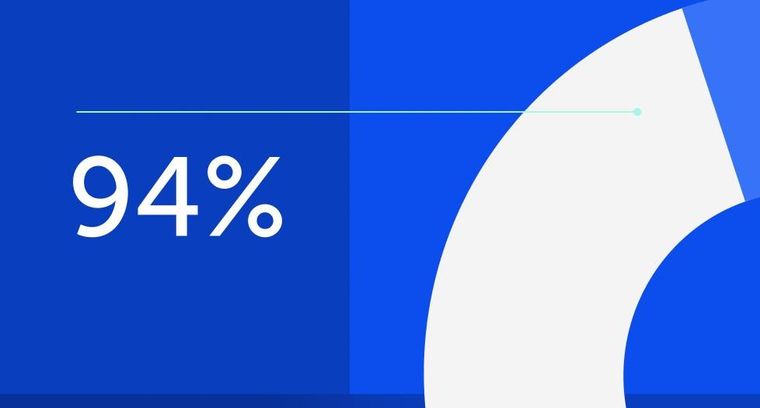
94% of researchers rate our articles as excellent or good
Learn more about the work of our research integrity team to safeguard the quality of each article we publish.
Find out more
REVIEW article
Front. Energy Res., 25 August 2021
Sec. Bioenergy and Biofuels
Volume 9 - 2021 | https://doi.org/10.3389/fenrg.2021.735141
This article is part of the Research TopicIntegrated Waste Biorefineries: Achieving Sustainable Development GoalsView all 11 articles
Microalgae offer a great potential to contribute significantly as renewable fuels and documented as a promising platform for algae-based bio refineries. They provide solutions to mitigate the environmental concerns posed by conventional fuel sources; however, the production of microalgal biofuels in large scale production system encounters few technical challenges. High quantity of nutrients requirements and water cost constrain the scaling up microalgal biomass to large scale commercial production. Crop protection against biomass losses due to grazers or pathogens is another stumbling block in microalgal field cultivation. With our existing technologies, unless coupled with high-value or mid-value products, algal biofuel cannot reach the economic target. Many microalgal industries that started targeting biofuel in the last decade had now adopted parallel business plans focusing on algae by-products application as cosmetic supplements, nutraceuticals, oils, natural color, and animal feed. This review provides the current status and proposes a framework for key supply demand, challenges for cost-effective and sustainable use of water and nutrient. Emphasis is placed on the future industrial market status of value added by products of microalgal biomass. The cost factor for biorefinery process development needs to be addressed before its potential to be exploited for various value-added products with algal biofuel.
The development of renewable energy resources has become a priority due to climate change and dwindling fossil fuel reserves. Algae holds much promise as a potential feedstock for biofuels because of their higher capacity for productivity per unit land area than conventional terrestrial feedstocks (Chisti, 2007; Wijffels et al., 2010; Georgianna and Mayfield, 2012; Khrunyk et al., 2020). Algal biofuel is immediately compatible with our existing transportation infrastructures like refineries, fuel stations, and the engines of cars (Hannon et al., 2010). If a profitable and sustainable algal biofuel process can be developed, the potential benefits of the technology are compelling including the use of non-arable land, recycling wastewater, and carbon dioxide.
An extensive research program on algal biofuels was sponsored more than 40 years ago by the US Department of Energy (DoE) at the National Renewable Energy Laboratory during the oil crisis of the 1970s. Despite being a successful demonstration of the feasibility of algal biomass as a source of oil, this Aquatic Species Program (1978–1996) was discontinued due to the decreasing federal budget and lower crude oil market (Hu et al., 2008). In the last decade, microalgae reemerged as a source of biofuel and concerted effort has been made towards isolating potential microalgal strain, strain improvement, elucidating biosynthesis pathways, optimize growth and cultivation parameter, harvesting, coproduct development, fuel extraction, refining and residual biomass utilization (Garrido-Cardenas et al., 2018; Wood, 2021). Like academic research, huge claims about the promise of algal biofuel (Chisti, 2007) and the high crude oil price at that time motivated a large number of companies to take an interest in microalgal biofuel, investing significant amounts of money to pursue that objective. Recent technoeconomic analysis has demonstrated that with existing technological readiness, algal biofuel is cost competitive with fossil fuel if combined with the production of high-value co-products (Ruiz et al., 2016; Cruce and Quinn, 2019). Today, most algae based companies have adopted parallel business plans that focus more on the expensive algae byproducts such as cosmetic supplements, nutraceuticals, specialty oils, natural color, and animal feed.
