- The Department of Medical Imaging, Guangdong Second Provincial General Hospital, Guangzhou, China
The increasing demands on stretchable power supply for wearable electronics accelerate the development of stretchable batteries. Zn-based batteries are promising to be applied in wearable electronics due to their outstanding performance, intrinsic safety, low cost, and environmental friendliness. Recently, stretchable Zn-based batteries are designed to demonstrate the capability of delivering excellent electrochemical performance, meanwhile maintaining their mechanical stability. This review provides an overview of different strategies and designs to realize stretchability in different Zn-based battery components. The general strategies to realize stretchability are first introduced, followed by the specific designs on the cathode, anode, and electrolytes of Zn batteries. Moreover, current issues and possible strategies are also highlighted.
Introduction
With the rapid development of wearable smart devices, such as wearable displays, health-monitoring devices, and active radio-frequency identification tags, many researchers have carried out in-depth research on stretchable batteries for their use as compatible power supplies (Liang et al., 2017a; Rogers et al., 2019; Lee et al., 2020). As for the stretchable batteries, they are required to continuously output stable electrochemical performance, even under a large level of mechanical deformation (Song et al., 2014; Mackanic et al., 2020b). The performance under stretchability should be reliable, where the as-fabricated device should be capable of enduring mechanical strain and meanwhile maintaining stable functionality. Such stretchability is challenging to achieve in conventionally layer-stacked battery configurations, where multiple component layers are intrinsically made into a grid (Zhang et al., 2015; Song et al., 2019). However, even a slight deformation can cause structural damages to active materials and consequential battery failures (Zhou et al., 2018; Gu et al., 2019). Thus, the first target to realize stretchable batteries is design stretchable frameworks to support the stable functionality of each battery component.
Until now, stretchable batteries based on many different chemistries have been developed, where safety is of paramount importance for human wearable electronics (Gaikwad et al., 2012; Song et al., 2019). Generally, mature battery chemistries have been explored, especially based on aprotic Li-ion batteries, while some corresponding severe safety issues of electrolytes, such as fire-catching and flammable property (Chawla et al., 2019; Du et al., 2020), resulted in safety concerns especially subjected to different mechanical manipulations. As alternative and promising systems, significant developments in aqueous chemistries have been achieved for stretchable batteries, due to the intrinsic safety of aqueous electrolytes (Zhang H. et al., 2021; Liang et al., 2021). The representative Zn-ion batteries are the most promising systems due to the outstanding electrochemical performance of Zn-metal anodes with a lower reaction potential (−0.76 V versus standard hydrogen reaction) and high theoretical gravimetric capacity (820 mA h g−1). Battery reaction chemistries regarding cathode materials are widely studied, such as ion-insertion–type cathodes like MnO2 (Pan et al., 2016; Fang et al., 2019; Zhang T. et al., 2020), V2O5 (Kundu et al., 2016; Xu et al., 2020), conversion-type cathodes (Ag2O) (Kumar et al., 2017; Kumar et al., 2019), and air cathodes (O2) (Stock et al., 2019; Zhang Y. et al., 2021), where some detailed reviews have recently summarized these cathode/anode chemistries of Zn battery (Fang et al., 2018; Zhang W. et al., 2020; Hansen and Liu, 2021). On the contrary, the Zn-anode reactions can be classified into mild/neutral electrolytes and alkaline electrolytes, where reactions can be elaborated as Zn ⇌ Zn2+ + 2e− for the mild/neutral electrolytes and they can be elaborated as Zn + 4OH−⇌ Zn(OH)42− + 2e− for alkaline cases (Zhou et al., 2021). Considering all these Zn-based battery systems can deliver outstanding energy density and power density, combining the structural/configurational designs with the aqueous Zn–based chemistry is necessary to develop safe and stretchable batteries with stable performance.
In this review, we suddenly shift from the reaction chemistry of the battery and first focus on the general designing strategies of different components and integrated devices and then move to discussing the specific designing strategies to enable stretchability of Zn-based battery chemistries. To make battery components stretchable, there are two general designing strategies as material designs and structural designs. According to these two points, the representative examples are subsequently showcased from different components in the Zn-based battery, i.e., electrodes and electrolytes. Finally, we provide commentaries and perspectives on the outstanding challenges that must be overcome to make deformable batteries a reality.
General Material and Structural Designing Strategies for Stretchable Batteries
Material and structural designs are employed to impart robust mechanical durability and conformity of stretchable batteries to nonplanar surfaces (Qian et al., 2019; Chen et al., 2021). Targets at realizing stretchable batteries would add mechanical difficulties, since the stretchability must be the zoomed-in area on a millimeter scale once subjected to different force forms and directions (Mackanic et al., 2020b; Yin et al., 2020). Generally, two methods are applied to enable stretchability as 1) material innovations and 2) structural designs. Specifically, material designs for stretchability focus on synthesizing novel stretchable active materials and/or combining active materials with stretchable hosting materials. On the contrary, structural designing strategies focus on combining specially designed stretchable shapes/structures to host rigid battery components.
Typical battery configurations are composed of four main components, anode, cathode, separator, electrolyte, and current collectors, as shown in Figure 1A. However, all of these components are generally rigid, which cannot preserve their structural integrity once subjected to forces exceeding their plastic deformation limits (Say et al., 2020). When targeted at endowing stretchability to these battery components, they are generally divided into three layers by integrating the cathode materials with their corresponding current collectors, the anode materials with their corresponding current collectors, and the electrolyte with the separator. Finally, stretchable batteries can be integrated and fabricated at the device level (Mackanic et al., 2020a). In other words, there would be three different layers of active materials to build up the stretchable battery devices (Figure 1B). Considering the electrode materials are generally inorganic materials without intrinsic deformability, stretchable polymer networks are generally introduced to endow stretchability, by building up percolating networks composed of conductive nanomaterials, electrochemical active materials, and stretchable supporting polymers, taking the polymeric cathode composite as an example in Figure 1C. Such strategy generally correlates the reengineering to obtain a composite electrode, where the polymeric ingredients are responsible for the mechanical stretchability and other materials are responsible for the conductive/electrochemical performance. A typical example is shown as embedding the silver nanowires into the stretchable polydimethylsiloxane (PDMS) substrate to obtain stretchability (Figure 1D) (Yan et al., 2014). Regarding the combination of the electrolyte and separator, stretchable hydrogel-based polymers are applied with intrinsic stretchability to act as the ion conductors, with more detailed discussions elaborated in the following.
