- 1State Key Laboratory of Environmental Criteria and Risk Assessment, Chinese Research Academy of Environmental Sciences, Beijing, China
- 2Key Laboratory of Organic Optoelectronics and Molecular Engineering, Ministry of Education, Department of Chemistry, Tsinghua University, Beijing, China
- 3State Key Laboratory of Tribology, Department of Mechanical Engineering, Tsinghua University, Beijing, China
Moisture is a ubiquitous and clean resource in nature, which continuously diffuses in the atmosphere and demonstrates huge chemical potential energy that is difficult to be utilized. Recently, the generation of power from interactions between graphene and gaseous water molecules in moisture has triggered great research interest that could provide a novel energy conversion system for our society. graphene-based assemblies have been considered as ideal platforms for moist-electric generation (MEG) in many studies, because of the abundant of functional groups, controllable microstructure and diverse macro morphologies. Therefore, in this short review, we will first state the preparation techniques of graphene-based assemblies for MEG. Then, the fundamental mechanisms of MEG are discussed and the latest advances on graphene MEG are reviewed. Finally, an overview of the current challenges and future development trends in graphene MEG is provided.
1 Introduction
The energy crisis and global warming caused by the increasing utilization of fossil fuels have created an urgent need for the development of sustainable and green energy resources (David, 2004; Steven and Arun, 2012; Chu et al., 2017). Electricity is the most important secondary energy in modern society, and the use of electric power is the outstanding feature of the second industrial revolution. Since the dawn of the age of electricity, this form of energy has had a great impact on human society by promoting rapid development. In recent years, research efforts have been focused on the generation of electricity from renewable natural resources such as light, heat, and mechanical movement (He et al., 2019; Jin et al., 2019; Jung et al., 2019), and currently, electric power is commonly generated from wind, solar energy, water flow energy (hydropower), and thermal energy. The hydrovoltaic effect is also used to produce electricity, based on the direct interaction between nanostructures and flowing, waving, dropping, or evaporating water. (Yin et al., 2014a; Yin et al., 2014b; Xue et al., 2017; Hou et al., 2018; Yao et al., 2020). The water energy resource is considered 100% environmentally friendly. Back in 1859, Quincke was the first to succeed in generating electricity from water by direct interaction between water and solid. Specifically, he created an electric voltage by flowing electrolytes through a narrow channel under a pressure gradient (van der Heyden et al., 2005). Since then, many studies have confirmed that electricity may be generated directly in nanomaterials, including carbon nanoparticles, carbon nanotubes, polymer materials, graphene, and nanostructured silicon, under the effects of water flow, waves, and water evaporation (Zhao et al., 2008; Fei et al., 2019; Zhang et al., 2019; Qin et al., 2020a; Kuriya et al., 2020; Zhou et al., 2020). Based on this principle, Wang et al. (Wang et al., 2021) have designed and developed a heterogeneous moisture-enabled electric generator that can yield voltages greater than 1,000 V under ambient conditions (25% relative humidity (RH), 25°C).
In a previous study, we had shown that graphene oxide (GO) assemblies with an oxygen gradient can induce a gradient of protons under moisture ingress, and in turn, the migration of protons induces a voltage output of ∼40 mV (Zhao et al., 2017a). The method is called MEG, and the voltage produced is much higher than the waving potential (Figure 1). The stimulation of pristine GO by moisture can also be used to generate electricity (Liang et al., 2018). In general, graphene assemblies with different dimensions have various power generation properties.
As the thinnest two-dimensional material in the world, graphene has sparked tremendous interest over the past few decades. Owing to its extraordinary properties of large theoretical specific surface area (2,600 m2 g−1), high carrier mobility at room temperature (15,000 cm2 V−1 s−1), remarkable thermal conductivity (3,000–5000 W m−1 K−1), high electrical conductivity (up to 6 × 103 S cm−1), and excellent optical transparency (97.3%) and reflectance (<0.1%), this material is of great value in multiple disciplines (Stankovich et al., 2006; Geim and Novoselov, 2007; Lee et al., 2008; Geim, 2009). In particular, macroscopic graphene assemblies have attracted tremendous attention due to their promising applications in the fields of energy conversion and storage (Yang et al., 2016). Many researchers have attempted to assemble graphene sheets into one dimensional (1D) fibers, two dimensional (2D) membranes, and three dimensional (3D) foams using different methods, and based on the published studies, graphene has great potential for use in electronics (Meng et al., 2018; Them et al., 2021) and biology (Hu et al., 2018; Dong et al., 2020), as well as in energy and environmental applications (Chen et al., 2015; Chen et al., 2021; Han et al., 2021).
Several excellent reviews discuss the rapid development of MEG (Cheng et al., 2017; Xu et al., 2018a; Shen et al., 2020). In this review, we focus on the progress of MEG using graphene assemblies. First, the main methods used to prepare these assemblies are discussed, and the fundamental mechanisms of harvesting energy from moisture are briefly introduced. Then, the recent advances in developing graphene-based advanced functional materials for MEG are summarized. Finally, the future challenges and potential trends related to the application of MEG are assessed.
2 Technology of Graphene Assembly Preparation
The large-scale preparation of graphene assemblies such as 1D graphene fibers/micro-tubules (Cong et al., 2012; Cheng et al., 2013; Xin et al., 2016), 2D graphene films (Wang et al., 2013a; Xie et al., 2020), and 3D graphene foams promotes the application of these materials on an industrial level (Chen et al., 2013; Chen et al., 2014). In this section, we introduce several technologies that are commonly used to assemble graphene.
2.1 Template Assembly Technology
The template method is an important method used to synthesize nanocomposites and nanomaterials. This method makes use of cheap, readily available, nanostructured materials with flexible shapes as templates, which enables the controlled synthesis of nanomaterials with specific morphology and pore size. However, the removal of the template may affect the quality of the material. Previously, the templating method had been used by Zhuo et al. (Zhuo et al., 2021) to encapsulate uniform well-dispersed Sn nanoparticles in an amorphous carbon tube. In a pioneer study, Huang et al. (Huang et al., 2014) developed a general emulsion soft-template approach that was applied in the preparation of porous graphene foams with controllable pore morphologies (Figure 2A). Macroporous graphene foams were synthesized for the first time using simple soft-templated metal frameworks (Tynan et al., 2016). In three simple steps, our group fabricated multifunctional microchannel-network graphene foams by using an alumina fiber blanket template (Yan et al., 2014). We also developed a surfactant-foaming sol-gel method that can be used to effectively disrupt and reconstruct the GO LCs in dispersions via microbubble templates. The resulting graphene hydrogel bulk is characterized by large size and intact structure, which indicates that this method of graphene preparation can be applied on an industrial level (Yang et al., 2018a).
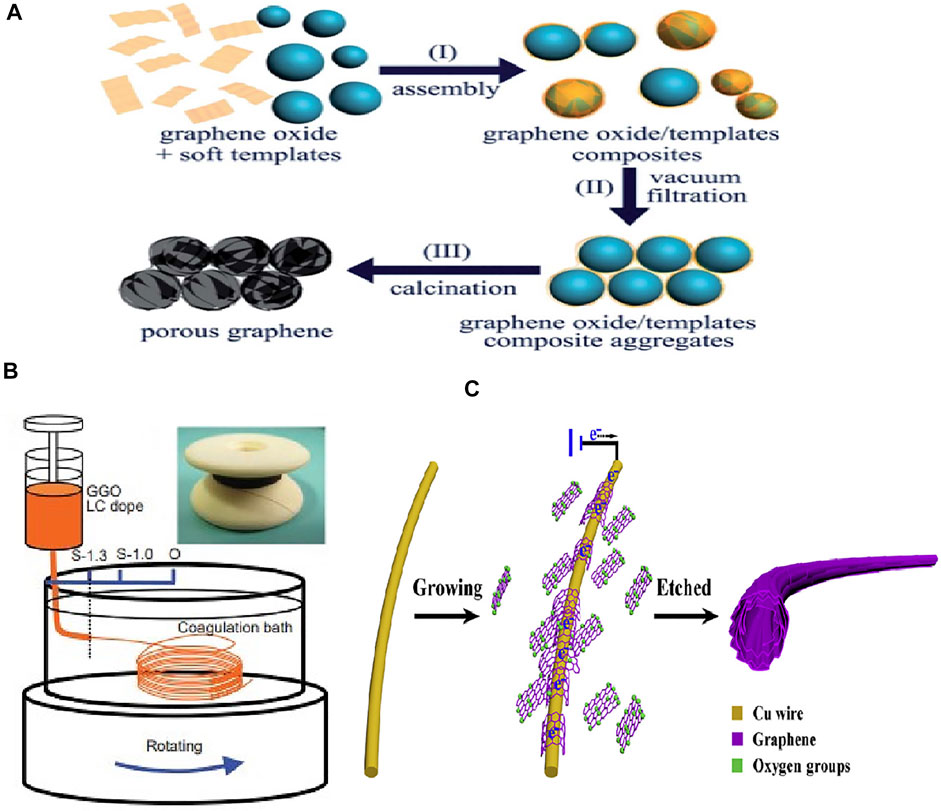
FIGURE 2. (A) Schematic illustration of the emulsion soft-template synthesis procedures for preparing porous graphene foams; (Figures reprinted from ref. 45 with permission. Copyright 2014; The Royal Society of Chemistry); (B) Schematic apparatus for spinning GGO fibers; (Figures reprinted from ref. 54 with permission. Copyright 2013; Wiley Online Library) (c) Preparation scheme of the electrochemically grown graphene microtube; (Figures reprinted from ref. 51 with permission. Copyright 2017; Elsevier Ltd.).