In this review, we provide a perspective on developing sustainable algal cultivation practices and bioproducts from microalgae to make the process of algal biofuel efficient and economically competitive. We have restricted this review to the photoautotrophic microalgal biomass production and to process them into biodiesel or converting them into biocrude at high temperature and pressure through hydrothermal liquefaction but does not include any other form of algal biofuel like bioethanol, biogas, or biohydrogen. The worldwide commercial production of microalgal products is also discussed.
Large capital investment in microalgae cultivation still limits economic biomass production (Acién et al., 2012; Ruiz et al., 2016; Kaur Nagi et al., 2021). Microalgae are not growing at a scale (<5 ha) that is required for the level of cost economy analysis of biofuels. To make “high-volume, low-cost” product like biofuel, microalgal production system must be increased several orders of magnitude, and it demand strategies to reduce the capital expenditure. A considerable variety of systems are available for photoautotrophic production of microalgal biomass, and they are broadly categorized into open raceway ponds and closed photobioreactor systems. Despite the challenge of biological contamination and water loss, raceway ponds are the major commercial production systems of algae biomass because of their large scalability, lower capital, and operational costs (Borowitzka and Vonshak, 2017; Schipper et al., 2021). To balance the strengths and weakness of open and closed systems, algae is cultivated in combined setup of photobioreactor and raceway, called as hybrid system. For production of microalgal biodiesel, two-stage hybrid system could be suitable where exponentially growing algal biomass is transferred from photobioreactor to open raceway ponds under nutrients replete condition to induce higher lipid yield (Liyanaarachchi et al., 2021).
Various research attempted to reduce the capital as well as the expenditure cost of the production systems (Table 1). Capital investment although about one order magnitude lower in an open pond than photobioreactor and again, the construction costs can be reduced by 24–75% if self-sealing layers are developed, rather than using synthetic liners, at the soil-water interface by microalgae and associated organisms through bioclogging process (Coleman et al., 2014; Pattullo et al., 2019). Sapphire Energy demonstrated stable microalgal productivity in an unlined pond (2,000 m2 surface area, 500,000 L volume, 10 cms−1 flow rate) at the Las Cruces test facility, New Mexico without any issues with suspended materials or major water loss through soil (McBride and Merrick, 2014). High-value products like eicosapentaenoic acid (EPA) and omega-3 fatty acid are produced in the unlined pond of Qualitas Health in Imperial, Texas (Efroymson et al., 2020). Greenhouse gas emissions associated with synthetic pond liner manufacture and transportation could be eliminated by using unlined ponds and which eventually will make technology more sustainable (Canter et al., 2014; Greene et al., 2020). Closed raceway ponds were also designed by enclosing a normal raceway ponds with transparent cover that prevents escaping supplied CO2 into atmosphere and consequently reduce the expenditure of CO2. Instead of using paddle wheel, airlift-driven raceway can reduce around 80% power consumption for algal production (Kumar Singh et al., 2021).
A considerable variation exists in data in literature and opinions among experts about the selecting the suitable production system for algal bioenergy. However, a general consensus of several life cycle assessment (LCA) studies indicates raceway ponds are better than photobioreactor in terms of net energy ratio and global warming potential (Ketzer et al., 2018; Herrera et al., 2021). The use of expensive photobioreactor system can be justified for making high-value products. Furthermore, the location of the production facility is one of the divers for selecting a commercial production system. For instance, astaxanthin is produced in raceway ponds in Hawaii, United States by Cyanotech Corporation, but it is not feasible under the sun of the Arava desert due to high water evaporation. Therefore, Algatech in Israel uses photobioreactors for the same product. However, substantial energy is used for cooling the photobioreactor. To minimize the cost associated with temperature control, reactor design or strain improvement should be considered in future research. Similar to NASA’s OMEGA (Offshore Membrane Enclosures for Growing Algae) system, microalgae can be grown in floated plastic tubes in seawater which functions as a temperature buffer (Wiley et al., 2013).
