriales (phylum Bacteroidetes, Figure 2; Supplementary Figure S3). In the inoculum used for the B reactors, Caldicoprobacteraceae and uncultivated members of MBA03 instead dominated (phylum Firmicutes), and Cloacimonadaceae was abundant in phylum Ca. Cloacimonetes (Figure 2).
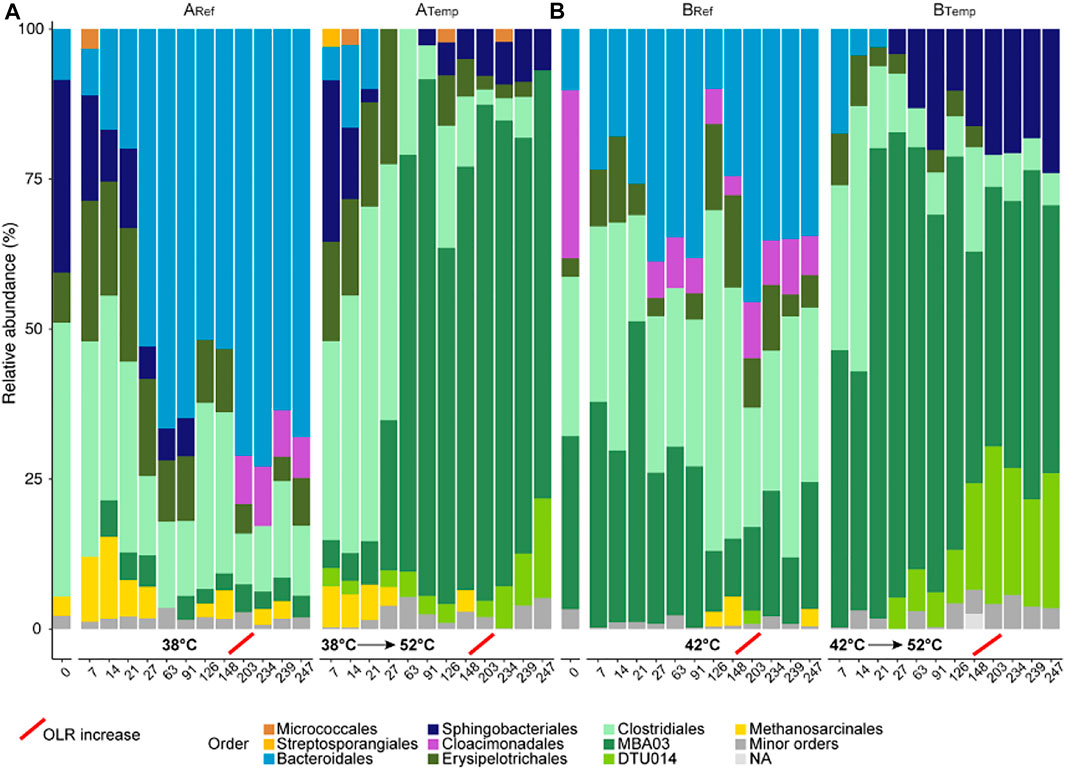
FIGURE 2. Relative abundance of microbial order (based on total bacterial and archaeal sequences) in the reference (ARef and BRef) and the reactors with increasing temperature (ATemp and BTemp). (A,B) Inocula used to seed the lab-scale reactors. Brown, blue, purple, and green bars represent orders within the phyla Actinomycetales, Bacteriodetes, Cloacimonetes, and Firmicutes, respectively. Yellow indicates methanogenic archaea. Orders representing <1% were included in minor orders. Red lines indicate the point for increased organic loading rate (OLR) in the reactors. Operating day and temperature at the point of sampling are given on the x-axis.
Despite that the reference reactors operated under constant parameters, and similar to the full-scale reactors from which the inoculums were taken, quite drastic dynamics were observed in the microbial community under the first phase of operation (Figure 2; Supplementary Figure S3). These changes were thus likely related to altered conditions associated with down-scaling the processes, including changed feeding interval, which previously has been shown to influence performance and bacterial structure (Mulat et al., 2015; Westerholm et al., 2019). Transition to lab-scale conditions promoted the family Rikenellaceae (Bacteroidetes) in Aref (fed with cattle and poultry manure), where its relative abundance increased from 5%–6% to 42%–64% (phases 1–2 of Aref, Supplementary Figure S2). In opposite, a decrease was seen in the relative abundance of Caldicoprobacteraceae and uncultivated members of the order Sphingobacteriales (ST-12K33) (Supplementary Figure S3). In Bref, Caldicoprobacteraceae as well as Cloacimonadaceae drastically decreased in association with change from large- to laboratory-scale digester conditions. Instead, members belonging to the family Erysipelotrichaceae, uncultured taxa of Bacteroidales UCG-001, and family XI increased in relative abundance.
3.6.2 Temperature Change and Response in the Bacterial Community
In Atemp and Btemp reactors, the microbial community structures initially resembled the respective reference reactor. As temperature increased above 45°C, the as-yet uncultivated Clostridia MBA03 (Firmicutes) distinctively increased in Atemp (from 5% to 69%–86%). The inoculum used for Btemp consisted of about 30% MBA03, and this group thereafter increased to almost 80% after temperature increase in Btemp. Searches of MBA03-related sequences from the present digester in the NCBI database using BLAST showed low identity (<91%) to previously characterized species. MBA03 was first reported in a thermophilic process treating municipal solid waste (Tang et al., 2004). MBA03 has thereafter been detected in various thermophilic AD, fed for example with cattle manure and steam-exploded wheat straw (Sun et al., 2015), swine manure (Wu et al., 2020), municipal solid waste (Nazina et al., 2018), or food waste under high solid conditions (Westerholm et al., 2020). MBA03 has also been shown to positively respond to trace element addition in mono-digestion of grass silage (FitzGerald et al., 2019). The presence of species belonging to MBA03 in the present digesters indicates a tolerance to high thermophilic temperature conditions and high levels of ammonia (>3 g NH4+-N/L; >1 g NH3-N/L). DTU14 was another group found in both thermophilic digesters, which has previously been detected in thermophilic AD fed with cattle manure (Campanaro et al., 2016). As similar to the abovementioned uncultivated taxa, the functional role of the clostridial DTU14 in biogas digesters is yet to be determined.