2.2 Wet Assembly Technology
Wet assembly technology mainly includes hydrothermal processes and liquid crystal wet spinning. Despite the advantages of the hydrothermal method, such as simple operation, mild reaction conditions, and wide application range, its use is limited by the difficulty in controlling the structure and morphology of the synthesized materials. A one-step hydrothermal process was used by Shi et al. to prepare a self-assembled graphene hydrogel for the first time by heating a homogeneous, aqueous GO dispersion in an autoclave. Using the same method, Zhao et al. (Zhao et al., 2012) prepared a novel versatile, ultralight, nitrogen-doped graphene framework, and Dong et al. (Dong et al., 2012) fabricated light, flexible, and multifunctional graphene fibers. Unlike the self-assembly method, the wet spinning method allows for the preparation of products whose morphology and structure can be well controlled simply by adjusting the composition of raw materials. Moreover, the excellent physical and chemical properties of graphene are maintained after wet spinning. So far, this method has been successfully used to synthesize ultra-strong graphene fibers (Xu et al., 2013) (Figure 2B), nitrogen-doped graphene films (Chu et al., 2020), and millimeter-scale super-elastic graphene aerogel spheres (Zhao et al., 2017b). The latter product has been prepared on a large scale by wet spinning of GO liquid crystals, followed by facile drying and thermal annealing.
2.3 Electrochemical Assembly Technology
The main advantages of electrochemical assembly technology are short preparation time, low temperature (room temperature), and eco-friendliness. Moreover, the morphology of the products synthesized by this technology can be well controlled, which favors its use by researchers. Previously, Yang et al. (Yang et al., 2018b) used the electrochemical technique to simultaneously deposit and reduce graphene oxides on a Cu wire template at room temperature (Figure 2C). After removing the template, a tubular structure with the shape of the Cu wire was obtained. Porous reduced graphene oxide/polypyrrole composite films were also prepared by a facile pulsed electrochemical method that involves blade casting of the GO gel (Chen et al., 2020). In addition to making use of a simple electrolyte precursor, this method preserves the porous structure of the GO gel.
2.4 Chemical Vapor Deposition
The CVD process characterized by excellent controllability of reaction conditions is an efficient method used to prepare high-quality graphene. In general, there are two types of CVD processes: 1) homogenous gas-phase reaction followed by physical deposition on substrate surfaces, and 2) heterogeneous chemical reaction on the surface of catalyst substrates (Qin et al., 2020b). In 2006, the CVD synthesis of few-layer graphene on nickel sheets was first reported by Somani et al. (Somani et al., 2006). Since then, the technology has developed rapidly, and in 2013, Wang et al. reported the direct CVD growth of graphene onto a CVD-grown h-BN film, resulting in the formation of a pristine graphene/hBN interface over a large area. Using this configuration of graphene assembly, the negative effect of substrate on the quality of graphene is eliminated (Wang et al., 2013b). Zeng et al. prepared 3D graphene fibers by electro-spinning, stabilization, carbonization, and CVD. The synthesized fibers possess a unique structure with fibrous shape, nanoscale pores, and exposed single-layer graphene edges, and their morphology can be effectively controlled and functionalized (Zeng et al., 2018). CVD was also used to grow hollow graphene tubes on a copper (Cu) wire substrate, and after removing the Cu template, the tubes obtained were drawn into graphene fibers (Chen and Dai, 2015). This study was the first to use the CVD method in fiber preparation. Using plasma-assisted thermal CVD, Chan et al. synthesized high-quality graphene films at low temperature (600°C) (Chan et al., 2013). Finally, Shi et al. directly prepared a 3D graphene foam on a shell substrate by CVD method, and their production process was scalable and cost-effective (Shi et al., 2016). Although the CVD method was a conventional and effective method to prepare graphene film, However, the graphene-based materials via CVD was hardly used for moisture induced electricity generation at present.
2.5 Other Technologies
3D printing technology (Wei et al., 2015; Fu et al., 2016; Ponnamma et al., 2021), the sol-gel method (Tang et al., 2014; Xu et al., 2016), direct freeze-drying (Yang et al., 2018b; Thomas and Agarwal, 2021), substrate-assisted reduction and GO assembly (Hu et al., 2013; Zhao et al., 2016a), and laser scribed patterning (El-Kady et al., 2012; Strong et al., 2012), among others (Ervin, 2015), are also effective methods of graphene assembly preparation. Due to their unique advantages, these methods are widely used in graphene assembly research.
3. Mechanism of Moisture-induced Electricity Generation
At present, the diffusion of ions stimulated by ion concentration gradient is widely accepted as the mechanism underlying moisture-induced power generation (Shen et al., 2020; Bai et al., 2019). Considering that the inner 2D-GO sheets are rich in oxygen-containing functional groups (e.g., -COOH) and have a large specific surface area, they can generate large quantities of protons (H+ ions) by absorbing ambient water molecules. If the concentration of protons on the graphene surface is different from that in the bulk, a concentration gradient will be formed, and H+ will spontaneously migrate from the high concentration region to the low concentration region, until a dynamic equilibrium is reached. The typical process is divided into four steps, as illustrated in Figure 3. First, ambient water molecules (i.e., moisture) are absorbed on the surface of the substrate material. Then, the functional groups on these sheets are dissolved, and numerous positively and/or negatively charged ions are released. Subsequently, the ions migrate, and electric potential is produced. Finally, the chemical potential energy is converted into electric potential power (Han et al., 2019).
Based on the mechanism detailed above, the formation of an ion concentration gradient is key to the generation of moisture-induced electricity. Currently, two strategies are used to form this gradient. The first strategy relies on controlling the chemical composition of the functional material surface by varying the type of material and the quantity and distribution of functional groups. For example, under the same RH conditions, materials with higher functional group contents release larger numbers of positive and negative ions. As for the second strategy, it is based on controlling the relative humidity. This means that different areas of the material must be exposed to different humidity conditions. The asymmetric moisturization of materials results in the development of high and low ion concentration regions, which triggers the diffusion of ions. Notably, the side of the material exposed to higher RH will release more charged ions than the side exposed to lower RH.
Besides the typical processes described above, Liu et al. (Liu et al., 2020) proposed that distribution of water molecules in MEG is important for the electricity generation. Meanwhile, water molecules in air naturally include ionized species or are ionized when adsorbed on the material surface. The ionized clusters (H(H2O)n+/HO(H2O)n−) donate charge (such as H+/e−) to the material, supplying the closed-loop current flow driven by the voltage resulting from the moisture gradient.
4 Graphene-Based Assemblies for MEG
4.1 Fibers for MEG
Due to the highly oriented arrangement of GO sheets, GO fibers are characterized by high porosity, large surface area, and fast electron transfer. Moreover, these fibers can be woven into wearable self-powered devices. According to Liang et al. (Liang et al., 2017), a graphene fiber that is less than 1-mm long and only 80-µm wide can supply a high voltage output of ca. 0.4 V upon varying the environmental RH (Figures 2C, 4A). The GO fiber precursor used by the authors was first prepared by large-scale wet-spinning; then, the GO fiber region was reduced by well-controlled region-selective laser irradiation technology. Finally, the concentration of oxygen-containing functional groups in the GO region was regulated by electrochemical treatment in order to obtain a non-uniform distribution, and the RGO-GO-RGO structure was formed. In the fiber power generator, RGO is used as the electrode and GO as the electrolyte. Inspired by this study, we designed a high-performance moisture-activated nanogenerator based on 1D gradient doped polypyrrole nanowire (GDNw) with a preformed sulfonate ion gradient. When the nanogenerator is exposed to moisture (△RH = 75%), the hydrophilic polypyrrole groups in the GDNw absorb water molecules. During this hydration process, a gradient distribution of Na+ ions is produced along the GDNw, which triggers the migration of Na+ from the high-concentration side to the low-concentration side. Although this work is not based on graphene, it shows that as long as there is an ion gradient, electricity can be generated (Nie et al., 2018).