An alternative to the traditional cultivation systems, biofilm-based systems have tested for biofuel production as summarized by Gross et al., in 2015 (Gross et al., 2015). Algal biofilm-based technology was first developed by Walter Adey in the 1980s called Algal Turf Scrubber™ (ATS) in which naturally seeded filamentous algae grow on a screen in a shallow basin through which water is pumped (Adey et al., 2011). This is used to treat wastewater and was commercialized it through a company Hydromentia based in Florida. Rather than in suspension, algae are attached to the surface and harvested through scraping and thus avoid the expensive harvesting procedures in traditional microalgal cultivation. Although ATS is a robust system for algal biomass production and its productivity comparable to the raceway ponds, higher ash (30–50%) and lower lipid in algal biomass are major challenges to use them for biofuel (DeRose et al., 2019). ATS could be a viable option for biofuel production coupling with wastewater treatment if the ash content can be reduced by growing desired algal communities especially by avoiding silica containing diatoms which contribute up to 65% of the total ash (Adey et al., 2011; Kim et al., 2021).
Maintaining long-term, stable, and highly productive algal biomass production in large scale outdoor conditions is the most significant barrier in algal biofuel commercialization. Much like terrestrial crops, microalgal cultivation systems are invaded by weeds, pests, and pathogens, making crop protection a major challenge in the commercialization effort. It is estimated that 30–40% of annual algal crop production is lost to pond crashes (Newby et al., 2016). In this section, we discuss integrated pest management practices (IPM) for healthy algal crop cultivation and a sustainable crop protection process against undesirable biomass losses (Figure 1).
IPM uses proactive strategies rather than controlling the pests in the production system. Selection of robust strain in terms of adaptability in varying water chemistry, tolerant to a wide range of field temperature is an essential criterion for any successful algal field cultivation (Lee and White, 2019; Harmon et al., 2021). A robust stain can withstand the variation of water quality parameters that is common in industrial effluents. Selection of suitable microalgal candidates is crucial for recycling flue gases that has high temperatures, fluctuating gas composition, and the presence of toxic chemicals (Kondaveeti et al., 2020). It is often argued that indigenous strains have better fitness to grow in that local environment (Winckelmann et al., 2015; Mutanda et al., 2020). Sero and colleagues reported that the microalgal strains isolated from extreme urban wastewater environments have inherent biological traits to proliferate in stress and capable of producing high biomass yield using wastewater (Sero et al., 2021). Adaptive evolution, mutagenesis, genetic engineering, and systems biology approaches have been used now for microalgal strain improvement with desired traits (Arora and Philippidis, 2021; Kumar Singh et al., 2021; LaPanse et al., 2021). Growing extremophilic microalgae have a successful commercial history. For example, Dunaliella is used for commercial β-carotene production in extreme salinity, and widely cultivated microalgal species Spirulina is grown in a highly alkaline solution. Extreme conditions help in reducing contaminations from other algal weeds or pests (Varshney et al., 2015; Lafarga et al., 2021). Alkaliphilic Chlorella used for biofuel also showed resistance to grazers in high alkaline cultures (Vadlamani et al., 2017). In addition to pest management, alkaliphilic microalgae can grow efficiently without external sparging of CO2 as alkaline solutions scavenge atmospheric CO2 at high rates. If a direct air capture technology is established with those microalgae, the capital expenditure, as well as around 65% of total operational cost associated with the recovery of CO2 from flue gases and delivery to the production unit, can be eliminated (Davis et al., 2016).