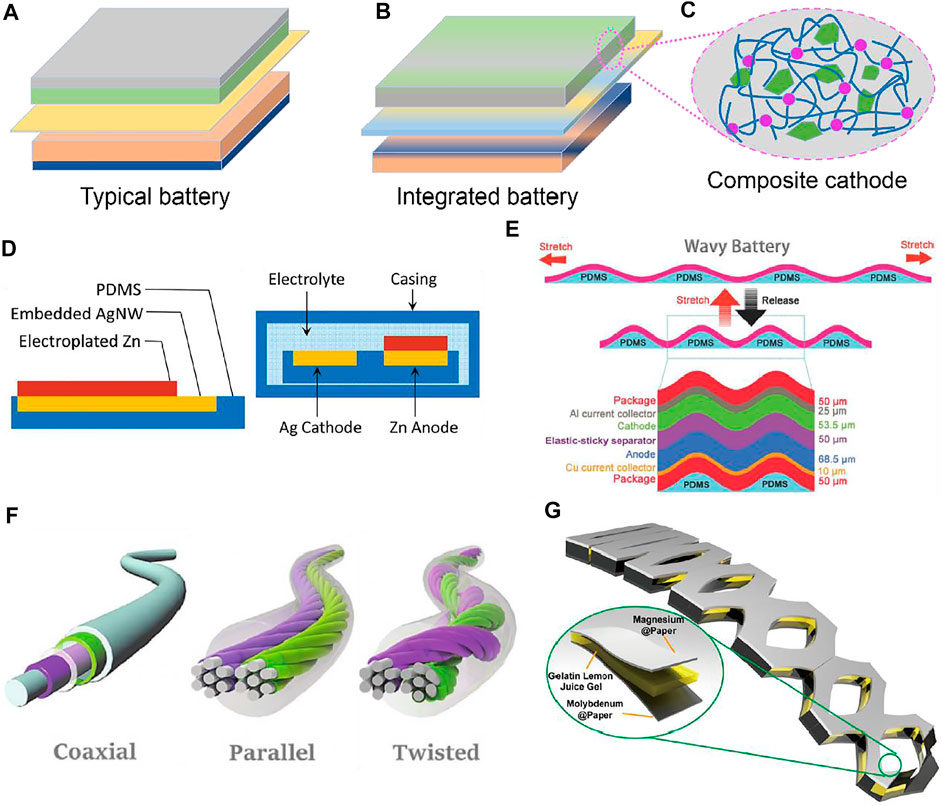
FIGURE 1. Illustration of one cycle of typical battery configurations with 5 stacked layers (A) and integrated battery configurations with 3 stacked layers (B) by integrating the current collector with the electrochemical active materials. (C) Illustrations of the active materials embedded into stretchable polymer networks as a composite electrode. (D) Schematics of battery configurations by embedding the current collector into stretchable PDMS (reproduced from Yan et al. (2014)). (E) The wavy battery structure based on the wavy stretchable PDMS substrate (reproduced from Mackanic et al. (2020a)). (F) Three types of fiber-shaped batteries (reproduced from Mo et al. (2020)). (G) Three types of kirigami-modified batteries (reproduced from Wang et al. (2021)).
Despite endowing stretchability to individual battery components, structural designs are also capable of enabling stretchability of rigid battery components by transforming them into stretchable configurations after adapting wavy (Figure 1E) (Mackanic et al., 2020a), fiber-shaped (Figure 1F) (Mo et al., 2020), or kirigami structures (Figure 1G) (Wang et al., 2021), to redistribute the external forces on the specific component unit. Of note, by applying these promising battery configurations, stretchable batteries could be realized based on the stretchability of supporting elastomers to redistribute force, without strict requirements on the stretchability of the battery components. One representative example is the stretchable batteries built on island-bridge configurations (Yin et al., 2018) with the battery components being rigid and connected by stress-tolerant conductive networks. Not all the workable structural designs schemed in Figure 1, such as the wavy structure, are explored and demonstrated in stretchable Zn-based battery systems, but these structures might be promising to be applied in growing research. Strategies from material and structural designing aspects to realize stretchable Zn-based battery configurations are discussed in the following.
Material Designs for Stretchable Zn-Based Batteries
In general, one representative strategy to obtain intrinsically stretchable battery components is to mix the active material with the precursor of the elastomer and then cure it. First, it is necessary to first obtain a stretchable current collector and the electrochemical active materials can be coated simultaneously with the conductive materials and/or subsequently electrodeposited in situ onto the current collector. For example, Yan et al. (2014) have designed stretchable rechargeable Zn–Ag batteries and then embedded conductive AgNWs into stretchable PDMS. They could simultaneously act as a stretchable composite current collector and cathode materials. In addition, the Zn anode can also be electrodeposited onto the stretchable AgNWs–based electrode to gain stretchability. The as-obtained Ag–Zn battery could endure stretchability up to 80% deformation and deliver a decent output voltage of ∼1.63 V at a current density of 1 mA cm−2 under 80% deformation with an energy density of 0.44 mW h cm−2.