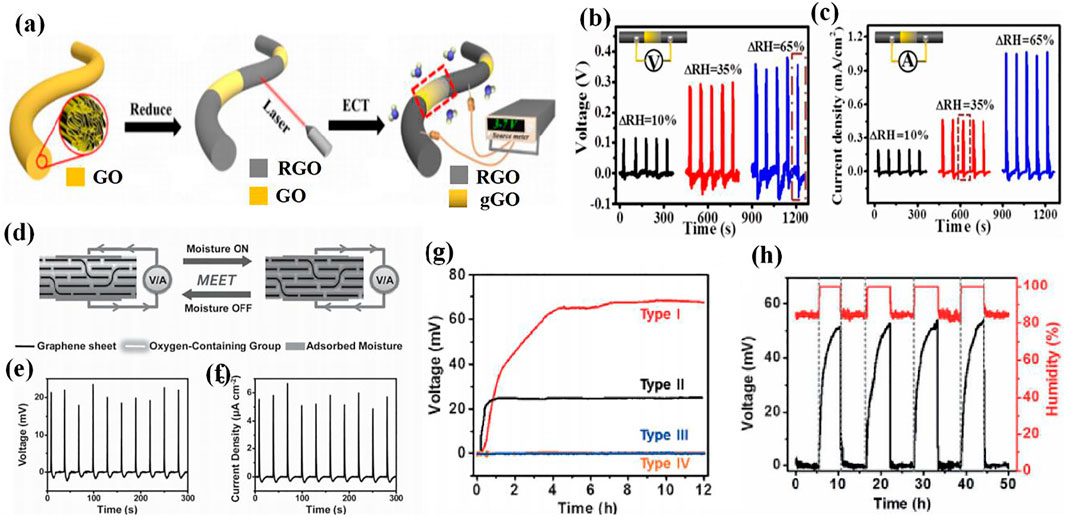
FIGURE 4. (A) Schematic illustration of the process of RGO-GO structure graphene fiber preparation. First, the GO fiber is prepared by wet spinning method. Then, it is reduced by laser selective writing. Finally, the distribution of functional groups is varied by electrochemical treatment. (B) Voltage and (C) current density responses of the RGO-GO unit under moisture stimulation (Figures reprinted from ref. 79 with permission. Copyright 2017; Elsevier). The insets show the testing circuits. (D) Schematic illustration of the MEG cycle. V and A represent the induced voltage and current along the direction of arrows, respectively. (E) Voltage and (F) current output cycles of a prototype MEG device of g-GOF in response to intermittent and periodic RH variation (ΔRH = 30%) (Figures reprinted from ref. 80 with permission. Copyright 2015; Wiley Online Library). (G) Open-circuit voltage generated by vapor adsorption, types I, II, III, and IV correspond to the sample with a half region functionalized in different ways; (H) Measured voltage of the device when the beaker was periodically sealed and unsealed. (Figures reprinted from ref. 90 with permission. Copyright 2016; Wiley Online Library).
4.2 Films for MEG
4.2.1 Graphene-Based Films
Currently, graphene-based films are most widely used in MEG research. In 2015, it was first discovered that graphene oxide films (GOFs) can generate electricity under moisture (Zhao et al., 2015). After polarization by moisture-electric annealing, the oxygen content in the GOF is gradually increased from top to bottom, and the g-GOF is formed. When the g-GOF is exposed to moisture, the gradient distribution of oxygen-containing groups results in a non-uniform release of free H+ from different parts of the film, with more ions being released from the O-rich part. Consequently, the H+ ions diffuse from the high concentration region to the low concentration region, thereby generating a strong induced potential and free electron movement in the external circuit. As shown in Figures 4D–F, the output voltage is about 35 mV, and the power density is about 4.2 mW m−2. In 2016, our group found that the surface structure of a single GO film is asymmetric, which indicates that the actuation ability of this film can be triggered by multiple stimuli such as moisture, heat, and infrared light. Moreover, the process of water adsorption/desorption occurring in oxygen-rich GO layers triggers fast and reversible expansion/contraction of GO films. Based on these findings, we conducted another study wherein we generated electric power by directly interacting the pristine graphene-oxide with water molecules. Once the material comes in contact with water vapor, an open-circuit voltage (Voc) of up to 0.4–0.7 V and a short-circuit current density of 2–25 μA cm−2 are generated by a single piece of GO film. Notably, the magnitude of the Voc signal increases rapidly with increasing film thickness; however, beyond 90 μm, this magnitude remains constant. In addition to film thickness, the voltage depends on the temperature (positive correlation) and incoming direction of moisture (Xu et al., 2018b).
In 2018, MEG technology witnessed extensive and rapid development. To improve the performance of electricity generation devices, researchers have attempted to control the composition and structure of materials. In general, the devices constructed using materials with rigid structures cannot be used in applications wherein the working conditions are complex and highly deformable (Fan et al., 2016). To overcome this limitation, our group designed a flexible in-plane moisture-electric converter (IPMEC) based on GO-assembled films for use in touchless interactive systems. The GO films were synthesized by vacuum filtration and subsequent peeling off; then, they were reduced by laser writing. The distribution of the oxygen-containing groups in the GO region of the IPMEC device was adjusted by electric field polarization, and during this process, the applied voltage was provided by the digital source table of a dual channel system. Upon exposure to moisture, the non-uniformly distributed functional groups in the GO combine with water molecules, resulting in the formation of an ion concentration gradient along the planar direction of inner GO sheets. Based on this technique, diverse self-powered touchless panels including finger position sensors for smart artificial skin, touchless switches, and even handwriting panels have been developed (Cheng et al., 2018a). To further improve the output voltage and device performance, a series of rollable, stretchable, 3D space-deformable, graphene-based MEG devices was developed by direct laser writing strategy. All generator units, including interdigital electrodes and circuits, were directly embedded in the flexible GO film. Despite being arbitrarily bent, this film exhibits excellent electricity generation ability. When the humidity in the atmosphere is varied, voltages as high as 1.5 V can be generated, which is enough to power a variety of commercial electronic devices (Yang et al., 2019). To reduce the cost of the device without compromising its efficiency, we used flexible, all-printable GO-functionalized paper as a moisture electric generator. This generator induces high voltages (2 V) that can power commercial liquid-crystal displays for portable electronic devices, and it is prepared by directly printing the well-ordered stacked GO nanosheets onto a moisture insulation substrate (MIS), such as glass, paper, or poly. Upon exposing the GO/MIS bilayer film to moisture, the GO nanosheets near the bare side are effectively moisturized, whereas those on the MIS side are not. Therefore, paper, a widely available, recyclable, and cheap resource that can be easily produced in large amounts and does not contribute to white pollution, is a viable moisture insulation substrate (Liang et al., 2018).
GQDs consist of nanometer-sized fragments of single- or few-layered graphene sheets. Due to quantum confinement and edge effects, the internal electron motion of GQDs is limited in all directions. In addition to their small size, GQDs have a large specific surface area and abundant edge sites, which endows them with excellent electrical and optical properties (Ponomarenko et al., 2008; Li et al., 2012; Yan et al., 2013; Hu et al., 2021). In a previous study, we had fabricated a high-performance MEG device by using small-sized (2–5 nm) GQDs that were prepared by direct oxidation and etching of natural graphite powder. To establish a gradient distribution of oxygen-containing functional groups in the material, moisture-assisted electrochemical treatment (ECT) was used. Based on our results, the voltage and current density produced by the as-fabricated nanogenerator are 270 mV and 27.7 mA cm−2, respectively, and the calculated power density is 1.68 mW cm−2. This work highlights a new method that can be used to harvest ambient energy (Huang et al., 2017). Although GQDs have great potential for constructing MEG devices, further research is needed.
4.2.2 Other Materials
Liu et al. (Liu et al., 2016)fabricated a device (size = 5*1 cm2) with a piece of porous carbon film, the voltage can increased to a maximum in 2 hours and then remained at a constant value of approximately 68 mV for over 6 h at fixed RH value (Figure 4G). They found that the output voltage was closely related to temperature and humidity (Figure 4H). They established the mathematical relationship between concentration gradient of protons and voltage-drop gradient along the device. They also concluded that the voltage is proportional to the proton concentration gradient and does not change with the device length or width, which was verified by altering the length/width of the device.
Considering that ordinary print paper is a low-cost biodegradable material that is rich in oxygen functional groups and micro-sized pores, we used it as hydroelectric material to construct paper-based moist-electric generators. The paper sheet (1.5 cm2) was sandwiched between a polyethylene terephthalate-indium tin oxide film and an Au-coated perforated stainless steel plate. The open-circuit voltage (Voc) of the device was found to be 0.25 V, and it increases linearly as more units are connected in series or parallel (Gao et al., 2019). Although printing paper is cheap, its application is limited by short service life.
The first attempt at constructing a direct and highly efficient polymer moist-electric generator (PMEG) based on the polyelectrolyte membrane of poly (4-styrensulfonic acid) (PSSA) was inspired by the process of electron and proton transport in the inner mitochondrial membrane. A 1 cm2 piece of pristine PSSA membrane provides an open-circuit-voltage (Voc) of up to ∼0.8 V and an Isc density as high as ∼0.1 mA cm (Steven and Arun, 2012) upon exposure to moisture. This work suggests that polymer films can greatly improve the service life of flexible power generators, which promotes the design and application of portable, wearable electronic devices that harvest energy from moisture (Xu et al., 2019).
In addition to the other generators, our group reported the first versatile moist-electric film generator (MEFG) integrated with transparent, self-healing, flexible, and arbitrary tailorable characters. The electrodes in the MEFG are conductive silver nanowire (Ag NW) networks, and the electricity-generating active material is the hydroscopic poly (4-styrensulfonic acid) and poly (vinyl alcohol) composite (PSS-PVA) film. A small 2 mm2 piece of this MEFG can produce a considerable voltage of about 0.6 V and a current of 2.0 μA, even if it is bent repeatedly. Furthermore, if the MEFG is broken, it undergoes a healing process upon exposure to moisture, such that it maintains its flexibility, transparency, and electric power efficiency (Wang et al., 2020).
4.3 3D-Foam for MEG
3D-GO structures with a gradient distribution of oxygen-containing functional groups are obtained by freeze-drying, tableting, and polarization. Previously, Zhao had fabricated a high-performance power generator based on super-hydrophilic 3D-GO. The non-uniform distribution of oxygen-containing groups in this material results in an ionic gradient upon the adsorption of moisture, which induces a periodic electric output under conditions wherein the RH is varied. Under tidal moisture, the generated output power can be as high as ca. 1 mW cm−2, and the energy conversion efficiency is ca. 52% (Zhao et al., 2016b).