Amoeba, ciliates, rotifers, flagellates and crustaceans are the commonly found grazers in microalgal cultivation (Rajvanshi and Sayre, 2020). Infections from fungi like chytrid, bacteria and virus may affect productivity (Grivalský et al., 2021). Crop rotation over the year is quite essential to prevent pest population buildup. The development of a microalgal cell line resistant to pests is another preventive approach that was demonstrated in Synechococcus elongatus against amoeba attack (Simkovsky et al., 2012). Pesticides or chemicals are often used to mitigate the challenges of contamination like traditional agriculture. Extensive application of insecticide to maintain productivity causes burden of the maintenance cost, development of insecticide resistant pest and water quality loss of nature. More than 550 species of insects were found resistant to insecticide in agriculture, albeit there is no report from algal cultivation (Whalon et al., 2008; Smith and Crews, 2014). However, commercial production of microalgae has the capacity to repeat the same environmental damage if we do not follow the IPM practice. Thus, identification of pests and understanding their life cycle are primary steps to develop a controlling measure. It is possible to forecast pest attacks and take preventive measures if we have clear knowledge about pest biology and their interactions with algae and the environment. Pest monitoring through microscopy is a common practice in algae cultivation. On several occasions, algae crashes were reported within 2–5 days after detection of pests. Thus, increasing the detection sensitivity and developing early detection tools are essential for algal crop protection techniques. Besides monitoring pests through molecular techniques, algal phenotypic response to pests was employed for early detection. For example, rapid decline in quantum yield (Fv/Fm) and non-photochemical quenching in microalgae were reported prior to pond crashes due to parasitic and grazers attack, respectively (McBride et al., 2014; Deore et al., 2020). Infochemicals released by Microchloropsis salina due to the grazers attack was used as a marker for pond health monitoring (Reese et al., 2019; Roccuzzo et al., 2020).
The use of mechanical or biological control rather than conventional chemical treatment is the essential component of IPM strategy (Lee and White, 2019; Al-Jabri et al., 2021). The pest types and their density often determine control operation. Mechanical treatments like pump cavitation or filtering through plankton net are applied to remove larger grazers like rotifers (Kim et al., 2017). Selective feeding of invertebrate consumers can be used to control grazers in algal ponds. Smith and colleagues experimentally demonstrated that introducing zooplanktivorous fish can control the negative impact of grazers and increase lipid productivity in open raceway ponds (Smith et al., 2010). This concept stems from the trophic cascade principle of ecology, which posits that the biomass of primary producers can be maintained by top predators that reduce the population density of primary consumers (Shurin et al., 2014). Invasion of undesired algal strains affects the community structure and alters the biomass productivity and composition that have an impact on the biorefinery process. Maintaining high density cultures in the field is an effective approach to protect against invading algal weed in field cultivation (Richmond et al., 1990). Like pest prevention, biological control was applied to treat small unicellular contaminants such as Chlorella vulgaris and Monoraphidium minutum in Spirulina culture. Herbivorous rotifer Brachionus plicatilis that can selectively ingest only small single-celled algae because of their small mouth opening were introduced in long filamentous Spirulina culture (Mitchell and Richmond, 1987).
Microalgal consortia have the potential to offer crop protection and increase the stability of yields (Smith et al., 2010; Newby et al., 2016; Mattsson et al., 2021). The use of consortia makes the biorefinery process more complex for extracting any species-specific product, however, managing microbial consortia could be a viable industrial practice for biofuel with higher productivity and stability. Consortia benefit from the “portfolio effect”, whereby some species populations will increase in response to pest or environmental fluctuations even if others decline (Shurin et al., 2014). Algal consortia could enhance the nutrients-use efficiency, eventually reducing the fertilizer cost of algal biomass production (Mandal et al., 2018b). In addition, consortia increase productivity in the field through niche partitioning, facilitation, and complementarity (Cardinale et al., 2007; Mandal et al., 2018a). However, random inclusion of species in algal consortia showed success or failures in previous algal biofuel studies. We urge here to design consortia based on the algal complementary traits. Whether it is intentional or nuisance, microbial consortia is the reality for open pond raceway and even in a photobioreactor. Molecular 16S and ITS2 regions analysis of year-long cultivation of industrial microalgal cultivation showed how the diversity of prokaryotic and eukaryotic communities changes over time, and pond productivity and stability positively linked with eukaryotic species diversity of the pond (Beyter et al., 2016). Analogous to rhizosphere in plants, phycosphere is proposed, but it is not studied systematically in commercial production (Wirth et al., 2020). Besides parasitic microbes, many microbes observed in the cultivation have a mutualistic relationship with microalgae and provide essential vitamins for microalgal growth (Kazamia et al., 2012; Yao et al., 2019; Kaur Nagi et al., 2021). Thus, careful management of microbial food-web structure can maximize crop protection and improve crop yield for industrial algal biofuels production (Yun et al., 2016).