In the ordination plot, the microbial tight clustering of Atemp and Btemp during operation at thermophilic temperature demonstrated a strong influence of the temperature on microbial community structure (Supplementary Figure S4), which is consistent with previous findings (De Vrieze et al., 2015; Westerholm et al., 2016b; Fontana et al., 2016; Stolze et al., 2016; Westerholm et al., 2018). There were also stronger similarities in thermophilic microbial communities than in mesophilic communities after the temperature changes (Supplementary Figure S4), indicating that temperature has a stronger influence diminishing the impact of substrate composition and OLR.
3.6.3 Organic Loading Rate and Response in the Bacterial Community
The increase in OLR had a neglectable effect on the microbial community structure in Aref and Bref, with the exception that the relative abundance of candidate W27 (Cloacimonetes) was enhanced in both reactors. The functional role of candidate W27 in anaerobic digestion processes is not yet established, but its presence has been linked to degradation of saturated long-chain fatty acids in a previous study (Yekta et al., 2019b). Overall, the most striking difference between Aref and Bref was the higher prevalence of Rikenellaceae in the former (both before and after the OLR increase). This indicates linkage of its members to the feeding of poultry manure or slightly lower temperature in Aref (38°C) than in Bref (42°C). In AD, members of Rikenellaceae have previously been shown highly abundant in different manure-based biogas processes operating at both mesophilic and thermophilic temperatures (Campanaro et al., 2016; Koo et al., 2019; Ning et al., 2019; Vendruscolo et al., 2020). Moreover, in line with the result of the present study, Rikenellaceae showed a significant increase in relative abundance in response to increased OLR during biogas production from pig manure and corn straw at mesophilic conditions (Ning et al., 2019). Rikenellaceae included acidogenic bacteria involved in degradation of carbohydrates (Graf, 2004), and their increased relative abundance in A compared to B reactors might be linked to the comparably more efficient degradation of hemicellulose and cellulose in the former.
3.6.4 Quantification of Methanogens
Quantification of methanogens by qPCR illustrated dominance of Methanosarcinaceae and Methanobacteriales and lower levels of Methanomicrobiales in both the A and B processes. This result is in accordance with previous studies of manure-based biogas systems (Sun et al., 2015). An increase of temperature and OLR separated the two investigated processes in regard to performance, likely caused by different levels of free ammonia. Even though accumulations of VFA in ATemp suggested reduced methanogenic function, results indicated no major changes in the abundance of any of the methanogenic groups, all showing stable levels during both the temperature and increase in OLR (Supplementary Figure S4). This result was a bit surprising as both temperature and ammonia levels in several studies have been suggested to be strong drivers for the development of the methanogenic community (Guo et al., 2014; Moestedt et al., 2014; Westerholm et al., 2018). High levels of ammonia is known to cause the development of methanogenesis via syntrophic acetate oxidation (SAO), due to selective inhibition of the aceticlastic methanogens. SAO is also commonly detected in thermophilic processes, likely as higher temperature increases the proportion of ammonia (Westerholm et al., 2016a). SAO is a two-step process, in which syntrophic acetate-oxidizing bacteria convert acetate to hydrogen and carbon dioxide, further used by hydrogenotrophic methanogens for methane production. This shift in pathways for methane formation from acetate typically induces increased abundance of hydrogenotrophic methanogens, with Methanomicrobiales and Methanobacteriales often dominating in mesophilic and thermophilic systems, respectively (Westerholm et al., 2016a). Such shift in methanogenic abundance could, however, not be observed in present study, and the abundance of the strictly hydrogenotrophic Methanobacteriales and Methanomicrobiales was stable over time. However, Methanosarcinaceae is able to use both the hydrogenotrophic and the aceticlastic pathways for methanogenesis and has been suggested not only to catalyze aceticlastic methanogenesis under high ammonia stress but also to perform the entire process, i.e., both acetate oxidation and subsequent methanogenesis (Westerholm et al., 2016a). Consequently, this methanogen can be engaged in the conversion of both acetate and hydrogen in the here-investigated processes. The constant and high levels of Methanosarcina in all reactors throughout the operating trial are also in accordance with the suggestion that these methanogens are robust and can manage various stress factors, such as high ammonia levels, temperature fluctuations, and increasing loads (De Vrieze et al., 2012). The minor effect on the abundance of the investigated methanogenic orders and families in response to increasing OLR was in accordance to previous observations made during increased OLR at both mesophilic and thermophilic conditions (Westerholm et al., 2018). However, others have showed increased levels of Methanosarcina and decreased levels of Methanosaeta (Moestedt et al., 2014; Regueiro et al., 2015), a shift in dominance from Methanobacterium to Methanosaeta, or increased levels of Methanospirillum and Methanoculleus and decreased levels of Methanosarcina in response to increased OLR (Lerm et al., 2012; Xiao et al., 2017). Consequently, the impact on methanogenic abundances by increased OLR and decreased HRT can differ considerably and is most likely depending on variations in substrate composition, the prevailing microbial community, temperature, and the extent and rate of change in OLR and HRT. However, it should be kept in mind that the methanogenic communities in the present study were evaluated at order and family levels and that changes could have had occurred at lower taxonomic rank.