The first graphene oxide foam (GOF) was synthesized in 2018, using the freeze-drying method. By applying the directional thermal reduction strategy, the asymmetric, porous GOF was obtained. This material consists of two layers: 1) the partially thermally reduced GO layer characterized by an uneven distribution of oxygen-containing groups, and 2) the unprocessed GO layer with homogeneous structure. Despite its small size (4 mm2), the as-prepared GOF can generate a sustained electrical voltage of up to 450 mV in air, with no need for any subsidiary conditions. By stacking many GOF units in series, voltages as high as 10 V may be achieved. Compared to other materials prepared previously, the performance of this device is much better (Cheng et al., 2018b).
Another efficient MEG device was constructed by skillfully incorporating a Schottky junction in a 3D GO aerogel (Figure 5A). First, the porous aerogel was prepared by direct lyophilization of the GO solution; then, the inner oxygen-containing functional groups were redistributed by controlled laser irradiation. The gradient distribution of these groups in the aerogel interior results in the formation of a Schottky junction at the interface. The output performance of the constructed material can reach ∼1.5 V, and 18 V may be achieved simply by connecting 15 units in series (Figures 5B,C). Such high voltage can easily power commercial electronic devices (Huang et al., 2018).
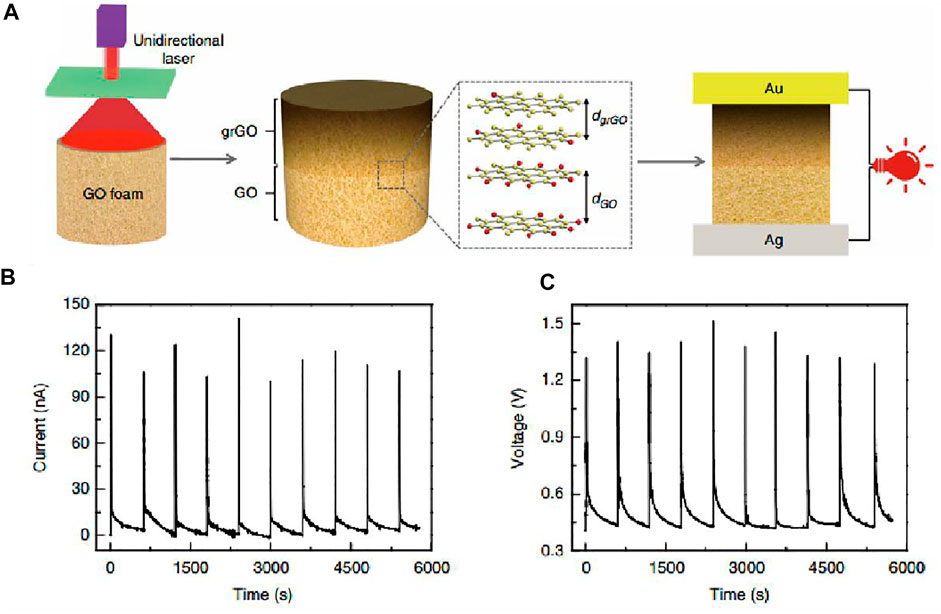
FIGURE 5. (A) Schematic illustration of the process of heterogeneous GO-based hygroelectric generator preparation. First, the porous GO foam was prepared by freeze-drying method; then, the material was reduced using a unidirectional laser. The resulting 3D-GO consists of a GO layer and a layer of reduced graphene oxide with a gradient distribution of functional groups and compact interlayer spacing. (B) Current and (C) voltage output cycle performances of the hygroelectric generator (Figures reprinted from ref. 91 with permission. Copyright 2018; Nature Publishing Group).
Recently, we reported an all-region-applicable and continuous power supply MEG that is composed of porous GO and sodium polyacrylate (PAAS) composite. This composite was synthesized by adding PAAS to a GO solution, then reducing the mixture using a directionally controlled laser. The resulting device works well under a wide range of conditions (e.g., temperature between 25°C and 50°C, and RH between 5 and 95%). Moreover, despite its small size (0.1 cm2), the MEG device can continuously deliver a comparatively high open-circuit voltage of 0.6 V for more than 120 h under ambient conditions. Therefore, it has great potential for use in practical applications (Huang et al., 2019).
Qi et al. (Qi et al., 2020) prepared a sponge-based moist-electric generator with a supramolecular assembly of poly (3,4-ethylenedioxythiophene), poly (styrene sulfonate), and graphene oxide by lyophilization. Under extreme thermal fluctuation, this PPGO device can efficiently harvest electric power from thermal evaporation. For example, in the presence of a 110 K temperature difference, the self-powered PPGO generator can supply an open-circuit voltage of up to 2.13 V in moist air (RH = 73 ± 10%), without any mechanical energy input. Overall, the work of Qi et al. provides a means for producing green electric power from moist-electrics and pyroelectrics, and it demonstrates that organic conductive polymer supramolecular assemblies can be used to construct carbon-based sheet composites.
5 Frontier Applications
The most basic function of power generation equipment is to provide electric energy. At present, most MEG devices are used to light LED bulbs (Yang et al., 2019) (Figures 6A,B) and to monitor the relationship between respiratory rate and heart rate after exercise (Zhao et al., 2015) (Figures 6C,D). Han et al. (Han et al., 2020) fabricated a hygroelectric power generator with energy self-storage ability by hybridizing a moist-electric energy harvester with a supercapacitora. In this section, we focus on some unconventional applications of MEG devices.
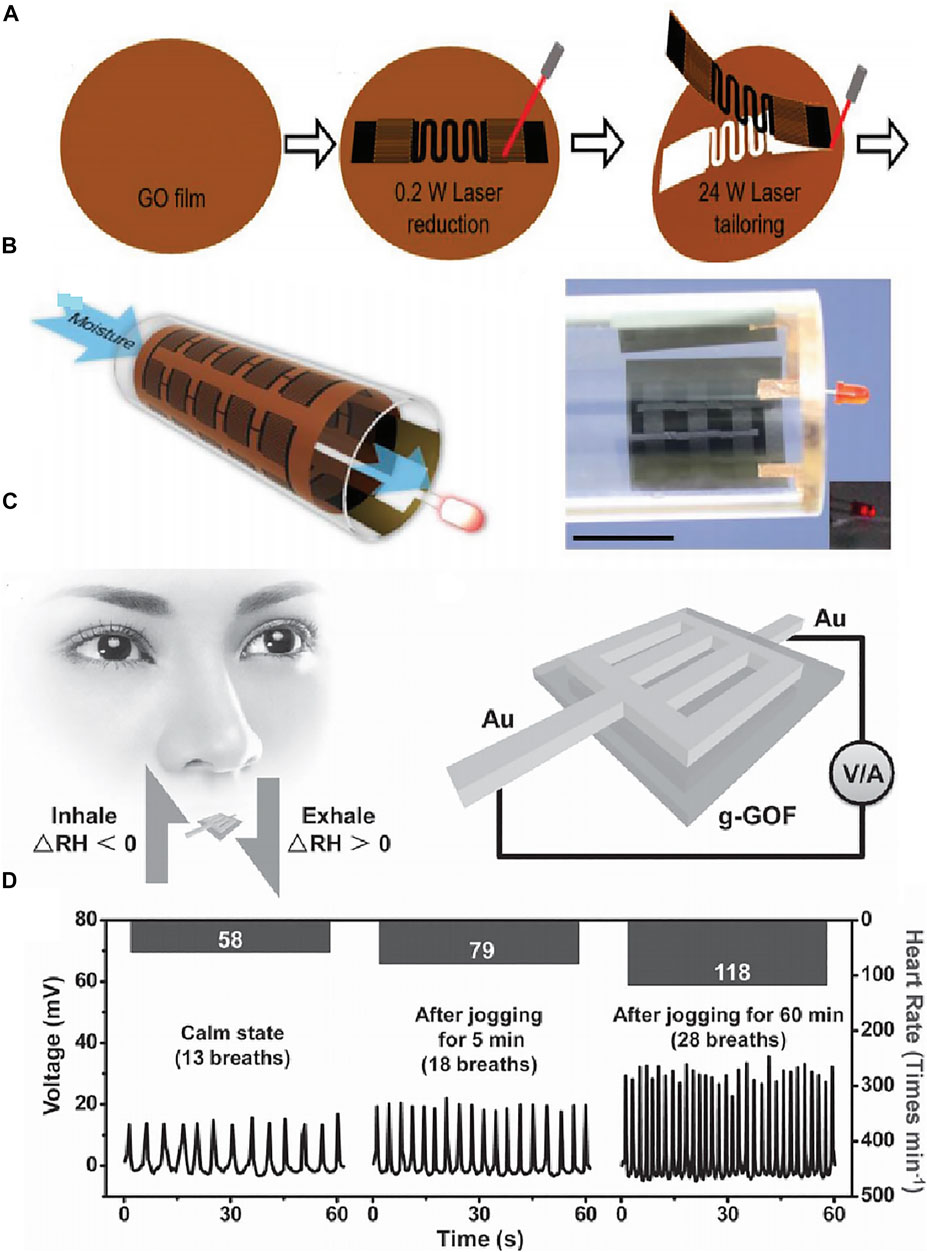
FIGURE 6. (A) Schematic illustration of the process of MEG preparation. The rGO inter digital electrodes and circuits were reduced by direct laser writing, and the freestanding MEG device was fabricated by programmable laser. (B) Scheme and optical images of rolled GO film with multiple GHEG devices powering an LED bulb once a man exhales moisture through the small tube. Scale bar: 20 mm (images reprinted from ref. 83 with permission. Copyright 2019; Wiley Online Library). (C) MEG device that is responsive to RH changes induced by human breathing. To construct the MEG device, the g-GOF is sandwiched between two gold electrodes with vents. (D) Voltage and current outputs generated by a respiratory moisture-tide with ΔRH ≈21% (Figure reprinted from ref. 79 with permission. Copyright 2015; Wiley Online Library).