In IPM, strong record-keeping and making a correlation of data between yield and operational activities over seasons is a common practice. The factors that determine pest pressure must be identified to predict the pest development time in future operations. The evaluation of the effectiveness of pest control treatments guides selecting the best crop protection strategy. Importantly, translating laboratory-scale results to farm-scale production is a shortcoming in the scaling up of algae cultivation. Field cultivation faces different selection pressures like variable irradiance, temperature, and additional biological challenges—most of which are not seen in the bench-scale studies. To close the lab-to-field yield gap for reliable biomass production, those variables can be tested at a small laboratory scale in more controlled environments before tested at a pilot scale.
Considering the large amounts of wastewater generated globally, around 28–38% of wastewater is treated in developing countries and it became down to almost 8% in underdeveloped ones (Sato et al., 2013). Nitrogen, phosphorus, other macro or micronutrients, the organic carbon in wastewaters is being used for the growth of microalgae. To produce each metric tonne of dry algal biomass requires around 88 kg of elemental N and 12 kg of elemental P, which in turn puts a significant impact on the economy of algal biomass production (Pate et al., 2011). Algal cultivation and wastewater treatment can be integrated to accomplish improved environmental and economic stability. This will not only save the cost of the nutrients of algal biomass production but also surplus the wastewater treatment cost. Techno-economic suggests the production cost can be reduced to more than five times when coupled with wastewater treatment (Acién et al., 2012).
The concept of treating municipal wastewater using microalgae was initiated in the 1950s by Oswald and colleagues at the University of California, Berkeley using high-rate algal ponds with shallow depth and paddlewheel mixed (Oswald and Golueke, 1960; Benemann, 1980; Benemann et al., 1980). Later, it advanced to different types of cultivations systems like photobioreactors, earthen lagoons, concrete tanks, corrugated raceway ponds, biocoils, for use (Craggs et al., 1997; Park et al., 2011; Posadas et al., 2015; Randrianarison and Ashraf, 2017). The advantages and limitations of using an algal turf scrubber system for treating wastewater and biofuel production were described in earlier section. The nutrient removal efficiency of different microalgal strains and their productivities varied in different cultivation systems and wastewater types as illustrated in Table 2. The treatment efficiency of algae-based system and biomass productivity can be improved by operating parameters such as mode of cultivation (batch or continuous), aeration, changing water chemistry (pH, adding require nutrients) (González-Camejo et al., 2021). Further, different stresses like pH, temperature, salinity changes or nutrients reduction in growth media have been suggested to increase lipid yield for biofuel production (Bélanger-Lépine et al., 2018).
TABLE 2. Microalgal nutrients removal efficiency and biomass productivity in different wastewater treatment.
The essentials for evaluation of wastewater treatment schemes involving algae include a clear understanding of the standard steps of treatment to justify the expense of such developmental efforts and more importantly, the characteristics of the wastewater with large flows (Laurens, 2017). These wastewaters are highly turbid, often polluted by algal growth inhibitors like organic compounds in highly toxic concentrations, salt accumulations, and allelopathic agents excreted by algae themselves (Bacellar Mendes and Vermelho, 2013). Be it a monoculture or polyculture of microalgal strains, efficient pilot harvesting of biomass is vital, especially when the treated wastewater must be brought to re-use. Leaving back the traditional concept of drying, solvent extraction of lipids, and transesterification for the production of fatty acid methyl esters, all the time more interest is being directed towards the hydrothermal liquefaction (HTL) process for bio-oil productions (Al-Jabri et al., 2021; Chen and Quinn, 2021). This aqueous phase from the HTL process contains high concentrations of nutrients like nitrogen, phosphorus, and other elements that can be recycled for microalgal growth. While varying compositions of the algal biomass in wastewater is a shortcoming in the refinery process, converting the carbohydrates, proteins, and lipids agnostically to bio-oil would be a feasible choice.