4 Conclusion
Farmers want to achieve high biogas yield and high nutrient levels in the digestate when using manure as the main substrate for biogas production. Ways to reach these goals include, for example, high organic load and thermophilic operating temperature. An important factor to consider during optimization of the digestion process is the risk of increased residual methane potential from the digestate, potentially resulting in emissions of methane during storage. This study showed that changing the operating temperature from mesophilic to thermophilic conditions was possible for both investigated processes. The microbial analyses illustrated that both processes adapted to the changing temperature, with similar changes in the taxonomic profile. Still, difference could be seen on process performance, depedning on the substrate mix. With cow manure, the increase in temperature did not change the process performance, but for the process operating with a mix of cattle and poultry manure, a decrease in methane production with increasing temperature was seen, probably caused by a process inhibition by ammonia and accumulation of VFA. As a result, this process also showed a comparably higher residual methane potential. Increasing the organic load and decreasing the hydraulic retention time increased total methane production, with the most pronounced effect at thermophilic temperatures. However, as a consequence of the higher load, the degree of degradation and specific methane production decreased. Moreover, the decrease in methane yield correlated with increased residual methane potential. Thus, high organic load in combination with decreasing retention time, even at thermophilic temperature, risks reducing the degradation efficiency of manure and therefore resulting in increased residual methane production and subsequent emissions from digestate. Consequently, during optimization, it is important to take measures to secure not only methane yields but also overall degradation in order to limit methane emissions from stored digestate. Furthermore, to avoid negative effects caused by high input of manure, it would be good if the regulations of the subsidy in Sweden were set not only to encourage high loads but to also require an analysis of the overall degradation efficiency and/or RMP.
Data Availability Statement
The dataset presented in this study can be found in online repositories. The raw data generated by Illumina Miseq have been submitted to NCBI SRA with the study acession number PRJNA638826.
Author Contributions
AS conceptualized and supervised the study, contributed resources, acquired funding, and reviewed and edited the manuscript. KA performed the investigation, acquired funding, conceptualized the study, wrote the original draft, and performed visualization and formal analyses. MW performed the investigation and reviewed and edited the manuscript. SI performed the investigation. All authors contributed to the article and approved the submitted version.
Funding
The study was funded by the Swedish Rural Economy and Agricultural Society of Sjuhärad and by the Västra Götaland County Council and the project “Stand up for Energy.”
Conflict of Interest
The authors declare that the research was conducted in the absence of any commercial or financial relationships that could be construed as a potential conflict of interest.
Publisher’s Note
All claims expressed in this article are solely those of the authors and do not necessarily represent those of their affiliated organizations, or those of the publisher, the editors and the reviewers. Any product that may be evaluated in this article, or claim that may be made by its manufacturer, is not guaranteed or endorsed by the publisher.
Acknowledgments
The authors gratefully acknowledge Gert Göransson and Lars Paulsson, respective owners of the Högryd and Kvarngården biogas plants. Claudia von Brömssen is acknowledged for statistical advice and the members of the biogas group at the Department of Molecular Sciences for their support and practical work.
Supplementary Material
The Supplementary Material for this article can be found online at: https://www.frontiersin.org/articles/10.3389/fenrg.2021.740314/full#supplementary-material
References
Achinas, S., and Euverink, G. J. W. (2020). Rambling Facets of Manure-Based Biogas Production in Europe: A Briefing. Renew. Sust Energ Rev. 119, 109566. doi:10.1016/j.rser.2019.109566
Adebayo, A. O., Jekayinfa, S. O., and Ahmed, N. A. (2018). Kinetic Study of Thermophilic Anaerobic Digestion of Cattle Manure in a Continuously Stirred Tank Reactor under Varying Organic Loading Rate. ARPN J. Eng. Appl. Sci. 13, 9.
Ahlberg-Eliasson, K. (2018). Swedish Farm-Scale Biogas Production - Substrates and Operating Parameters. Uppsala, Sweden: Swedish University of Agricultural Sciences.
Ahlberg-Eliasson, K., Nadeau, E., Levén, L., and Schnürer, A. (2017). Production Efficiency of Swedish Farm-Scale Biogas Plants. Biomass and Bioenergy 97, 27–37. doi:10.1016/j.biombioe.2016.12.002
Altschul, S. F., Gish, W., Miller, W., Myers, E. W., and Lipman, D. J. (1990). Basic Local Alignment Search Tool. J. Mol. Biol. 215, 403–410. doi:10.1016/s0022-2836(05)80360-2
Amon, B., Kryvoruchko, V., Amon, T., and Zechmeister-Boltenstern, S. (2006). Methane, Nitrous Oxide and Ammonia Emissions during Storage and after Application of Dairy Cattle Slurry and Influence of Slurry Treatment. Agric. Ecosyst. Environ. 112, 153–162. doi:10.1016/j.agee.2005.08.030
Angelidaki, I., Boe, K., and Ellegaard, L. (2005). Effect of Operating Conditions and Reactor Configuration on Efficiency of Full-Scale Biogas Plants. Water Sci. Technol. 52, 189–194. doi:10.2166/wst.2005.0516
APHA (1998). Standard Methods for the Examination of Water and Wastewater. 20th edition. American Public Health Association, American Water Works Association and Water Environment Federation.
Bacenetti, J., Bava, L., Zucali, M., Lovarelli, D., Sandrucci, A., Tamburini, A., et al. (2016). Anaerobic Digestion and Milking Frequency as Mitigation Strategies of the Environmental burden in the Milk Production System. Sci. Total Environ. 539, 450–459. doi:10.1016/j.scitotenv.2015.09.015
Balsberg-Påhlsson, A. (1990). Förbehandling, Uppslutning Och Extraktberedning Av Växt-Och Förnaprov, 10. Lund, Sweden: Lunds University, 18–19.