Printing technologies can be applied by one-spot mixing of all the active materials as ink (Guo et al., 2021), including conductive materials, electrochemical active materials, and the elastomer precursors, together (Figure 2A). Wang et al. have used Ag2O and Zn as cathode and anode materials (Kumar et al., 2017), respectively, which can deliver excellent capacity at a rate of 2.5 mA h cm−2 and preserving their structure can maintain structural integrity after being subjected to 100% deformation (Figures 2B–E). Such outstanding structural stability can be assigned to the excellent resiliency of the applied elastomer against severe battery stretching (Kumar et al., 2017; Song et al., 2020). It should be emphasized that in a stretchable device, the structure resiliency is an important performance parameter to evaluate the structural stability of the stretchable device, which should rely on the selected suitable elastomers to support active materials. In addition, Li et al. (2020) have also applied the analogous strategy with screen printing to embed the conductive circuit into the elastomer and conformally deposited the electrochemical active materials onto the stretchable substrate, building the zinc-ion microbattery. Despite embedding the active material into the stretchable substrate, Zhu et al. (2018) have also developed more facile strategy by a dip-coating strategy to design a stretchable substrate that is modified from commercial polyurethane sponge, to host the cathode and anode materials. The bonding force between the conductive AgNWs and the stretchable substrates can be further enhanced by introducing interfacial chemical anchoring (Huang et al., 2019).
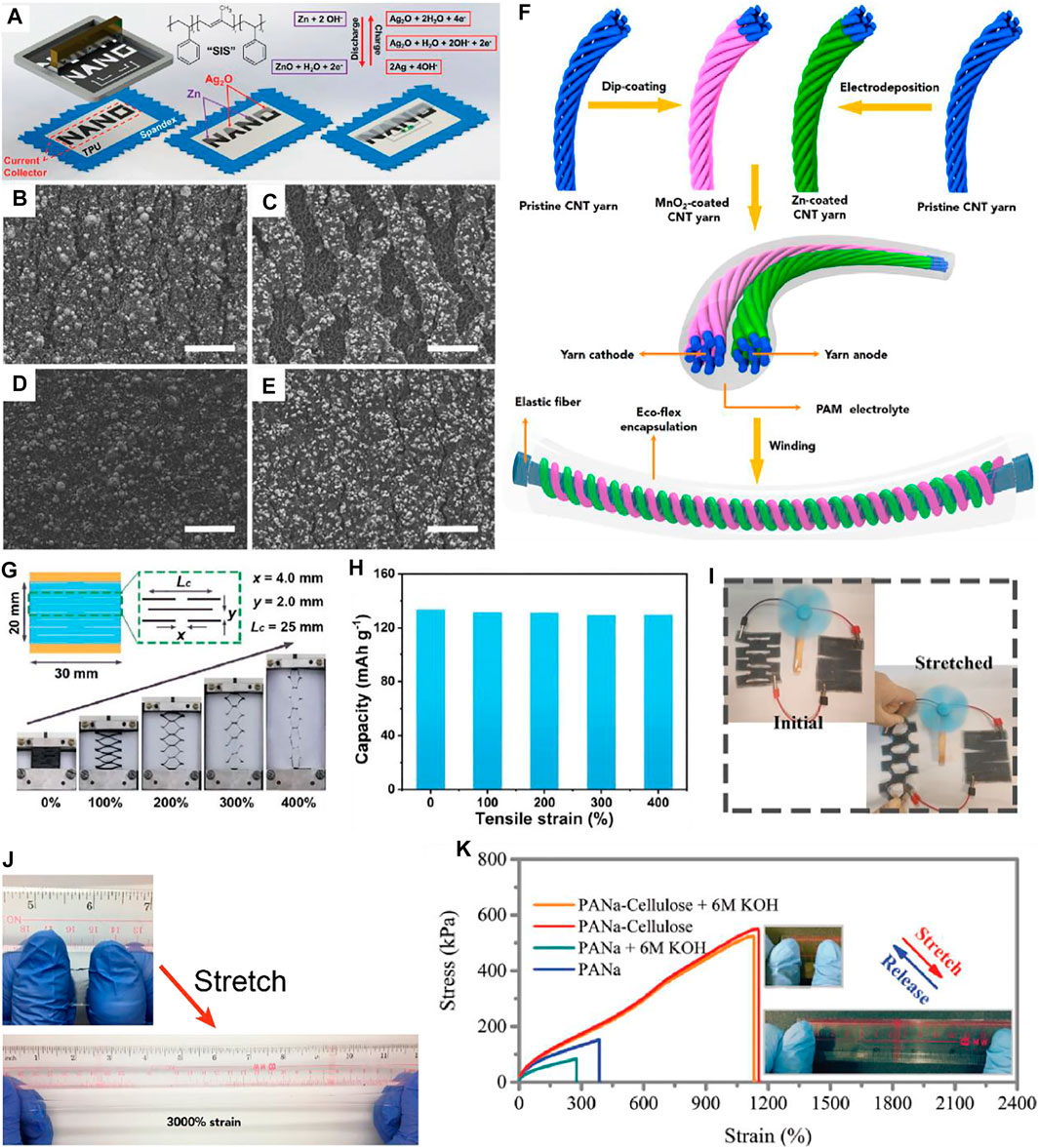
FIGURE 2. (A) Screen-printing steps of a Zn–Ag2O battery on a stretchable textile, exhibiting the cathode and anode reactions. Stretched states of the Zn anode (B) and the Ag2O cathode (C), and recovered states of the Zn anode (D) and the Ag2O cathode (E), respectively; scale bar: 50 µm (reproduced from Kumar et al. (2019)). (F) Schematic diagram of the fabrication process of the fiber-shaped batteries (reproduced from Li et al. (2020)). (G) Kirigami strategies to obtain Zn–polyaniline batteries with 400% stretchability. (H) The stable capacity retention under different tensile strain values (reproduced from Yao et al. (2021)). (I) Kirigami strategies to realize Zn–air batteries with 100% stretchability to power a fan (reproduced from Qu et al. (2020)). (J) The PAM hydrogel electrolyte for mild Zn battery systems with a stretchability over 3,000% (reproduced from Li et al. (2020)). (K) The PANa hydrogel electrolyte for alkaline Zn battery systems with a stretchability over 1,100% (reproduced from Ma et al. (2019)).