Amongst various non-conventional sources, microalgae are promising microorganisms that play a key role in the biobased economy, since they serve as a continuous and reliable source of several bioactive natural products (Fabris et al., 2020). Microalgae are factories for producing various compounds other than the only lipid for making biofuel. Lipid is converted into biodiesel through transesterification process in which triglycerides react with alcohol in the presence catalyst. In thermochemical process like pyrolysis, gasification, combustion or hydrothermal liquefaction biomass is thermally breakdown into organic chemicals which reform into various types of biofuels (Figure 2). The biochemical conversion involves the hydrolysis of biomass by bacteria into fermentable sugars which is converted into bioethanol, biogas and biohydrogen (Saad et al., 2019). As shown in Figure 2, the algal biomass residue after high-value co-products and biodiesel production can be route into thermochemical or biochemical process for maximal valorization of algal materials. However, most microalgal companies focus on single product development. Recently, the focus of microalgae biomass delivering a single product is shifting towards delivering multiple products along with lipid derived biofuels in a biorefinery approach (Wijffels et al., 2010; Ansari et al., 2017). The current production of microalgae derived products (more than 75%) are finding their way towards food, feed, nutraceutical, and cosmetic industries (Nethravathy et al., 2019; Rahman, 2020). Thus, if algal biofuel is combined with the production of bulk chemicals, food, and feed ingredients, the cost gap between biofuel and fossil fuel would be closed (Shukla and Kumar, 2018). The proposed biorefinery approach, on other hand, may cause the market saturation of high-value products. Thus, the market niche and demand of such algal high-value products must be analyzed critically. However, nowadays people are trending towards natural products, especially in the COVID era. In the following section, we analyzed the algal products that can zeal with algal biofuel, their potential, current industrial situation, future market (Table 3).
Microalgal pigments is a profitable business nowadays. Pigments from algae such as Dunaliella, Scenedesmus, Nannochloropsis, Haematococcus, Muriellopsis, Chlorella, Phaeodactylum, Spirulina, Porphyridium have gained more popularity in the health food industry as they produce carotenoids, chlorophylls, and phycobiliproteins in high amounts (Noreña-Caro and Benton, 2018; Arashiro et al., 2020; Silva et al., 2020). Currently, the global demand for pigments produced from natural sources is growing rapidly with great health benefits to humans (“BCC Research: Market Research., 2021 Reports and Industry Analysis”). In terms of commercialization, pigments from microalgae have a high revenue generation > USD 1 billion (selling price—USD 400/kg) and the global carotenoid market is expected to be USD 2.0 billion by 2026. The lutein and zeaxanthin eye health care market from microalgae crosses USD250 million per year. The market for canthaxanthin and zeaxanthin is still in its developing stages (Lin et al., 2015; Pereira et al., 2021). On other hand, the average market size of astaxanthin and β-carotene from Dunaliella for food supplements is in the range of 2,500 USD per kilograms and 75 million USD (Hejazi and Wijffels, 2004; BIOPRO, 2013; Levasseur et al., 2020). Astaxanthin is considered the leading molecule propelling microalgal biorefinery (Dawidziuk et al., 2017; Khoo et al., 2019) with production costs shifting between 300 and 3600 USD/kg depending upon the purity (Li et al., 2011; Dawidziuk et al., 2017). Algae Health Science, Yunnan, China is one of the biggest producers of astaxanthin from Haematococcus pluvialis (Schultz., 2020). Numerous pigments such as astaxanthin from marine algae, xanthophylls, and phycobiliproteins from red algae, have a great potential in cosmetic application (Morocho-Jácome et al., 2020).