Baral, K. R., Jégo, G., Amon, B., Bol, R., Chantigny, M. H., Olesen, J. E., et al. (2018). Greenhouse Gas Emissions during Storage of Manure and Digestates: Key Role of Methane for Prediction and Mitigation. Agric. Syst. 166, 26–35. doi:10.1016/j.agsy.2018.07.009
Baudez, J. C., Slatter, P., and Eshtiaghi, N. (2013). The Impact of Temperature on the Rheological Behaviour of Anaerobic Digested Sludge. Chem. Eng. J. 215-216, 182–187. doi:10.1016/j.cej.2012.10.099
Bousková, A., Dohányos, M., Schmidt, J. E., and Angelidaki, I. (2005). Strategies for Changing Temperature from Mesophilic to Thermophilic Conditions in Anaerobic CSTR Reactors Treating Sewage Sludge. Water Res. 39, 1481–1488. doi:10.1016/j.watres.2004.12.042
Burg, V., Bowman, G., Haubensak, M., Baier, U., and Thees, O. (2018). Valorization of an Untapped Resource: Energy and Greenhouse Gas Emissions Benefits of Converting Manure to Biogas through Anaerobic Digestion. Resour. Conservation Recycling 136, 53–62. doi:10.1016/j.resconrec.2018.04.004
Campanaro, S., Treu, L., Kougias, P. G., De Francisci, D., Valle, G., and Angelidaki, I. (2016). Metagenomic Analysis and Functional Characterization of the Biogas Microbiome Using High Throughput Shotgun Sequencing and a Novel Binning Strategy. Biotechnol. Biofuels 9, 26. doi:10.1186/s13068-016-0441-1
Chadwick, D., Sommer, S., Thorman, R., Fangueiro, D., Cardenas, L., Amon, B., et al. (2011). Manure Management: Implications for Greenhouse Gas Emissions. Anim. Feed Sci. Technol. 166-167, 514–531. doi:10.1016/j.anifeedsci.2011.04.036
Chae, K. J., Jang, A., Yim, S. K., and Kim, I. S. (2008). The Effects of Digestion Temperature and Temperature Shock on the Biogas Yields from the Mesophilic Anaerobic Digestion of Swine Manure. Bioresour. Technol. 99, 1–6. doi:10.1016/j.biortech.2006.11.063
Chai, W., and Udén, P. (1998). An Alternative Oven Method Combined with Different Detergent Strengths in the Analysis of Neutral Detergent Fibre. Anim. Feed Sci. Technol. 74, 281–288. doi:10.1016/s0377-8401(98)00187-4
Chen, Y., Cheng, J. J., and Creamer, K. S. (2008). Inhibition of Anaerobic Digestion Process: A Review. Bioresour. Technol. 99, 4044–4064. doi:10.1016/j.biortech.2007.01.057
Choong, Y. Y., Norli, I., Abdullah, A. Z., and Yhaya, M. F. (2016). Impacts of Trace Element Supplementation on the Performance of Anaerobic Digestion Process: A Critical Review. Bioresour. Technol. 209, 369–379. doi:10.1016/j.biortech.2016.03.028
Córdoba, V., Fernández, M., and Santalla, E. (2016). The Effect of Different Inoculums on Anaerobic Digestion of Swine Wastewater. J. Environ. Chem. Eng. 4, 115–122. doi:10.1016/j.jece.2015.11.003
Danielsson, R., Dicksved, J., Sun, L., Gonda, H., Müller, B., Schnürer, A., et al. (2017). Methane Production in Dairy Cows Correlates with Rumen Methanogenic and Bacterial Community Structure. Front. Microbiol. 8, 226. doi:10.3389/fmicb.2017.00226
De Vrieze, J., Hennebel, T., Boon, N., and Verstraete, W. (2012). Methanosarcina: The Rediscovered Methanogen for Heavy Duty Biomethanation. Bioresour. Technol. 112, 1–9. doi:10.1016/j.biortech.2012.02.079
De Vrieze, J., Saunders, A. M., He, Y., Fang, J., Nielsen, P. H., Verstraete, W., et al. (2015). Ammonia and Temperature Determine Potential Clustering in the Anaerobic Digestion Microbiome. Water Res. 75, 312–323. doi:10.1016/j.watres.2015.02.025
Elsgaard, L., Olsen, A. B., and Petersen, S. O. (2016). Temperature Response of Methane Production in Liquid Manures and Co-digestates. Sci. Total Environ. 539, 78–84. doi:10.1016/j.scitotenv.2015.07.145
FitzGerald, J. A., Wall, D. M., Jackson, S. A., Murphy, J. D., and Dobson, A. D. W. (2019). Trace Element Supplementation Is Associated with Increases in Fermenting Bacteria in Biogas Mono-Digestion of Grass Silage. Renew. Energ. 138, 980–986. doi:10.1016/j.renene.2019.02.051
Fontana, A., Patrone, V., Puglisi, E., Morelli, L., Bassi, D., Garuti, M., et al. (2016). Effects of Geographic Area, Feedstock, Temperature, and Operating Time on Microbial Communities of Six Full-Scale Biogas Plants. Bioresour. Technol. 218, 980–990. doi:10.1016/j.biortech.2016.07.058
Fuchs, W., Wang, X., Gabauer, W., Ortner, M., and Li, Z. (2018). Tackling Ammonia Inhibition for Efficient Biogas Production from Chicken Manure: Status and Technical Trends in Europe and China. Renew. Sust. Energ. Rev. 97, 186–199. doi:10.1016/j.rser.2018.08.038
Guo, X., Wang, C., Sun, F., Zhu, W., and Wu, W. (2014). A Comparison of Microbial Characteristics between the Thermophilic and Mesophilic Anaerobic Digesters Exposed to Elevated Food Waste Loadings. Bioresour. Technol. 152, 420–428. doi:10.1016/j.biortech.2013.11.012
Hansen, K. H., Angelidaki, I., and Ahring, B. K. (1998). Anaerobic Digestion of Swine Manure: Inhibition by Ammonia. Water Res. 32, 5–12. doi:10.1016/s0043-1354(97)00201-7
Holliger, C., Alves, M., Andrade, D., Angelidaki, I., Astals, S., Baier, U., et al. (2016). Towards a Standardization of Biomethane Potential Tests. Water Sci. Technol. 74, 2515–2522. doi:10.2166/wst.2016.336