In natural organisms, ion migration across bio-membranes can induce a bioelectric potential that regulates biochemical processes in organisms. For example, the memory function in humans is achieved by converting the biochemical energy to bioelectric potential in brain neurons (Doyle et al., 1998; Barnett et al., 2001). In recent years, specially designed electronic systems have been successfully used to store information in nonbiological media. Inspired by this knowledge, we developed a novel micro-membrane for self-powered potential switching memory by stacking graphene oxide nanoribbon (GOR) networks (GOR-Ns) (Figures 7A,B). The fabricated prototype of the WORM-type memory exhibits stable and reversible hydration/dehydration cycles and has a remarkably high ON/OFF potential switching ratio. Based on these characteristics, the material can maintain a stable ON/OFF voltage ratio of 106, regardless of whether it is subjected to bending or rolled up into various radii of curvature over 500 cycles. To demonstrate the potential of the prepared micro-membrane in self-powered information reading, a 24-bit GOR-NM-based WORM-chip was constructed. When a person blows the WORM-chip with his/her mouth (Figure 7C,D), the word ‘BIT’ may appear at any time, even 30 days after the writing (Liu et al., 2013).
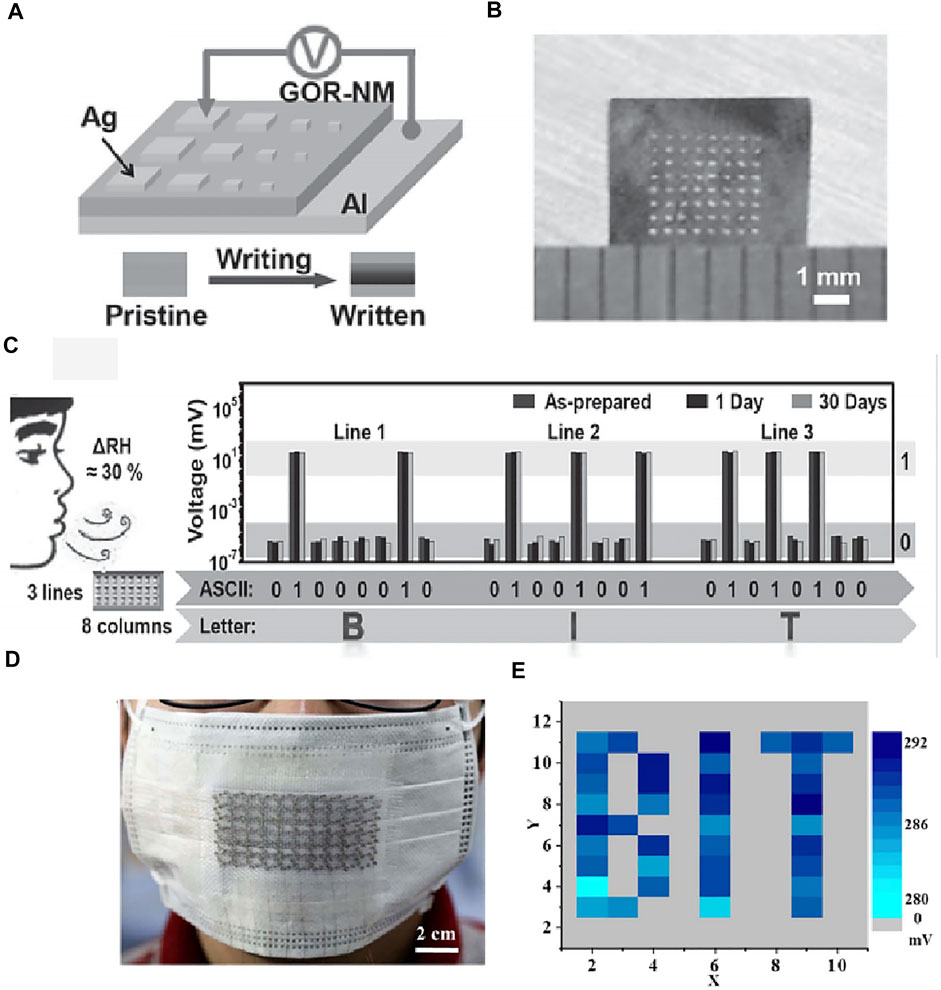
FIGURE 7. (A) Schematic illustration and (B) photograph of the GOR-NM-based memory cell array. (C) Schematic illustration of a WORM-chip prototype with 24 pixels (three lines and eight columns) that can be read by human breathing. Moisture-powered reading of binary codes and corresponding demodulated display for the word ‘BIT’ according to the ASCII. The data can be reliably stored beyond 30 days (Figure reprinted from ref. 21 with permission. Copyright 2016; Wiley Online Library). (D) Image of the device containing 136 GF power generator units attached on a mask. Expression of the electronic label: voltage outputs of all GF power generator units. The voltage outputs are tested after a deep breath (ΔRH = 35%) (image reprinted from ref. 78 with permission. Copyright 2017; Elsevier).
By drawing the graphene fiber power generator into flexible textiles, Liang et al. (Hu et al., 2013) were able to achieve information expression. In total, 136 information units were woven into a piece of 3 cm × 7 cm fabric (Figure 7D). Considering that each unit can have the value of “0” or “1” the fabric can convey 2136 kinds of different information. Moreover, due to its high mechanical flexibility, the fabric can serve as a novel wearable electronic label that can be attached onto a mask. The constant moisture tide provided by human breathing contributes to information expression. The output voltage of the device varies depending on the area where people take a deep breath, and as shown in Figure 7E, the three letters of ‘BIT’ are successfully displayed.
6 Conclusion and Perspectives
In conclusion, this review summarizes the different techniques used to prepare graphene assemblies and discusses recent developments in the field of MEG. Overall, it is shown that the design of assemblies from graphene sheets rationally constitutes a promising strategy for the development of MEG devices. Continuous research efforts have succeeded in increasing the output voltage of these devices from 35 mV in 2015 to more than 2 V at present (Table 1).
Although the field of MEG has progressed rapidly in the past few years, the large-scale application of MEG devices is limited by many challenges. First, the current output voltage and power density of these devices are too small to meet the needs of practical production, moreover, the time for stable continuous output is not long enough. Second, the generation of power by interaction between water molecules and graphene is a complex process whose mechanism is not completely clear. Third, the research results obtained in the laboratory cannot be easily reproduced in application due to the changeability of the natural environment. Therefore, the service life of the MEG device cannot be predicted. Finally, to meet the needs of practical application, the designed device must perform well under different conditions and scenarios. Despite these challenges, graphene has shown great potential for use in MEG. We believe that with the joint efforts of researchers from different professional disciplines, graphene-based MEG can be rapidly developed and applied in real life.
Author Contributions
QC completed the writing, and JZ participated in part of the writing. HC provided guidance for the paper.
Conflict of Interest
The authors declare that the research was conducted in the absence of any commercial or financial relationships that could be construed as a potential conflict of interest.
Publisher’s Note
All claims expressed in this article are solely those of the authors and do not necessarily represent those of their affiliated organizations, or those of the publisher, the editors and the reviewers. Any product that may be evaluated in this article, or claim that may be made by its manufacturer, is not guaranteed or endorsed by the publisher.
Acknowledgments
This work was supported by the National Key Research and Development Program of China (2019YFD1100200).