According to a recent study, mainly Spirulina and Chlorella are the key algal strains that top the algal market worldwide in health and nutrition with a production of 12,000 tons per year and 5,000 tons per year, respectively (Koyande et al., 2019; Nethravathy et al., 2019; Wang et al., 2020). Chlorophyll and phycobiliproteins have been widely used as coloring agents and fluorescent markers in both strains due to their high stability (Pulz and Gross, 2004; Dasgupta, 2016). Fucoxanthin product named fücoTHIN® was used as a supplement in body weight products (Liu J., 2016; Liu Q., 2016). Moreover, this valuable pigment can be used in animal feed products as it is regarded as safe (Yi et al., 2015). Algal Technologies Ltd., Israel (2018) reported the fucoxanthin production from microalgae with a growing global market of approximately USD 600 million during 2018–2025 (Global Fucoxanthin Market, 2020). The effective use of microbial pigments depends on high productivity, production costs, pigment characterization, and stability at a broader range of temperature and light (Morales-Oyervides et al., 2017). Being the most revenue generating compounds in algal biorefinery pigments can play a major role in the economy of biofuel production (Ruiz et al., 2016; Mutanda et al., 2020).
Microalgae are known for producing proteins with a healthy balance of essential amino acids and widely used for decades as a feedstock in the pharma and nutrition sectors. In past years, proteins from microalgae have now been investigated thoroughly in food sciences as a cheap and more sustainable source, qualifying as proven alternatives to conventional ones, thus meeting the global demands of protein in nutrition (Kay and Barton, 1991; Becker, 2007). Several microalgal strains have a protein content higher than conventional plant or animal sources. For example, protein content in Spirulina platensis is 65%, higher than that in meat (45%), soy flour (37%), milk (24%), or fish (24%) (Younes et al., 2011; Barka and Blecker, 2016; Ritala et al., 2017).
Algal proteins from Chlorella and Spirulina are recognized as safe for human consumption. The market for algae protein has witnessed a huge upsurged demand, due to their high nutritional value, exploration by the vegan population, and being a sustainable source. Currently, among the algal sources of protein, blue-green algae hold the largest market share with revenue surpassing USD 300 million in 2019. Asia- Pacific algal protein market is expected to witness 6.5% CAGR till 2026. In 2019, Swiss food manufacturer Nestle made a strategic partnership with Corbion for the development of commercial microalgae-based protein products. The microalgae proteins and peptides hold anticancer, immunosuppressive, anti-hypertensive, and antioxidant properties (Wang and Zhang, 2013). Microalgal proteins are mostly being used as supplements, and are available in the market in form of tablets, capsules, or liquid. The use of microalgae as a bulk commodity in human food is rare because of their unfavorable sensory attributes like the smell, color, and texture; a smaller part is applied as an ingredient in pasta, baked goods, snacks (Ritala et al., 2017). Microalgal protein is now used as an ingredient of meat analogs through modifying texture and flavor in food processing techniques (Fu et al., 2021). Microalgae or protein are proven feedstock for the animal. For instance, Scenedesmus obliquus protein extracted from a sequential refinery process was used as an alternative to a fish meal before converting biomass into biodiesel (Patnaik et al., 2019).
Extracting the protein from algal biomass before processing it into biofuel can make the microalgal biofuels economically viable. Several researchers have reported the production of proteins along with advanced biofuels from Chlorella and Scenedesmus (Illman et al., 2000). However, a technoeconomic analysis of the algal biorefinery process revealed the extraction and purification of soluble protein with chemical extraction followed by diafiltration membrane purification encompassed about 75% of refinery cost (Suarez Ruiz et al., 2018). Thus, developing a suitable technology for this refinery cost reduction is one of the critical challenges in the bioprocess.
Microalgae are well known for being the source of PUFA such as γ-linolenic acid, arachidonic acid, eicosapentaenoic acid (EPA), and docosahexaenoic acid (DHA) (Ratledge, 2010). Although, both EPA and DHA from fish oil dominate the market, the demand for microalgal sources is increasing because of the vegan characteristic of algal oil. The presence of persisting contaminants such as dioxins, heavy metals such as methyl mercury, and polychlorinated bisphenols in fish oil is also a challenge (Ruiz-Rodriguez et al., 2010; Ryckebosch et al., 2014). Advantages of fatty acids from microalgae were also observed against inflammation and cardiac related disease such as myocardial infarction, hypertension, thrombosis, etc. (Nauroth et al., 2010; Adarme-Vega et al., 2014). PUFAs, particularly DHA and EPA, are reported to have a therapeutic role in a variety of inflammatory pathologies, for instance, arthritis, Alzheimer’s disease, and lupus (Yates et al., 2014). There is an increasing market potential for long chain polyunsaturated fatty acids (LC-PUFAs) due to their intense application in health (Saini and Keum, 2018). PUFA market is likely to expand at an annual growth rate of 13.5% globally (Rahman, 2020). Several microalgal species like Schizochytrium, Crypthecodinium, and Ulkenia have been cultivated heterotrophically for DHA production at an industrial scale. The company, DSM which is the major driver in this particular oil market, commercialized a DHA rich oil from “Crypthecodinium cohnii”. called DHASCO™ (Wynn et al., 2010). This is popularly used in infant formula, supplements, and products for pregnancy and nursing. DSM commercialized another DHA and EPA rich algal oil, Life’s TM OMEGA, which is approved for use as a novel food ingredient in specific food categories and dietary supplements. Martek Biosciences commercialized DHA production from the microalgae Crypthecodinium.