Holm-Nielsen, J. B., Al Seadi, T., and Oleskowicz-Popiel, P. (2009). The Future of Anaerobic Digestion and Biogas Utilization. Bioresour. Technol. 100, 5478–5484. doi:10.1016/j.biortech.2008.12.046
Hou, Y., Velthof, G. L., Lesschen, J. P., Staritsky, I. G., and Oenema, O. (2017). Nutrient Recovery and Emissions of Ammonia, Nitrous Oxide, and Methane from Animal Manure in Europe: Effects of Manure Treatment Technologies. Environ. Sci. Technol. 51, 375–383. doi:10.1021/acs.est.6b04524
Huang, J. J. H., and Shih, J. C. H. (1981). The Potential of Biological Methane Generation from Chicken Manure. Biotechnol. Bioeng. 23, 2307–2314. doi:10.1002/bit.260231013
Hupfauf, S., Winkler, A., Wagner, A. O., Podmirseg, S. M., and Insam, H. (2020). Biomethanation at 45 °C Offers High Process Efficiency and Supports Hygienisation. Bioresour. Technol. 300, 122671. doi:10.1016/j.biortech.2019.122671
Insam, H., Gómez-Brandón, M., and Ascher, J. (2015). Manure-based Biogas Fermentation Residues - Friend or Foe of Soil Fertility? Soil Biol. Biochem. 84, 1–14. doi:10.1016/j.soilbio.2015.02.006
Koo, T., Yulisa, A., and Hwang, S. (2019). Microbial Community Structure in Full Scale Anaerobic Mono-And Co-digesters Treating Food Waste and Animal Waste. Bioresour. Technol. 282, 439–446. doi:10.1016/j.biortech.2019.03.050
Labatut, R. A., Angenent, L. T., and Scott, N. R. (2011). Biochemical Methane Potential and Biodegradability of Complex Organic Substrates. Bioresour. Technol. 102, 2255–2264. doi:10.1016/j.biortech.2010.10.035
Labatut, R. A., Angenent, L. T., and Scott, N. R. (2014). Conventional Mesophilic vs. Thermophilic Anaerobic Digestion: A Trade-Off between Performance and Stability? Water Res. 53, 249–258. doi:10.1016/j.watres.2014.01.035
Larsson, K., and Bengtsson, S. (1983). Determination of Nonstructural Carbohydrates in Plant Material, Method Description No. 22. Uppsala: National Laboratory of Agricultural Chemistry. Sweden 8.
Lebuhn, M., Munk, B., and Effenberger, M. (2014). Agricultural Biogas Production in Germany - from Practice to Microbiology Basics. Energ Sustain. Soc. 4, 10. doi:10.1186/2192-0567-4-10
Lehtomaki, A. (2006) Biogas Production from Energy Crops and Crops Residues: University of Juväskylä 2006. Report No: 163.
Lerm, S., Kleyböcker, A., Miethling-Graff, R., Alawi, M., Kasina, M., Liebrich, M., et al. (2012). Archaeal Community Composition Affects the Function of Anaerobic Co-digesters in Response to Organic Overload. Waste Manage. 32, 389–399. doi:10.1016/j.wasman.2011.11.013
Li, D., Liu, S., Mi, L., Li, Z., Yuan, Y., Yan, Z., et al. (2015). Effects of Feedstock Ratio and Organic Loading Rate on the Anaerobic Mesophilic Co-digestion of rice Straw and Pig Manure. Bioresour. Technol. 187, 120–127. doi:10.1016/j.biortech.2015.03.040
Lindorfer, H., Corcoba, A., Vasilieva, V., Braun, R., and Kirchmayr, R. (2008a). Doubling the Organic Loading Rate in the Co-digestion of Energy Crops and Manure - A Full Scale Case Study. Bioresour. Technol. 99, 1148–1156. doi:10.1016/j.biortech.2007.02.033
Lindorfer, H., Waltenberger, R., Köllner, K., Braun, R., and Kirchmayr, R. (2008b). New Data on Temperature Optimum and Temperature Changes in Energy Crop Digesters. Bioresour. Technol. 99, 7011–7019. doi:10.1016/j.biortech.2008.01.034
Liu, T., Sun, L., Müller, B., and Schnürer, A. (2017). Importance of Inoculum Source and Initial Community Structure for Biogas Production from Agricultural Substrates. Bioresour. Technol. 245, 768–777. doi:10.1016/j.biortech.2017.08.213
Ma, G., Ndegwa, P., Harrison, J. H., and Chen, Y. (2020). Methane Yields during Anaerobic Co-digestion of Animal Manure with Other Feedstocks: A Meta-Analysis. Sci. Total Environ. 728, 138224. doi:10.1016/j.scitotenv.2020.138224
Mannheim, B. (1984). Methods of Enzymatic Food Analysis Using Test-Combinations. Boehringer Mannheim GmbH 9, 27–29.
Marchaim, U., and Krause, C. (1993). Propionic to Acetic Acid Ratios in Overloaded Anaerobic Digestion. Bioresour. Technol. 43, 195–203. doi:10.1016/0960-8524(93)90031-6
Mata-Alvarez, J., Dosta, J., Romero-Güiza, M. S., Fonoll, X., Peces, M., and Astals, S. (2014). A Critical Review on Anaerobic Co-digestion Achievements between 2010 and 2013. Renew. Sust. Energ. Rev. 36, 412–427. doi:10.1016/j.rser.2014.04.039
Moestedt, J., Nilsson Påledal, S., and Schnürer, A. (2013). The Effect of Substrate and Operational Parameters on the Abundance of Sulphate-Reducing Bacteria in Industrial Anaerobic Biogas Digesters. Bioresour. Technol. 132, 327–332. doi:10.1016/j.biortech.2013.01.043
Moestedt, J., Nordell, E., and Schnürer, A. (2014). Comparison of Operating Strategies for Increased Biogas Production from Thin Stillage. J. Biotechnol. 175, 22–30. doi:10.1016/j.jbiotec.2014.01.030
Moller, H. B., Sommer, S. G., and Ahring, B. (2004). Methane Productivity of Manure, Straw and Solid Fractions of Manure. Biomass Bioenerg. 26, 485–495.