Structural Designs for Stretchable Zn-Based Batteries
There are two types of structural designs applied in stretchable Zn-based batteries, namely, fiber-shaped batteries and kirigami structure–modified batteries. Generally, these devices in planar configurations are not intrinsically stretchable by themselves, but stretchability was realized when the battery was built up into a serpentine fiber shape or kirigami shape.
As for the fiber-shaped batteries, one representative example in Zn-based batteries is as follows: Li et al. (2018) have first utilized facile methods to coat/deposit the active cathode and anode materials on two separate conductive yarns and then wrapped them around an elastic fiber, finally obtaining stretchable yarn–Zn–MnO2 batteries (Figure 2F). The as-fabricated battery demonstrated an outstanding mechanical stretchability of up to 300% deformation. The fiber-shaped batteries also deliver good tailorability and outstanding energy density (53.8 mW h cm−3). In addition, the fiber-shaped Zn–Ag2O battery was also designed by sequentially depositing the zinc anode, gel electrolyte, and Ag cathode onto a spring-like copper current collector with remarkable rate 3.5 mA h cm−2, where the battery could be stretched to 100% and could maintain its capacity after 500 times of deformation (Zamarayeva et al., 2017; Chu et al., 2021). Another example of the fiber-shaped Zn-ion battery is fabricated by inserting the cathode (Mg0.23V2O5) and anode (Zn wire) into a curled spring to obtain stretchability (Xu et al., 2020). The prospect of the fiber-shaped battery is promising that, despite being capable of withstanding large stretching stresses, another important application scenario is to be integrated into the energy fabric to realize a large area of integrated energy storage cloth (Chen S. et al., 2020; Wang L. et al., 2020).
Another workable approach was applying kirigami strategies by cutting and folding planar batteries for strain-tolerant configurations, while the strain was redistributed relying on performing out-of-plane deformation at local positions and reduced by the blank area. Kirigami-derived configurations (e.g., cellular, pyramid, and basket patterns) have already been explored for stretchable Li-ion batteries and supercapacitors (Fu et al., 2016; Ning et al., 2018), while such configuration designs have recently been demonstrated for stretchable Zn-based batteries. One advantage of kirigami-shaped batteries is their capability of maintaining planar geometries, which make them ready to be integrated with other wearable electronics compared to other mountainous structures. For example, Yao et al. (2021) have demonstrated editable and cuttable polyaniline–Zn batteries through different editing programs as a stretchable honeycomb-like structure, which could deliver stable electrochemical performance even enduring the tensile strain of up to 400% (Figures 2G,H). In addition, the stretchable Zn–air battery was also demonstrated by the kirigami-cutting strategy, obtaining a 160% stretchable serpentine-shaped battery (Qu et al., 2020) (Figure 2I), where stretchable Zn–air battery can deliver 11.5 Wh L−1 and the performance was stable even subjected to 100% deformation with slight variation of the output voltage compared to the initial state.
Stretchable Electrolytes for Stretchable Zn-Based Batteries
After discussing the material and structural design, the main strategy to realize stretchability of electrode materials is to combine them with elastic substrates. Regarding aqueous electrolytes as another significant component, polymer-based electrolytes were designed and applied, which not only act as ion conductors but also as separators (Lopez et al., 2019; Chen P. et al., 2020; Wang Z. et al., 2020). The most attractive is the designated properties from these polymer networks, such as stretchability, antifreezing, and self-healing properties (Lu et al., 2020). Specifically, to enhance the mechanical stretchability of polymeric electrolytes, a dual-network strategy can be applied by introducing two sorts of polymer networks to disperse the external force (Ma et al., 2019). In addition, to endow the functionality, modifications of foreign functional molecules, such as antifreezing molecules (Mo et al., 2019), can be introduced into polymeric networks to endow the whole electrolyte with the antifreezing properties. Very recently, there are some detailed reviews on the designing strategies on polymer networks for aqueous batteries for specific hydrogel designing chemistries (Wang Z. et al., 2020; Mohanta et al., 2020).
For Zn-based batteries, there are generally two types of aqueous electrolytes, namely, mild electrolytes and alkaline electrolytes, which have different requirements on stretchable electrolytes. First, regarding the mild electrolytes, the highly cross-linked polyacrylamide (PAM) hydrogel was demonstrated with a high ionic conductivity of up to 17.3 × 10−3 S cm−1 and remarkable stretchability of up to 3,000% deformation (Figure 2J) (Li et al., 2018), which has successfully been applied to different Zn-ion battery systems in mild electrolytes, where the battery performance can accommodate a high specific capacity of 302.1 mA h g−1 and a volumetric energy density of 53.8 mWh cm−3. In addition, these delivered a high capacity retention of 94.8% after cycling 100 times when subjected to a strain of 300%.
On the other hand, regarding the alkaline electrolytes, the sodium polyacrylate (PANa) hydrogel was applied for nickel–cobalt–based cathodes as well as air cathodes. However, the stretchability of PANa polymer networks is not satisfying, showing even decrease in the alkaline environment. Improving strategies have been applied by introducing cellulose to build dual-network hydrogel that can be stretched over 1,100% (Ma et al., 2019). The as-obtained Zn–air batteries can be stretched up to 800% deformation (Figure 2K). Apart from the realization of stretchability, the as-fabricated Zn–air battery exhibits a high power density of 108.6 mW cm−2, increasing to 210.5 mW cm−2 upon being 800% stretched, because of the increased contact areas between active materials and the hydrogel electrolyte. It should be noted that the adhesion is one of the most significant factor to determine the stability of flexible/stretchable batteries with multiple layers, especially for the hydrogel electrolyte layer and electrode layers. Introducing effective adhesives can solve this issue, but it needs more delicate material designs to guarantee the adhesion and resilience at the wet interface.