References
Bai, J., Huang, Y., Cheng, H., and Qu, L. (2019). Moist-Electric Generation. Nanoscale 11, 23083–23091. doi:10.1039/c9nr06113d
Barnett, R. N., Cleveland, C. L., Joy, A., Landman, U., and Schuster, G. B. (2001). Charge Migration in DNA: Ion-Gated Transport. Science 294, 567–571. doi:10.1126/science.1062864
Chan, S.-H., Chen, S.-H., Lin, W.-T., Li, M.-C., Lin, Y.-C., and Kuo, C.-C. (2013). Low-Temperature Synthesis of Graphene on Cu Using Plasma-Assisted Thermal Chemical Vapor Deposition. Nanoscale Res. Lett. 8, 285. doi:10.1186/1556-276x-8-285
Chen, T., and Dai, L. (2015). Macroscopic Graphene Fibers Directly Assembled from CVD‐Grown Fiber‐Shaped Hollow Graphene Tubes. Angew. Chem. Int. Ed. 54, 14947–14950. doi:10.1002/anie.201507246
Chen, Z., Xu, C., Ma, C., Ren, W., and Cheng, H.-M. (2013). Lightweight and Flexible Graphene Foam Composites for High-Performance Electromagnetic Interference Shielding. Adv. Mater. 25, 1296–1300. doi:10.1002/adma.201204196
Chen, Q., Hu, Y., Hu, C., Cheng, H., Zhang, Z., Shao, H., et al. (2014). Graphene Quantum Dots-Three-Dimensional Graphene Composites for High-Performance Supercapacitors. Phys. Chem. Chem. Phys. 16, 19307–19313. doi:10.1039/c4cp02761b
Chen, Q., Zhao, Y., Huang, X., Chen, N., and Qu, L. (2015). Three-Dimensional Graphitic Carbon Nitride Functionalized Graphene-Based High-Performance Supercapacitors. J. Mater. Chem. A. 3, 6761–6766. doi:10.1039/c5ta00734h
Chen, N., Liu, Q., Liu, C., Zhang, G., Jing, J., Shao, C., et al. (2019). MEG Actualized by High-Valent Metal Carrier Transport. Nano Energy 65, 104047. doi:10.1016/j.nanoen.2019.104047
Chen, J., Wang, Y., Cao, J., Liao, L., Liu, Y., Zhou, Y., et al. (2020). Pulsed Electrochemical Fabrication of Graphene/Polypyrrole Composite Gel Films for High Performance and Flexible Supercapacitors. Electrochimica Acta 361, 137036. doi:10.1016/j.electacta.2020.137036
Chen, Q., Zhao, J., Cheng, H. H., and Qu, L. T. (2021). Progress in 3D-Graphene Assemblies Preparation for Solar-Thermal Steam Generation and Water Treatment. Acta Phys. -Chim. Sin. 38, 2101020. doi:10.3866/PKU.WHXB202101020
Cheng, H., Liu, J., Zhao, Y., Hu, C., Zhang, Z., Chen, N., et al. (2013). Graphene Fibers with Predetermined Deformation as Moisture-Triggered Actuators and Robots. Angew. Chem. Int. Ed. 52, 10482–10486. doi:10.1002/anie.201304358
Cheng, H., Huang, Y., Shi, G., Jiang, L., and Qu, L. (2017). Graphene-Based Functional Architectures: Sheets Regulation and Macrostructure Construction toward Actuators and Power Generators. Acc. Chem. Res. 50, 1663–1671. doi:10.1021/acs.accounts.7b00131
Cheng, H., Huang, Y., Qu, L., Cheng, Q., Shi, G., and Jiang, L. (2018). Flexible In-Plane Graphene Oxide Moisture-Electric Converter for Touchless Interactive Panel. Nano Energy 45, 37–43. doi:10.1016/j.nanoen.2017.12.033
Cheng, H., Huang, Y., Zhao, F., Yang, C., Zhang, P., Jiang, L., et al. (2018). Spontaneous Power Source in Ambient Air of a Well-Directionally Reduced Graphene Oxide Bulk. Energy Environ. Sci. 11, 2839–2845. doi:10.1039/c8ee01502c
Chu, S., Cui, Y., and Liu, N. (2017). The Path towards Sustainable Energy. Nat. Mater 16, 16–22. doi:10.1038/nmat4834
Chu, X., Huang, T., Hu, Y., Dong, R., Luo, J., Cai, S., et al. (2020). Wet-Spinning Assembly of Nitrogen-Doped Graphene Film for Stable Graphene-Polyaniline Supercapacitor Electrodes with High Mass Loading. Sci. China Mater. 63, 1889–1897. doi:10.1007/s40843-019-9436-1
Cong, H.-P., Ren, X.-C., Wang, P., and Yu, S.-H. (2012). Wet-Spinning Assembly of Continuous, Neat and Macroscopic Graphene Fibers. Sci. Rep. 2, 613. doi:10.1038/srep00613
David, A. K. (2004). Climate Change Science: Adapt, Mitigate, or Ignore? Science 303, 176–177. doi:10.1126/science.1094329
Dong, Z., Jiang, C., Cheng, H., Zhao, Y., Shi, G., Jiang, L., et al. (2012). Facile Fabrication of Light, Flexible and Multifunctional Graphene Fibers. Adv. Mater. 24, 1856–1861. doi:10.1002/adma.201200170
Dong, Y., Zhang, T., Lin, X., Feng, J., and He, Q. (2020). Graphene/Aptamer Probes for Small Molecule Detection: from In Vitro Test to In Situ Imaging. Microchim. Acta 187, 179. doi:10.1007/s00604-020-4128-8
Doyle, D. A., Cabral, J. M., Pfuetzner, R. A., Kuo, A., Gulbis, J. M., Cohen, S. L., et al. (1998). The Structure of the Potassium Channel: Molecular Basis of K + Conduction and Selectivity. Science 280, 69–77. doi:10.1126/science.280.5360.69
El-Kady, M. F., Strong, V., Dubin, S., and Kaner, R. B. (2012). Laser Scribing of High-Performance and Flexible Graphene-Based Electrochemical Capacitors. Science 335, 1326–1330. doi:10.1126/science.1216744
Ervin, M. H. (2015). Etching Holes in Graphene Supercapacitor Electrodes for Faster Performance. Nanotechnology 26, 234003–234012. doi:10.1088/0957-4484/26/23/234003
Fan, F. R., Tang, W., and Wang, Z. L. (2016). Flexible Nanogenerators for Energy Harvesting and Self-Powered Electronics. Adv. Mater. 28, 4283–4305. doi:10.1002/adma.201504299
Fei, W., Shen, C., Zhang, S., Chen, H., Li, L., and Guo, W. (2019). Waving Potential at Volt Level by a Pair of Graphene Sheets. Nano Energy 60, 656–660. doi:10.1016/j.nanoen.2019.04.020
Fu, K., Wang, Y., Yan, C., Yao, Y., Chen, Y., Dai, J., et al. (2016). Graphene Oxide-Based Electrode Inks for 3D-Printed Lithium-Ion Batteries. Adv. Mater. 28, 2587–2594. doi:10.1002/adma.201505391
Gao, X., Xu, T., Shao, C., Han, Y., Lu, B., Zhang, Z., et al. (2019). Electric Power Generation Using Paper Materials. J. Mater. Chem. A. 7, 20574–20578. doi:10.1039/c9ta08264f
Geim, A. K., and Novoselov, K. S. (2007). The Rise of Graphene. Nat. Mater 6, 183–191. doi:10.1038/nmat1849
Geim, A. K. (2009). Graphene: Status and Prospects. Science 324, 1530–1534. doi:10.1126/science.1158877
Han, Y., Zhang, Z., and Qu, L. (2019). Power Generation from Graphene-Water Interactions. FlatChem 14, 100090. doi:10.1016/j.flatc.2019.100090
Han, Y., Lu, B., Shao, C., Xu, T., Liu, Q., Liang, Y., et al. (2020). A Hygroelectric Power Generator with Energy Self-Storage. Chem. Eng. J. 384, 123366. doi:10.1016/j.cej.2019.123366
Han, Z.-Y., Huang, L.-J., Qu, H.-J., Wang, Y.-X., Zhang, Z.-J., Rong, Q.-L., et al. (2021). A Review of Performance Improvement Strategies for Graphene Oxide-Based and Graphene-Based Membranes in Water Treatment. J. Mater. Sci. 56, 9545–9574. doi:10.1007/s10853-021-05873-7
He, H., Zhao, T., Guan, H., Zhong, T., Zeng, H., Xing, L., et al. (2019). A Water-Evaporation-Induced Self-Charging Hybrid Power Unit for Application in the Internet of Things. Sci. Bull. 64, 1409–1417. doi:10.1016/j.scib.2019.06.020
He, P., Wu, J., Pan, X., Chen, L., Liu, K., Gao, H., et al. (2020). Anti-Freezing and Moisturizing Conductive Hydrogels for Strain Sensing and Moist-Electric Generation Applications. J. Mater. Chem. A. 8, 3109–3118. doi:10.1039/c9ta12940e
Hou, B., Kong, D., Qian, J., Yu, Y., Cui, Z., Liu, X., et al. (2018). Flexible and Portable Graphene on Carbon Cloth as a Power Generator for Electricity Generation. Carbon 140, 488–493. doi:10.1016/j.carbon.2018.09.005
Hu, C., Zhai, X., Liu, L., Zhao, Y., Jiang, L., and Qu, L. (2013). Spontaneous Reduction and Assembly of Graphene Oxide into Three-Dimensional Graphene Network on Arbitrary Conductive Substrates. Sci. Rep. 3, 2065. doi:10.1038/srep02065
Hu, Q., Liu, X., Zhu, B., Fan, L., Chai, X., Zhang, Q., et al. (2018). Crafting MoC2-Doped Bimetallic alloy Nanoparticles Encapsulated within N-Doped Graphene as Roust Bifunctional Electrocatalysts for Overall Water Splitting. Nano Energy 50, 212–219. doi:10.1016/j.nanoen.2018.05.033