Monalu, F., Sambusiti, C., Ficara, E., Aboulkas, A., Barakat, A., and Carrere, H. (2015). New Opportunities for Agricultural Digestate Valorization - Current Situation and Perspectives. Energ Env Sci. 8, 2600–2621.
Monlau, F., Sambusiti, C., Ficara, E., Aboulkas, A., Barakat, A., and Carrère, H. (2015). New Opportunities for Agricultural Digestate Valorization: Current Situation and Perspectives. Energy Environ. Sci. 8, 2600–2621. doi:10.1039/c5ee01633a
Moset, V., Poulsen, M., Wahid, R., Højberg, O., and Møller, H. B. (2015). Mesophilic versus Thermophilic Anaerobic Digestion of Cattle Manure: Methane Productivity and Microbial Ecology. Microb. Biotechnol. 8, 787–800. doi:10.1111/1751-7915.12271
Mulat, D. G., Jacobi, H. F., Feilberg, A., Adamsen, A. P. S., Richnow, H., and Nikolausz, M. (2015). Changing Feeding Regimes to Demonstrate Flexible Biogas Production: Effects on Process Performance, Microbial Community Structure and Methanogenic Pathways. Appl. Environ. Microbiol.
Nazina, T. N., Sokolova, D. S., Babich, T. L., Semenova, E. M., Borzenkov, I. A., Bidzhieva, S. K., et al. (2018). Phylogenetic Diversity of Microorganisms from the Sludge of a Biogas Reactor Processing Oil-Containing and Municipal Waste. Microbiology 87, 416–424. doi:10.1134/s0026261718030074
Niemi Hjulfors, L., and Edström, F. Utvärdering Av Gödselgasstödet 2015-2016. Available at: http://www2.jordbruksverket.se/download/18.3a9fff8015d0a58556c7572f/1499259064453/ra17_11.pdf. Sweden: Swedish Board of Agriculture2017. Report No: 2017:11.
Ning, J., Zhou, M., Pan, X., Li, C., Lv, N., Wang, T., et al. (2019). Simultaneous Biogas and Biogas Slurry Production from Co-digestion of Pig Manure and Corn Straw: Performance Optimization and Microbial Community Shift. Bioresour. Technol. 282, 37–47. doi:10.1016/j.biortech.2019.02.122
Nolan, S., Waters, N. R., Brennan, F., Auer, A., Fenton, O., Richards, K., et al. (2018). Toward Assessing Farm-Based Anaerobic Digestate Public Health Risks: Comparative Investigation with Slurry, Effect of Pasteurization Treatments, and Use of Miniature Bioreactors as Proxies for Pathogen Spiking Trials. Front. Sust Food Syst. 2. doi:10.3389/fsufs.2018.00041
Ozbayram, E. G., Akyol, Ç., Ince, B., Karakoç, C., and Ince, O. (2018). Rumen Bacteria at Work: Bioaugmentation Strategies to Enhance Biogas Production from Cow Manure. J. Appl. Microbiol. 124, 491–502. doi:10.1111/jam.13668
Persson, T., Månsson, L., and Bohn, I. (2017). Vattenverksslam Reducerar Biogasens Svavelväte - Bra För Miljön Och Ekonomin. Energiforsk AB, 344. 2017.
Pratt, C., Redding, M., Hill, J., Mudge, S. R., Westermann, M., Paungfoo-Lonhienne, C., et al. (2014). Assessing Refrigerating and Freezing Effects on the Biological/chemical Composition of Two Livestock Manures. Agric. Ecosyst. Environ. 197, 288–292. doi:10.1016/j.agee.2014.08.012
Rajagopal, R., Massé, D. I., and Singh, G. (2013). A Critical Review on Inhibition of Anaerobic Digestion Process by Excess Ammonia. Bioresour. Technol. 143, 632–641. doi:10.1016/j.biortech.2013.06.030
Raposo, F., Borja, R., Martín, M. A., Martín, A., de la Rubia, M. A., and Rincón, B. (2009). Influence of Inoculum-Substrate Ratio on the Anaerobic Digestion of sunflower Oil Cake in Batch Mode: Process Stability and Kinetic Evaluation. Chem. Eng. J. 149, 70–77. doi:10.1016/j.cej.2008.10.001
Rasi, S., Läntelä, J., and Rintala, J. (2011). Trace Compounds Affecting Biogas Energy Utilisation - A Review. Energ. Convers. Manage. 52, 3369–3375. doi:10.1016/j.enconman.2011.07.005
Regueiro, L., Lema, J. M., and Carballa, M. (2015). Key Microbial Communities Steering the Functioning of Anaerobic Digesters during Hydraulic and Organic Overloading Shocks. Bioresour. Technol. 197, 208–216. doi:10.1016/j.biortech.2015.08.076
Rhode, L., Baky, A., Olsson, J., and Nordberg, Å (2012). Växthusgaser Från Stallgödsel - Littertur Genomgång Och Modellberäkningar. Agric. Industry 402, 14.