Improving Directions for Future Stretchable Zn-Based Batteries
Through material and structural designs, stretchable zinc batteries can be realized with remarkable stretchability and good performance retention after cycled stretching. However, there are still some aspects, from our point of view, that need to be further strengthened from scientific and technical points.
The first is the low utilization of active materials. Generally, high stretchability and high electrochemical performance trade off with each other, because the system definitely needs the introduction of extra dispersing materials to enable stretchability and redundancy in volume to release strain in batteries. Thus, careful calculations are needed to decrease the gravimetric and volumetric capacity to improve corresponding energy densities (Wang D. et al., 2020). The second is the poor contacting and adhering forces at the interface of different layers. Considering batteries are always layered structures with layer-by-layer stacking of different materials based on weak physical bonding, they are easy to delaminate under external stresses. Thus, it is necessary to introduce chemical bonds at the interface and simultaneously attempt to reduce the shearing force at the interface of each specific spot. In addition, the configurational designs to dilute the external applied forces are another type of strategies to maintain the structural and performance stability (Liao et al., 2019; Qian et al., 2019; Hansen and Liu, 2021). The third is that more number of functionalities should be added into the stretchability to realize the final application as wearable power supplies. Material properties through the material design, such as antifreeze, self-healing, waterproof, and degradable features, can be introduced, especially through the applied electrolytes. The fourth is that the stretchable batteries should be integrated into the wearable electronic system (Liang et al., 2017b), and there would be some derivative requirements on such stretchable batteries, such as softness and skin conformability. Thus, a more in-depth understanding of compatible integration of as-fabricated stretchable Zn batteries is needed. The fifth is the evaluation parameters on stretchability. Considering different battery designs and that their performances are evaluated under different variable parameters, such as the stretching strain and thickness of the device, it would be informative and helpful to compare the battery performance once the evaluating parameters are unified. The sixth is the scalability of stretchable batteries. Scalability is the final target to developing the stretchable batteries. Developing suitable materials compatible with the current fabrication equipment is the primary issue, where the corresponding scientific challenges correlate with the addition of stretchable components into the battery configurations and meanwhile guarantee the battery performance. Some successful attempts for scalability have been demonstrated, such as screen printing for Ag–Zn alkaline batteries (Kumar et al., 2017), which can be applied for large-scale preparation based on other battery material systems.
Conclusion
The field of stretchable Zn-based batteries has witnessed rapid development in the past few years, showing huge potential for practical wearable applications. We summarize the state-of-the-art stretchable aqueous Zn-based batteries from aspects of material designs and structural designs. It should be emphasized that, even though batteries in rigid configurations have received more attentions than stretchable/flexible batteries, the developments of stretchable devices is beginning to catch up. Continuous efforts will be devoted to pursuing higher energy density, mechanical stability under stretched state, low-cost fabrication strategies. We hope that this review can attract more attentions on the rational designs on battery materials and structures for stretchable aqueous Zn batteries to be well-integrated into human’s daily life.
Author Contributions
GJ conceived the idea. LH wrote the manuscript, and GJ revised it. All authors discussed the topics and contributed to the organization of this paper.
Funding
This work is supported by the National Natural Science Foundation of China (NSFC) (Grant Nos. 51903162 and U1903120), Science Foundation of Guangdong Second Provincial General hospital (YN 2018-001), Doctoral Workstation Foundation of Guangdong Second Provincial General Hospital (2019BSG 2024), and Guangzhou Science and Technology Plan Project (202102020646).
Conflict of Interest
The authors declare that the research was conducted in the absence of any commercial or financial relationships that could be construed as a potential conflict of interest.
Publisher’s Note
All claims expressed in this article are solely those of the authors and do not necessarily represent those of their affiliated organizations, or those of the publisher, the editors and the reviewers. Any product that may be evaluated in this article, or claim that may be made by its manufacturer, is not guaranteed or endorsed by the publisher.