Hu, L., Sun, W., Tang, Y., Li, S., Zhang, B., Sun, X., et al. (2021). Photothermal Effect Enhancing Graphene Quantum Dots/semiconducting Polymer/Nanozyme-Mediated Cancer Catalytic Therapy. Carbon 176, 148–156. doi:10.1016/j.carbon.2021.01.132
Huang, X., Sun, B., Su, D., Zhao, D., and Wang, G. (2014). Soft-Template Synthesis of 3D Porous Graphene Foams with Tunable Architectures for Lithium-O2 Batteries and Oil Adsorption Applications. J. Mater. Chem. A. 2, 7973–7979. doi:10.1039/c4ta00829d
Huang, Y., Cheng, H., Shi, G., and Qu, L. (2017). Highly Efficient Moisture-Triggered Nanogenerator Based on Graphene Quantum Dots. ACS Appl. Mater. Inter. 9, 38170–38175. doi:10.1021/acsami.7b12542
Huang, Y., Cheng, H., Yang, C., Zhang, P., Liao, Q., Yao, H., et al. (2018). Interface-Mediated Hygroelectric Generator with an Output Voltage Approaching 1.5 Volts. Nat. Commun. 9, 4166. doi:10.1038/s41467-018-06633-z
Huang, Y., Cheng, H., Yang, C., Yao, H., Li, C., and Qu, L. (2019). All-Region-Applicable, Continuous Power Supply of Graphene Oxide Composite. Energ. Environ. Sci. 12, 1848–1856. doi:10.1039/c9ee00838a
Jin, Q., Jiang, S., Zhao, Y., Wang, D., Qiu, J., Tang, D.-M., et al. (2019). Flexible Layer-Structured Bi2Te3 Thermoelectric on a Carbon Nanotube Scaffold. Nat. Mater 18, 62–68. doi:10.1038/s41563-018-0217-z
Jung, E. H., Jeon, N. J., Park, E. Y., Moon, C. S., Shin, T. J., Yang, T.-Y., et al. (2019). Efficient, Stable and Scalable Perovskite Solar Cells Using Poly(3-Hexylthiophene). Nature 567, 511–515. doi:10.1038/s41586-019-1036-3
Kuriya, K., Ochiai, K., Kalita, G., Tanemura, M., Komiya, A., Kikugawa, G., et al. (2020). Output Density Quantification of Electricity Generation by Flowing Deionized Water on Graphene. Appl. Phys. Lett. 117, 123905. doi:10.1063/5.0018862
Lee, C., Wei, X., Kysar, J. W., and Hone, J. (2008). Measurement of the Elastic Properties and Intrinsic Strength of Monolayer Graphene. Science 321, 385–388. doi:10.1126/science.1157996
Li, Y., Zhao, Y., Cheng, H., Hu, Y., Shi, G., Dai, L., et al. (2012). Nitrogen-Doped Graphene Quantum Dots with Oxygen-Rich Functional Groups. J. Am. Chem. Soc. 134, 15–18. doi:10.1021/ja206030c
Liang, Y., Zhao, F., Cheng, Z., Zhou, Q., Shao, H., Jiang, L., et al. (2017). Self-Powered Wearable Graphene Fiber for Information Expression. Nano Energy 32, 329–335. doi:10.1016/j.nanoen.2016.12.062
Liang, Y., Zhao, F., Cheng, Z., Deng, Y., Xiao, Y., Cheng, H., et al. (2018). Electric Power Generation via Asymmetric Moisturizing of Graphene Oxide for Flexible, Printable and Portable Electronics. Energ. Environ. Sci. 11, 1730–1735. doi:10.1039/c8ee00671g
Liu, J., Yin, Z., Cao, X., Zhao, F., Wang, L., Huang, W., et al. (2013). Fabrication of Flexible, All-Reduced Graphene Oxide Non-Volatile Memory Devices. Adv. Mater. 25, 233–238. doi:10.1002/adma.201203349
Liu, K., Yang, P., Li, S., Li, J., Ding, T., Xue, G., et al. (2016). Induced Potential in Porous Carbon Films Through Water Vapor Absorption. Angew. Chem. Int. Ed. Engl. 55, 8003–8007. doi:10.1002/anie.201602708
Liu, X., Gao, H., Ward, J. E., Liu, X., Yin, B., Fu, T., et al. (2020). Power Generation from Ambient Humidity Using Protein Nanowires. Nature 578, 550–554. doi:10.1038/s41586-020-2010-9
Luo, Z., Liu, C., and Fan, S. (2019). A Moisture Induced Self-Charging Device for Energy Harvesting and Storage. Nano Energy 60, 371–376. doi:10.1016/j.nanoen.2019.03.073
Meng, X., Yu, C., Song, X., Iocozzia, J., Hong, J., Rager, M., et al. (2018). Scrutinizing Defects and Defect Density of Selenium‐Doped Graphene for High‐Efficiency Triiodide Reduction in Dye‐Sensitized Solar Cells. Angew. Chem. Int. Ed. 57, 4682–4686. doi:10.1002/anie.201801337
Nie, X., Ji, B., Chen, N., Liang, Y., Han, Q., and Qu, L. (2018). Gradient Doped Polymer Nanowire for Moistelectric Nanogenerator. Nano Energy 46, 297–304. doi:10.1016/j.nanoen.2018.02.012
Ponnamma, D., Yin, Y. F., Salim, N., Parameswaranpillai, J., Thomas, S., and Hamee, N. (2021). Recent Progress and Multifunctional Applications of 3D Printed Graphene Nanocomposites. Compos. B. Eng. 204, 108493. doi:10.1016/j.compositesb.2020.108493
Ponomarenko, L. A., Schedin, F., Katsnelson, M. I., Yang, R., Hill, E. W., Novoselov, K. S., et al. (2008). Chaotic Dirac Billiard in Graphene Quantum Dots. Science 320, 356–358. doi:10.1126/science.1154663
Qi, X., Miao, T., Chi, C., Zhang, G., Zhang, C., Du, Y., et al. (2020). Ultralight PEDOT:PSS/Graphene Oxide Composite Aerogel Sponges for Electric Power Harvesting from Thermal Fluctuations and Moist Environment. Nano Energy 77, 105096. doi:10.1016/j.nanoen.2020.105096
Qin, Y., Wang, Y., Sun, X., Li, Y., Xu, H., Tan, Y., et al. (2020). Constant Electricity Generation in Nanostructured Silicon by Evaporation‐Driven Water Flow. Angew. Chem. Int. Ed. 59, 10619–10625. doi:10.1002/anie.202002762
Qin, B., Ma, H., Hossain, M., Zhong, M., Xia, Q., Li, B., et al. (2020). Substrates in the Synthesis of Two-Dimensional Materials via Chemical Vapor Deposition. Chem. Mater. 32, 10321–10347. doi:10.1021/acs.chemmater.0c03549
Shen, D., Duley, W. W., Peng, P., Xiao, M., Feng, J., Liu, L., et al. (2020). Moisture‐Enabled Electricity Generation: From Physics and Materials to Self‐Powered Applications. Adv. Mater. 32, 2003722. doi:10.1002/adma.202003722
Shi, L., Chen, K., Du, R., Bachmatiuk, A., Rümmeli, M. H., Xie, K., et al. (2016). Scalable Seashell-Based Chemical Vapor Deposition Growth of Three-Dimensional Graphene Foams for Oil-Water Separation. J. Am. Chem. Soc. 138, 6360–6363. doi:10.1021/jacs.6b02262
Somani, P. R., Somani, S. P., and Umeno, M. (2006). Planer Nano-Graphenes from Camphor by CVD. Chem. Phys. Lett. 430, 56–59. doi:10.1016/j.cplett.2006.06.081
Stankovich, S., Dikin, D. A., Dommett, G. H. B., Kohlhaas, K. M., Zimney, E. J., Stach, E. A., et al. (2006). Graphene-Based Composite Materials. Nature 442, 282–286. doi:10.1038/nature04969
Steven, C., and Arun, M. (2012). Opportunities and Challenges for a Sustainable Energy Future. Nature 488, 294–303. doi:10.1038/nature11475
Strong, V., Dubin, S., El-Kady, M. F., Lech, A., Wang, Y., Weiller, B. H., et al. (2012). Patterning and Electronic Tuning of Laser Scribed Graphene for Flexible All-Carbon Devices. ACS Nano 6 (2), 1395–1403. doi:10.1021/nn204200w
Sun, Z., Feng, L., Xiong, C., He, X., Wang, L., Qin, X., et al. (2021). Electrospun Nanofiber Fabric: an Efficient, Breathable and Wearable Moist-Electric Generator. J. Mater. Chem. A. 9, 7085–7093. doi:10.1039/d0ta11974a
Tang, G., Jiang, Z.-G., Li, X., Zhang, H.-B., Dasari, A., and Yu, Z.-Z. (2014). Three Dimensional Graphene Aerogels and Their Electrically Conductive Composites. Carbon 77, 592–599. doi:10.1016/j.carbon.2014.05.063
Them, N. C. Y., Sahoo, P. K., Kim, Y., Hegde, C., and Murugesan, V. M. (2021). Thermally Controlled Localized Porous Graphene for Integrated Graphene Paper Electronics. Adv. Mater. Tech. 6, 2001156. doi:10.1002/admt.202001156
Thomas, T., and Agarwal, A. (2021). A Facile and Scalable Approach in the Fabrication of Tailored 3D Graphene Foam via Freeze Drying. Materials 14, 864. doi:10.3390/ma14040864
Tynan, M. K., Johnson, D. W., Dobson, B. P., and Coleman, K. S. (2016). Formation of 3D Graphene Foams on Soft Templated Metal Monoliths. Nanoscale 8, 13303–13310. doi:10.1039/c6nr02455f
van der Heyden, F. H. J., Stein, D., and Dekker, C. (2005). Streaming Currents in a Single Nanofluidic Channel. Phys. Rev. Lett. 95, 116104. doi:10.1103/physrevlett.95.116104
Wang, G., Zhang, M., Zhu, Y., Ding, G., Jiang, D., Guo, Q., et al. (2013). Direct Growth of Graphene Film on Germanium Substrate. Sci. Rep. 3, 2465. doi:10.1038/srep02465