Rico, C., Muñoz, N., and Rico, J. L. (2015). Anaerobic Co-digestion of Cheese Whey and the Screened Liquid Fraction of Dairy Manure in a Single Continuously Stirred Tank Reactor Process: Limits in Co-substrate Ratios and Organic Loading Rate. Bioresour. Technol. 189, 327–333. doi:10.1016/j.biortech.2015.04.032
Risberg, K., Sun, L., Levén, L., Horn, S. J., and Schnürer, A. (2013). Biogas Production from Wheat Straw and Manure - Impact of Pretreatment and Process Operating Parameters. Bioresour. Technol. 149, 232–237. doi:10.1016/j.biortech.2013.09.054
Ruile, S., Schmitz, S., Mönch-Tegeder, M., and Oechsner, H. (2015). Degradation Efficiency of Agricultural Biogas Plants - A Full-Scale Study. Bioresour. Technol. 178, 341–349. doi:10.1016/j.biortech.2014.10.053
Schnürer, A., Bohn, I., and Moestedt, J. (2017). “Protocol for Start-Up and Operation of CSTR Biogas Processes,” in Hydrocarbon and Lipid Microbiology Protocols. Editor M. G. TJea (Springer Protocols Handbooks), 171–200.
Shakeri Yekta, S., Liu, T., Axelsson Bjerg, M., Šafarič, L., Karlsson, A., Björn, A., et al. (2019b). Sulfide Level in Municipal Sludge Digesters Affects Microbial Community Response to Long-Chain Fatty Acid Loads. Biotechnol. Biofuels 12, 259. doi:10.1186/s13068-019-1598-1
Shakeri Yekta, S., Hedenström, M., Svensson, B. H., Sundgren, I., Dario, M., Enrich-Prast, A., et al. (2019a). Molecular Characterization of Particulate Organic Matter in Full Scale Anaerobic Digesters: An NMR Spectroscopy Study. Sci. Total Environ. 685, 1107–1115. doi:10.1016/j.scitotenv.2019.06.264
Sondergaard, M. M., Fotidis, I. A., Kovalovszki, A., and Angelidaki, I. (2015). Anaerobic Co-digestion of Agricultural Byproducts with Manure for Enhanced Biogas Production. Energ Fuel 29, 8088–8094.
SOU2019:63 (2019). Mer Biogas! För Ett Hållbart Sverige (2019) Govermental Biogas Market Investigation. 978-91-38-25002-0.
Stolze, Y., Bremges, A., Rumming, M., Henke, C., Maus, I., Pühler, A., et al. (2016). Identification and Genome Reconstruction of Abundant Distinct Taxa in Microbiomes from One Thermophilic and Three Mesophilic Production-Scale Biogas Plants. Biotechnol. Biofuels 9, 156. doi:10.1186/s13068-016-0565-3
Sun, L., Liu, T., Müller, B., and Schnürer, A. (2016). The Microbial Community Structure in Industrial Biogas Plants Influences the Degradation Rate of Straw and Cellulose in Batch Tests. Biotechnol. Biofuels 9, 128. doi:10.1186/s13068-016-0543-9
Sun, L., Pope, P. B., Eijsink, V. G. H., and Schnürer, A. (2015). Characterization of Microbial Community Structure during Continuous Anaerobic Digestion of Straw and Cow Manure. Microb. Biotechnol. 8, 815–827. doi:10.1111/1751-7915.12298
Swedish Board of Agriculture (2015). Statens Jordbruksverks Föreskrifter Om Statligt Stöd till Produktion Av Biogas Från Gödsel SJVFS 2015:10. Available from: Swedish Board of Agriculture https://djur.jordbruksverket.se/download/18.2a80628d14ccceff204dc56d/1429509937376/2015-010.pdf.
Swedish Board of Agriculture (2013). Utformning Av Pilotprojekt Avseende Ersättning För Dubbel Miljönytta 2014-2023 (Gödselgasstöd). Available from: Swedish Board of Agriculture https://djur.jordbruksverket.se/download/18.465e4964142dbfe4470395e/1387528575113/Förslag%20till%20gödselgasstöd_131220.pdf.
Swedish Energy Agency (2020). Produktion Och Användning Av Biogas Och Rötrester År 2019 (2019b). Rep. ER 25.978-91-89184-75-6
Swedish Government (2010). The Swedish National Action Plan for the Promotion of the Use of Renewable Energy in Accordance with Directive 2009/28/EC and the Comission Decision of 30.06, 2009.
Tang, Y., Shigematsu, T., Ikbal Morimura, S., and Kida, K. (2004). The Effects of Micro-aeration on the Phylogenetic Diversity of Microorganisms in a Thermophilic Anaerobic Municipal Solid-Waste Digester. Water Res. 38, 2537–2550. doi:10.1016/j.watres.2004.03.012
Team, R. (2016). RStudio. Boston, MA: Integrated Development for R. RStudio, Inc. Available at: http://www.rstudio.com/. 2016.