References
Chawla, N., Bharti, N., and Singh, S. (2019). Recent Advances in Non-flammable Electrolytes for Safer Lithium-Ion Batteries. Batteries 5 (1), 19. doi:10.3390/batteries5010019
Chen, A., Guo, X., Yang, S., Liang, G., Li, Q., Chen, Z., et al. (2021). Human Joint-Inspired Structural Design for a Bendable/foldable/stretchable/twistable Battery: Achieving Multiple Deformabilities. Energy Environ. Sci. 14 (6), 3599–3608. doi:10.1039/D1EE00480H
Chen, P., Zhang, K., Tang, D., Liu, W., Meng, F., Huang, Q., et al. (2020a). Recent Progress in Electrolytes for Zn-Air Batteries. Front. Chem. 8 (372). doi:10.3389/fchem.2020.00372
Chen, S., Ma, L., Wu, S., Wang, S., Li, Z., Emmanuel, A. A., et al. (2020b). Uniform Virus‐Like Co-N-cs Electrocatalyst Derived from Prussian Blue Analog for Stretchable Fiber‐Shaped Zn-Air Batteries. Adv. Funct. Mater. 30 (10), 1908945. doi:10.1002/adfm.201908945
Chu, W., Jiang, T., and Ho, P. S. (2021). Effect of Wiring Density and Pillar Structure on Chip Packaging Interaction for Mixed-Signal Cu Low K Chips. IEEE Trans. Device Mater. Relib., 1. doi:10.1109/TDMR.2021.3082043
Du, K., Wang, C., Subasinghe, L. U., Gajella, S. R., Law, M., Rudola, A., et al. (2020). A Comprehensive Study on the Electrolyte, Anode and Cathode for Developing Commercial Type Non-flammable Sodium-Ion Battery. Energ. Storage Mater. 29, 287–299. doi:10.1016/j.ensm.2020.04.021
Fang, G., Zhou, J., Pan, A., and Liang, S. (2018). Recent Advances in Aqueous Zinc-Ion Batteries. ACS Energ. Lett. 3 (10), 2480–2501. doi:10.1021/acsenergylett.8b01426
Fang, G., Zhu, C., Chen, M., Zhou, J., Tang, B., Cao, X., et al. (2019). Suppressing Manganese Dissolution in Potassium Manganate with Rich Oxygen Defects Engaged High‐Energy‐Density and Durable Aqueous Zinc‐Ion Battery. Adv. Funct. Mater. 29 (15), 1808375. doi:10.1002/adfm.201808375
Fu, K. K., Cheng, J., Li, T., and Hu, L. (2016). Flexible Batteries: From Mechanics to Devices. ACS Energ. Lett. 1 (5), 1065–1079. doi:10.1021/acsenergylett.6b00401
Gaikwad, A. M., Zamarayeva, A. M., Rousseau, J., Chu, H., Derin, I., and Steingart, D. A. (2012). Highly Stretchable Alkaline Batteries Based on an Embedded Conductive Fabric. Adv. Mater. 24 (37), 5071–5076. doi:10.1002/adma.201201329
Gu, M., Song, W.-J., Hong, J., Kim, S. Y., Shin, T. J., Kotov, N. A., et al. (2019). Stretchable Batteries with Gradient Multilayer Conductors. Sci. Adv. 5 (7), eaaw1879. doi:10.1126/sciadv.aaw1879
Guo, B., Liang, G., Yu, S., Wang, Y., Zhi, C., and Bai, J. (2021). 3D Printing of Reduced Graphene Oxide Aerogels for Energy Storage Devices: A Paradigm from Materials and Technologies to Applications. Energ. Storage Mater. 39, 146–165. doi:10.1016/j.ensm.2021.04.021
Hansen, E. J., and Liu, J. (2021). Materials and Structure Design for Solid-State Zinc-Ion Batteries: A Mini-Review. Front. Energ. Res. 8 (616665). doi:10.3389/fenrg.2020.616665
Huang, S., Liu, Y., Zhao, Y., Ren, Z., and Guo, C. F. (2019). Flexible Electronics: Stretchable Electrodes and Their Future. Adv. Funct. Mater. 29 (6), 1805924. doi:10.1002/adfm.201805924
Kumar, R., Johnson, K. M., Williams, N. X., and Subramanian, V. (2019). Scaling Printable Zn-Ag 2 O Batteries for Integrated Electronics. Adv. Energ. Mater. 9 (13), 1803645. doi:10.1002/aenm.201803645
Kumar, R., Shin, J., Yin, L., You, J. M., Meng, Y. S., and Wang, J. (2017). All‐Printed, Stretchable Zn‐Ag 2 O Rechargeable Battery via Hyperelastic Binder for Self‐Powering Wearable Electronics. Adv. Energ. Mater. 7 (8), 1602096. doi:10.1002/aenm.201602096
Kundu, D., Adams, B. D., Duffort, V., Vajargah, S. H., and Nazar, L. F. (2016). A High-Capacity and Long-Life Aqueous Rechargeable Zinc Battery Using a Metal Oxide Intercalation Cathode. Nat. Energ. 1 (10), 16119. doi:10.1038/nenergy.2016.119
Lee, G.-H., Moon, H., Kim, H., Lee, G. H., Kwon, W., Yoo, S., et al. (2020). Multifunctional Materials for Implantable and Wearable Photonic Healthcare Devices. Nat. Rev. Mater. 5 (2), 149–165. doi:10.1038/s41578-019-0167-3
Li, H., Liu, Z., Liang, G., Huang, Y., Huang, Y., Zhu, M., et al. (2018). Waterproof and Tailorable Elastic Rechargeable Yarn Zinc Ion Batteries by a Cross-Linked Polyacrylamide Electrolyte. ACS Nano 12 (4), 3140–3148. doi:10.1021/acsnano.7b09003
Li, R., Li, L., Jia, R., Jiang, K., Shen, G., and Chen, D. (2020). A Flexible Concentric Circle Structured Zinc‐Ion Micro‐Battery with Electrodeposited Electrodes. Small Methods 4 (9), 2000363. doi:10.1002/smtd.202000363
Liang, G., Hu, H., Liao, L., He, Y., and Ye, C. (2017a). Highly Flexible and Bright Electroluminescent Devices Based on Ag Nanowire Electrodes and Top-Emission Structure. Adv. Electron. Mater. 3 (3), 1600535. doi:10.1002/aelm.201600535