Wang, M., Jang, S. K., Jang, W.-J., Kim, M., Park, S.-Y., Kim, S.-W., et al. (2013). A Platform for Large-Scale Graphene Electronics - CVD Growth of Single-Layer Graphene on CVD-Grown Hexagonal Boron Nitride. Adv. Mater. 25, 2746–2752. doi:10.1002/adma.201204904
Wang, H., Cheng, H., Huang, Y., Yang, C., Wang, D., Li, C., et al. (2020). Transparent, Self-Healing, Arbitrary Tailorable Moist-Electric Film Generator. Nano Energy 67, 104238. doi:10.1016/j.nanoen.2019.104238
Wang, H. Y., Sun, Y. L., He, T. C., Huang, Y. X., Cheng, H. H., Li, C., et al. (2021). Bilayer of Polyelectrolyte Films for Spontaneous Power Generation in Air up to an Integrated 1,000Voutput. Nat. .Nanothchol 16, 811–819. doi:10.1038/s41565-021-00903-6
Wei, X., Li, D., Jiang, W., Gu, Z., Wang, X., Zhang, Z., et al. (2015). 3D Printable Graphene Composite. Sci. Rep. 5, 11181. doi:10.1038/srep11181
Xie, H., Zhang, J., and Mu, H. (2020). Fast Growth of Continuous Single-Crystal Graphene Film on Copper by Low-Pressure Chemical Vapor Deposition. J. Elec Materi 49, 5064–5069. doi:10.1007/s11664-020-08235-2
Xin, G., Yao, T., Sun, H., Scott, S. M., Shao, D., Wang, G., et al. (2016). Highly Thermally Conductive and Mechanically strong Graphene Fibers. Science 349, 1083–1087. doi:10.1126/science.aaa6502
Xu, Z., Sun, H., Zhao, X., and Gao, C. (2013). Ultrastrong Fibers Assembled from Giant Graphene Oxide Sheets. Adv. Mater. 25, 188–193. doi:10.1002/adma.201203448
Xu, X., Zhang, Q., Yu, Y., Chen, W., Hu, H., and Li, H. (2016). Naturally Dried Graphene Aerogels with Superelasticity and Tunable Poisson's Ratio. Adv. Mater. 28, 9223–9230. doi:10.1002/adma.201603079
Xu, T., Han, Q., Cheng, Z., Zhang, J., and Qu, L. (2018). Interactions between Graphene-Based Materials and Water Molecules toward Actuator and Electricity-Generator Applications. Small Methods 2, 1800108. doi:10.1002/smtd.201800108
Xu, T., Ding, X., Shao, C., Song, L., Lin, T., Gao, X., et al. (2018). Electric Power Generation through the Direct Interaction of Pristine Graphene-Oxide with Water Molecules. Small 14, 1704473. doi:10.1002/smll.201704473
Xu, T., Ding, X., Huang, Y., Shao, C., Song, L., Gao, X., et al. (2019). An Efficient Polymer Moist-Electric Generator. Energ. Environ. Sci. 12, 972–978. doi:10.1039/c9ee00252a
Xue, G., Xu, Y., Ding, T., Li, J., Yin, J., Fei, W., et al. (2017). Water-Evaporation-Induced Electricity with Nanostructured Carbon Materials. Nat. Nanotech 12, 317–321. doi:10.1038/nnano.2016.300
Yan, X., Li, B., and Li, L.-s. (2013). Colloidal Graphene Quantum Dots with Well-Defined Structures. Acc. Chem. Res. 46, 2254–2262. doi:10.1021/ar300137p
Yan, J., Ding, Y., Hu, C., Cheng, H., Chen, N., Feng, Z., et al. (2014). Preparation of Multifunctional Microchannel-Network Graphene Foams. J. Mater. Chem. A. 2, 16786–16792. doi:10.1039/c4ta03057e
Yang, Y., Han, C., Jiang, B., Iocozzia, J., He, C., Shi, D., et al. (2016). Graphene-Based Materials with Tailored Nanostructures for Energy Conversion and Storage. Mater. Sci. Eng. R: Rep. 102, 1–72. doi:10.1016/j.mser.2015.12.003
Yang, H., Li, Z., Lu, B., Gao, J., Jin, X., Sun, G. Q., et al. (2018). Reconstruction of Inherent Graphene Oxide Liquid Crystals for Large-Scale Fabrication of Structure-Intact Graphene Aerogel Bulk toward Practical Applications. ACS Nano 12, 11407–11416. doi:10.1021/acsnano.8b06380
Yang, J., Weng, W., Zhang, Y., Du, X., Liang, Y., Yang, L., et al. (2018). Highly Flexible and Shape-Persistent Graphene Microtube and its Application in Supercapacitor. Carbon 126, 419–425. doi:10.1016/j.carbon.2017.10.045
Yang, C., Huang, Y., Cheng, H., Jiang, L., and Qu, L. (2019). Rollable, Stretchable, and Reconfigurable Graphene Hygroelectric Generators. Adv. Mater. 31, 1805705. doi:10.1002/adma.201805705
Yao, Y., Lv, T., Chen, Z., Li, H., Li, N., Yang, Y., et al. (2020). High‐Performance Tubular Electricity Generators Operated by Magnetically Driving Movement of Droplet. Adv. Mater. Inter. 7, 2001592. doi:10.1002/admi.202001592
Yin, J., Zhang, Z., Li, X., Yu, J., Zhou, J., Chen, Y., et al. (2014). Waving Potential in Graphene. Nat. Commun. 5, 3582. doi:10.1038/ncomms4582
Yin, J., Li, X., Yu, J., Zhang, Z., Zhou, J., and Guo, W. (2014). Generating Electricity by Moving a Droplet of Ionic Liquid along Graphene. Nat. Nanotech 9, 378–383. doi:10.1038/nnano.2014.56
Zeng, J., Ji, X., Ma, Y., Zhang, Z., Wang, S., Ren, Z., et al. (2018). 3D Graphene Fibers Grown by Thermal Chemical Vapor Deposition. Adv. Mater. 30, 1705380. doi:10.1002/adma.201705380
Zhang, G., Duan, Z., Qi, X., Xu, Y., Li, L., Ma, W., et al. (2019). Harvesting Environment Energy from Water-Evaporation over Free-Standing Graphene Oxide Sponges. Carbon 148, 1–8. doi:10.1016/j.carbon.2019.03.041
Zhao, Y., Song, L., Deng, K., Liu, Z., Zhang, Z., Yang, Y., et al. (2008). Individual Water-Filled Single-Walled Carbon Nanotubes as Hydroelectric Power Converters. Adv. Mater. 20, 1772–1776. doi:10.1002/adma.200702956
Zhao, Y., Hu, C., Hu, Y., Cheng, H., Shi, G., Qu, L., et al. (2012). A Versatile, Ultralight, Nitrogen-Doped Graphene Framework. Angew. Chem. 124, 11533–11537. doi:10.1002/ange.201206554
Zhao, F., Cheng, H., Zhang, Z., Jiang, L., and Qu, L. (2015). Direct Power Generation from a Graphene Oxide Film under Moisture. Adv. Mater. 27, 4351–4357. doi:10.1002/adma.201501867
Zhao, M., Li, X., Song, L., He, D., and Zhang, Z. (2016). Substrate-Assisted Deposition of Metal Oxides on Three-Dimensional Porous Reduced Graphene Oxide Networks as Bifunctional Hybrid Electrocatalysts for the Oxygen Evolution and Oxygen Reduction Reactions. Chem Cat Chem 8, 2808–2816. doi:10.1002/cctc.201600562
Zhao, F., Liang, Y., Cheng, H., Jiang, L., and Qu, L. (2016). Highly Efficient Moisture-Enabled Electricity Generation from Graphene Oxide Frameworks. Energ. Environ. Sci. 9, 912–916. doi:10.1039/c5ee03701h
Zhao, F., Wang, L., Zhao, Y., Qu, L., and Dai, L. (2017). Graphene Oxide Nanoribbon Assembly toward Moisture-Powered Information Storage. Adv. Mater. 29, 1604972. doi:10.1002/adma.201604972
Zhao, X., Yao, W., Gao, W., Chen, H., and Gao, C. (2017). Wet-Spun Superelastic Graphene Aerogel Millispheres with Group Effect. Adv. Mater. 29, 1701482. doi:10.1002/adma.201701482
Zhou, Q., Hui, Z., Xiao, M., and Zhou, N. Y. (2020). A Highly Sensitive Double-Layer Structured Nanodevice for Moisture Induced Power Generation. Nanotechnology 31, 265401. doi:10.1088/1361-6528/ab7fcd
Keywords: graphene assemblies, moisture, electric generation, foam, film
Citation: Chen Q, Zhao J and Cheng H (2021) Graphene-Based Assemblies for Moist-Electric Generation. Front. Energy Res. 9:738142. doi: 10.3389/fenrg.2021.738142
Received: 07 August 2021; Accepted: 08 November 2021;
Published: 13 December 2021.
Edited by:
Wei-Ren Liu, Chung Yuan Christian University, TaiwanReviewed by:
Shao Changxiang, Beijing Institute of Technology, ChinaYongqi Zhang, University of Electronic Science and Technology of China, China
Copyright © 2021 Chen, Zhao and Cheng. This is an open-access article distributed under the terms of the Creative Commons Attribution License (CC BY). The use, distribution or reproduction in other forums is permitted, provided the original author(s) and the copyright owner(s) are credited and that the original publication in this journal is cited, in accordance with accepted academic practice. No use, distribution or reproduction is permitted which does not comply with these terms.
*Correspondence: Qing Chen, Y2hlbnFpbmc5ODIxQDE2My5jb20=, Huhu Cheng, aHVodWNoZW5nQHRzaW5naHVhLmVkdS5jbg==