Thygesen, O., Sommer, S. G., Shin, S. G., and Triolo, J. M. (2014). Residual Biochemical Methane Potential (BMP) of Concentrated Digestate from Full-Scale Biogas Plants. Fuel 132, 44–46. doi:10.1016/j.fuel.2014.04.062
Tian, Z., Zhang, Y., Li, Y., Chi, Y., and Yang, M. (2015). Rapid Establishment of Thermophilic Anaerobic Microbial Community during the One-step Startup of Thermophilic Anaerobic Digestion from a Mesophilic Digester. Water Res. 69, 9–19. doi:10.1016/j.watres.2014.11.001
Triolo, J. M., Sommer, S. G., Møller, H. B., Weisbjerg, M. R., and Jiang, X. Y. (2011). A New Algorithm to Characterize Biodegradability of Biomass during Anaerobic Digestion: Influence of Lignin Concentration on Methane Production Potential. Bioresour. Technol. 102, 9395–9402. doi:10.1016/j.biortech.2011.07.026
Van Soest, P. J., Robertson, J. B., and Lewis, B. A. (1991). Methods for Dietary Fiber, Neutral Detergent Fiber, and Nonstarch Polysaccharides in Relation to Animal Nutrition. J. Dairy Sci. 74, 3583–3597. doi:10.3168/jds.s0022-0302(91)78551-2
Vendruscolo, E. C. G., Mesa, D., Rissi, D. V., Meyer, B. H., de Oliveira Pedrosa, F., de Souza, E. M., et al. (2020). Microbial Communities Network Analysis of Anaerobic Reactors Fed with Bovine and Swine Slurry. Sci. Total Environ. 742, 140314. doi:10.1016/j.scitotenv.2020.140314
Ward, A. J., Hobbs, P. J., Holliman, P. J., and Jones, D. L. (2008). Optimisation of the Anaerobic Digestion of Agricultural Resources. Bioresour. Technol. 99, 7928–7940. doi:10.1016/j.biortech.2008.02.044
Watcharasukarn, M., Kaparaju, P., Steyer, J.-P., Krogfelt, K. A., and Angelidaki, I. (2009). Screening Escherichia coli, Enterococcus faecalis, and Clostridium perfringens as Indicator Organisms in Evaluating Pathogen-Reducing Capacity in Biogas Plants. Microb. Ecol. 58, 221–230. doi:10.1007/s00248-009-9497-9
Weiland, P. (2010). Biogas Production: Current State and Perspectives. Appl. Microbiol. Biotechnol. 85, 849–860. doi:10.1007/s00253-009-2246-7
Westerholm, M., Liu, T., and Schnürer, A. (2020). Comparative Study of Industrial-Scale High-Solid Biogas Production from Food Waste: Process Operation and Microbiology. Bioresour. Technol. 304, 122981. doi:10.1016/j.biortech.2020.122981
Westerholm, M., Castillo, M. d. P., Chan Andersson, A., Jahre Nilsen, P., and Schnürer, A. (2019). Effects of thermal Hydrolytic Pre-treatment on Biogas Process Efficiency and Microbial Community Structure in Industrial- and Laboratory-Scale Digesters. Waste Manage. 95, 150–160. doi:10.1016/j.wasman.2019.06.004
Westerholm, M., Hansson, M., and Schnürer, A. (2012a). Improved Biogas Production from Whole Stillage by Co-digestion with Cattle Manure. Bioresour. Technol. 114, 314–319. doi:10.1016/j.biortech.2012.03.005
Westerholm, M., Isaksson, S., Karlsson Lindsjö, O., and Schnürer, A. (2018). Microbial Community Adaptability to Altered Temperature Conditions Determines the Potential for Process Optimisation in Biogas Production. Appl. Energ. 226, 838–848. doi:10.1016/j.apenergy.2018.06.045
Westerholm, M., Levén, L., and Schnürer, A. (2012b). Bioaugmentation of Syntrophic Acetate-Oxidizing Culture in Biogas Reactors Exposed to Increasing Levels of Ammonia. Appl. Environ. Microbiol. 78, 7619–7625. doi:10.1128/aem.01637-12
Westerholm, M., Moestedt, J., and Schnürer, A. (2016a). Biogas Production through Syntrophic Acetate Oxidation and Deliberate Operating Strategies for Improved Digester Performance. Appl. Energ. 179, 124–135. doi:10.1016/j.apenergy.2016.06.061
Westerholm, M., Moestedt, J., and Schnürer, A. (2016b). Biogas Production through Syntrophic Acetate Oxidation and Deliberate Operating Strategies for Improved Digester Performance. Appl. Energ. 179, 124–135. doi:10.1016/j.apenergy.2016.06.061
Wu, X., Tian, Z., Lv, Z., Chen, Z., Liu, Y., Yong, X., et al. (2020). Effects of Copper Salts on Performance, Antibiotic Resistance Genes, and Microbial Community during Thermophilic Anaerobic Digestion of Swine Manure. Bioresour. Technol. 300, 122728. doi:10.1016/j.biortech.2019.122728
Xiao, X., Shi, W., Huang, Z., Ruan, W., Miao, H., Ren, H., et al. (2017). Process Stability and Microbial Response of Anaerobic Membrane Bioreactor Treating High-Strength Kitchen Waste Slurry under Different Organic Loading Rates. Int. Biodeterioration Biodegradation 121, 35–43. doi:10.1016/j.ibiod.2017.03.012
Yenigün, O., and Demirel, B. (2013). Ammonia Inhibition in Anaerobic Digestion: A Review. Process Biochem. 48, 901–911. doi:10.1016/j.procbio.2013.04.012
Yu, Y., Lee, C., Kim, J., and Hwang, S. (2005). Group-specific Primer and Probe Sets to Detect Methanogenic Communities Using Quantitative Real-Time Polymerase Chain Reaction. Biotechnol. Bioeng. 89, 670–679. doi:10.1002/bit.20347
Keywords: methane production, residual methane potential, cattle and poultry manure, process optimization, ammonia inhibition, microbial community structure, operational temperature
Citation: Ahlberg-Eliasson K, Westerholm M, Isaksson S and Schnürer A (2021) Anaerobic Digestion of Animal Manure and Influence of Organic Loading Rate and Temperature on Process Performance, Microbiology, and Methane Emission From Digestates. Front. Energy Res. 9:740314. doi: 10.3389/fenrg.2021.740314
Received: 12 July 2021; Accepted: 06 October 2021;
Published: 20 December 2021.
Edited by:
Luís Ferreira, National Innovation Agency, PortugalReviewed by:
Qaisar Mahmood, COMSATS University, PakistanAlawi Sulaiman, MARA University of Technology, Malaysia
Copyright © 2021 Ahlberg-Eliasson, Westerholm, Isaksson and Schnürer. This is an open-access article distributed under the terms of the Creative Commons Attribution License (CC BY). The use, distribution or reproduction in other forums is permitted, provided the original author(s) and the copyright owner(s) are credited and that the original publication in this journal is cited, in accordance with accepted academic practice. No use, distribution or reproduction is permitted which does not comply with these terms.
*Correspondence: Anna Schnürer, YW5uYS5zY2huJiN4MDAwZmM7cmVyQHNsdS5zZQ==