Liang, G., Mo, F., Ji, X., and Zhi, C. (2021). Non-metallic Charge Carriers for Aqueous Batteries. Nat. Rev. Mater. 6 (2), 109–123. doi:10.1038/s41578-020-00241-4
Liang, G., Yi, M., Hu, H., Ding, K., Wang, L., Zeng, H., et al. (2017b). Coaxial-Structured Weavable and Wearable Electroluminescent Fibers. Adv. Electron. Mater. 3 (12), 1700401. doi:10.1002/aelm.201700401
Liao, X., Shi, C., Wang, T., Qie, B., Chen, Y., Yang, P., et al. (2019). High‐Energy‐Density Foldable Battery Enabled by Zigzag‐Like Design. Adv. Energ. Mater. 9 (4), 1802998. doi:10.1002/aenm.201802998
Lopez, J., Mackanic, D. G., Cui, Y., and Bao, Z. (2019). Designing Polymers for Advanced Battery Chemistries. Nat. Rev. Mater. 4 (5), 312–330. doi:10.1038/s41578-019-0103-6
Lu, K., Jiang, T., Hu, H., and Wu, M. (2020). Hydrogel Electrolytes for Quasi-Solid Zinc-Based Batteries. Front. Chem. 8 (885), 546728. doi:10.3389/fchem.2020.546728
Ma, L., Chen, S., Wang, D., Yang, Q., Mo, F., Liang, G., et al. (2019). Super‐Stretchable Zinc-Air Batteries Based on an Alkaline‐Tolerant Dual‐Network Hydrogel Electrolyte. Adv. Energ. Mater. 9 (12), 1803046. doi:10.1002/aenm.201803046
Mackanic, D. G., Chang, T.-H., Huang, Z., Cui, Y., and Bao, Z. (2020a). Stretchable Electrochemical Energy Storage Devices. Chem. Soc. Rev. 49 (13), 4466–4495. doi:10.1039/D0CS00035C
Mackanic, D. G., Kao, M., and Bao, Z. (2020b). Enabling Deformable and Stretchable Batteries. Adv. Energ. Mater. 10 (29), 2001424. doi:10.1002/aenm.202001424
Mo, F., Liang, G., Huang, Z., Li, H., Wang, D., and Zhi, C. (2020). An Overview of Fiber‐Shaped Batteries with a Focus on Multifunctionality, Scalability, and Technical Difficulties. Adv. Mater. 32 (5), 1902151. doi:10.1002/adma.201902151
Mo, F., Liang, G., Meng, Q., Liu, Z., Li, H., Fan, J., et al. (2019). A Flexible Rechargeable Aqueous Zinc Manganese-Dioxide Battery Working at −20 °C. Energ. Environ. Sci. 12 (2), 706–715. doi:10.1039/C8EE02892C
Mohanta, J., Kang, D.-W., Cho, J. S., Jeong, S. M., and Kim, J.-K. (2020). Stretchable Electrolytes for Stretchable/flexible Energy Storage Systems - Recent Developments. Energ. Storage Mater. 28, 315–324. doi:10.1016/j.ensm.2020.03.009
Ning, X., Wang, X., Zhang, Y., Yu, X., Choi, D., Zheng, N., et al. (2018). Assembly of Advanced Materials into 3D Functional Structures by Methods Inspired by Origami and Kirigami: A Review. Adv. Mater. Inter. 5 (13), 1800284. doi:10.1002/admi.201800284
Pan, H., Shao, Y., Yan, P., Cheng, Y., Han, K. S., Nie, Z., et al. (2016). Reversible Aqueous Zinc/manganese Oxide Energy Storage from Conversion Reactions. Nat. Energ. 1 (5), 16039. doi:10.1038/nenergy.2016.39
Qian, G., Liao, X., Zhu, Y., Pan, F., Chen, X., and Yang, Y. (2019). Designing Flexible Lithium-Ion Batteries by Structural Engineering. ACS Energ. Lett. 4 (3), 690–701. doi:10.1021/acsenergylett.8b02496
Qu, S., Liu, B., Wu, J., Zhao, Z., Liu, J., Ding, J., et al. (2020). Kirigami-Inspired Flexible and Stretchable Zinc-Air Battery Based on Metal-Coated Sponge Electrodes. ACS Appl. Mater. Inter. 12 (49), 54833–54841. doi:10.1021/acsami.0c17479
Rogers, J., Bao, Z., and Lee, T.-W. (2019). Wearable Bioelectronics: Opportunities for Chemistry. Acc. Chem. Res. 52 (3), 521–522. doi:10.1021/acs.accounts.9b00048
Say, M. G., Brooke, R., Edberg, J., Grimoldi, A., Belaineh, D., Engquist, I., et al. (2020). Spray-coated Paper Supercapacitors. Npj Flex Electron. 4 (1), 14. doi:10.1038/s41528-020-0079-8
Song, W. J., Hwang, C., Lee, S., Kong, M., Kim, J., Park, H. K., et al. (2020). Design of a Janus‐Faced Electrode for Highly Stretchable Zinc-Silver Rechargeable Batteries. Adv. Funct. Mater. 30 (42), 2004137. doi:10.1002/adfm.202004137
Song, W. J., Yoo, S., Song, G., Lee, S., Kong, M., Rim, J., et al. (2019). Recent Progress in Stretchable Batteries for Wearable Electronics. Batteries. Supercaps 2 (3), 181–199. doi:10.1002/batt.201800140
Song, Z., Ma, T., Tang, R., Cheng, Q., Wang, X., Krishnaraju, D., et al. (2014). Origami Lithium-Ion Batteries. Nat. Commun. 5 (1), 3140. doi:10.1038/ncomms4140
Stock, D., Dongmo, S., Janek, J., and Schröder, D. (2019). Benchmarking Anode Concepts: The Future of Electrically Rechargeable Zinc-Air Batteries. ACS Energ. Lett. 4 (6), 1287–1300. doi:10.1021/acsenergylett.9b00510
Wang, D., Han, C., Mo, F., Yang, Q., Zhao, Y., Li, Q., et al. (2020a). Energy Density Issues of Flexible Energy Storage Devices. Energ. Storage Mater. 28, 264–292. doi:10.1016/j.ensm.2020.03.006
Wang, L., Fu, X., He, J., Shi, X., Chen, T., Chen, P., et al. (2020b). Application Challenges in Fiber and Textile Electronics. Adv. Mater. 32 (5), 1901971. doi:10.1002/adma.201901971
Wang, Z., Li, X., Yang, Z., Guo, H., Tan, Y. J., Susanto, G. J., et al. (2021). Fully Transient Stretchable Fruit‐based Battery as Safe and Environmentally Friendly Power Source for Wearable Electronics. EcoMat 3 (1), e12073. doi:10.1002/eom2.12073
Wang, Z., Zhu, M., Pei, Z., Xue, Q., Li, H., Huang, Y., et al. (2020c). Polymers for Supercapacitors: Boosting the Development of the Flexible and Wearable Energy Storage. Mater. Sci. Eng. R: Rep. 139, 100520. doi:10.1016/j.mser.2019.100520
Xu, W., Liu, C., Wu, Q., Xie, W., Kim, W.-Y., Lee, S.-Y., et al. (2020). A Stretchable Solid-State Zinc Ion Battery Based on a Cellulose Nanofiber-Polyacrylamide Hydrogel Electrolyte and a Mg0.23V2O5·1.0H2O Cathode. J. Mater. Chem. A. 8 (35), 18327–18337. doi:10.1039/D0TA06467J
Yan, C., Wang, X., Cui, M., Wang, J., Kang, W., Foo, C. Y., et al. (2014). Stretchable Silver-Zinc Batteries Based on Embedded Nanowire Elastic Conductors. Adv. Energ. Mater. 4 (5), 1301396. doi:10.1002/aenm.201301396
Yao, M., Yuan, Z., Li, S., He, T., Wang, R., Yuan, M., et al. (2021). Scalable Assembly of Flexible Ultrathin All‐in‐One Zinc‐Ion Batteries with Highly Stretchable, Editable, and Customizable Functions. Adv. Mater. 33 (10), 2008140. doi:10.1002/adma.202008140
Yin, L., Lv, J., and Wang, J. (2020). Structural Innovations in Printed, Flexible, and Stretchable Electronics. Adv. Mater. Technol. 5 (11), 2000694. doi:10.1002/admt.202000694
Yin, L., Seo, J. K., Kurniawan, J., Kumar, R., Lv, J., Xie, L., et al. (2018). Highly Stable Battery Pack via Insulated, Reinforced, Buckling‐Enabled Interconnect Array. Small 14 (43), 1800938. doi:10.1002/smll.201800938
Zamarayeva, A. M., Ostfeld, A. E., Wang, M., Duey, J. K., Deckman, I., Lechêne, B. P., et al. (2017). Flexible and Stretchable Power Sources for Wearable Electronics. Sci. Adv. 3 (6), e1602051. doi:10.1126/sciadv.1602051
Zhang, H., Liu, X., Li, H., Hasa, I., and Passerini, S. (2021a). Challenges and Strategies for High‐Energy Aqueous Electrolyte Rechargeable Batteries. Angew. Chem. Int. Ed. 60 (2), 598–616. doi:10.1002/anie.202004433
Zhang, T., Tang, Y., Fang, G., Zhang, C., Zhang, H., Guo, X., et al. (2020a). Electrochemical Activation of Manganese‐Based Cathode in Aqueous Zinc‐Ion Electrolyte. Adv. Funct. Mater. 30 (30), 2002711. doi:10.1002/adfm.202002711
Zhang, W., Zhai, X., Zhang, Y., Wei, H., Ma, J., Wang, J., et al. (2020b). Application of Manganese-Based Materials in Aqueous Rechargeable Zinc-Ion Batteries. Front. Energ. Res. 8 (195). doi:10.3389/fenrg.2020.00195
Zhang, Y., Deng, Y.-P., Wang, J., Jiang, Y., Cui, G., Shui, L., et al. (2021b). Recent Progress on Flexible Zn-Air Batteries. Energ. Storage Mater. 35, 538–549. doi:10.1016/j.ensm.2020.09.008
Zhang, Y., Huang, Y., and Rogers, J. A. (2015). Mechanics of Stretchable Batteries and Supercapacitors. Curr. Opin. Solid State. Mater. Sci. 19 (3), 190–199. doi:10.1016/j.cossms.2015.01.002
Zhou, B., He, D., Hu, J., Ye, Y., Peng, H., Zhou, X., et al. (2018). A Flexible, Self-Healing and Highly Stretchable Polymer Electrolyte via Quadruple Hydrogen Bonding for Lithium-Ion Batteries. J. Mater. Chem. A. 6 (25), 11725–11733. doi:10.1039/C8TA01907J
Zhou, M., Guo, S., Li, J., Luo, X., Liu, Z., Zhang, T., et al. (2021). Surface‐Preferred Crystal Plane for a Stable and Reversible Zinc Anode. Adv. Mater. 33 (21), 2100187. doi:10.1002/adma.202100187
Keywords: stretchable batteries, aqueous Zn-based batteries, wearable batteries, material design, battery structural design
Citation: Hang L and Jiang G (2021) Realizing Stretchable Aqueous Zn–Based Batteries by Material and Structural Designs. Front. Energy Res. 9:739150. doi: 10.3389/fenrg.2021.739150
Received: 10 July 2021; Accepted: 27 July 2021;
Published: 24 August 2021.
Edited by:
Deping Li, Harbin Institute of Technology, ChinaReviewed by:
Li Xinliang, City University of Hong Kong, ChinaGuozhao Fang, Central South University, China
Funian Mo, Harbin Institute of Technology, China
Copyright © 2021 Hang and Jiang. This is an open-access article distributed under the terms of the Creative Commons Attribution License (CC BY). The use, distribution or reproduction in other forums is permitted, provided the original author(s) and the copyright owner(s) are credited and that the original publication in this journal is cited, in accordance with accepted academic practice. No use, distribution or reproduction is permitted which does not comply with these terms.
*Correspondence: Lifeng Hang, aGFuZ2xmQHVzdGMuZWR1LmNu; Guihua Jiang, amlhbmdnaEBnZDJoLm9yZy5jbg==