Green alga Parietochloris incisa (Bigogno et al., 2002) comprises a higher amount of arachidonic acid content; though, the total content of lipid is lower when compared to other existing commercialized fungus for arachidonic acid production. Spirulina platensis is the best source for linolenic acid production (Tanticharoen et al., 1994). EPA producing microalgal strains, in particular, Nitzchia, Nannochloropsis, and Phaeodactylum tricornutum are widely cultivated (Spolaore et al., 2006). Almega PL™, an EPA-rich product is marketed by Qualitas Health by using autotrophic production of microalgal biomass. In a sustainable biorefinery approach, omega-3 fatty acids can be separated from microalgal lipids, while the rest of the lipid or other components of the biomass could be used for making food, fuel, or other valuables. For example, after the separation of omega-3 fatty acids from Nannochloropsis salina oil, the waste oils were used to produce flexible polyurethane foam (Phung Hai et al., 2020). This biodegradable polyurethane foam is an alternative to petroleum-based polymer and showed its application in making footwear and surfboard. In recent years, concurrent production of fucoxanthin and docosahexaenoic acid from Isochrysis strain has been examined with encouraging effects (Sun et al., 2019).
Next to pigments and fatty acids, microalgae have long been of interest as sources of bioactive compounds to use in cosmetics. Bioactive compounds from microalgae have potential applications like water-binding, thickening, and antioxidant agents, prevention of hyperpigmentation, stimulation of bleaching, modulation of melanogenesis in hair, melanocyte proliferation, improvement and stimulation of keratinocyte differentiation, growth of human hair follicles, improvement or maintenance of skin’s barrier function, improvement of aged skin appearance, collagen stimulation, and improving skin’s firmness and elasticity (Levasseur et al., 2020; Randhir et al., 2020; Chouhan et al., 2021). Several protective and efficient systems against the free radicals and reactive oxygen species are developed in algae because of the natural exposure to oxidative stress. This, in turn, produces compounds that can be used to replace the currently employed organic and inorganic filters against the damaging effects of UV radiation (Wheeler et al., 2008; Gouveia et al., 2009).
Both Nannochloropsis and Isochrysis have been found effective against UVA and UVB transmissions with the same profile as any formulation containing SPF15 fighting organic and inorganic filters (Lotan, 2012). Compared to the sunscreen formulations used commercially, cyanobacteria showed better absorption in the visible spectral region and UV A, UVB region as well, i.e., 290–650 nm (Ariede et al., 2017). Mycosporine-like amino acids such as asterina, palythene, palythine, and porphyra have been reportedly produced by cyanobacteria of Nostoc sp. R76Dm and have shown in-vivo reactive oxygen species (ROS) scavenging potential and in-vitro dose-dependent antioxidant potential (Rastogi et al., 2016).
Pentapharm in Basel, Switzerland launched a commercial product called Pepha-Tight using a compound from Nannochloropsis oculata for short-term and long-term skin-tightening properties and other called Pepha-Ctive using extracts from Dunaliella salina to positively influence the energy metabolism of the skin and to stimulate cell proliferation (Spolaore et al., 2006). The cosmetic industry is growing worldwide with a market size was valued at $380.2 billion in 2019, and is projected to reach $463.5 billion by 2027, registering a compound annual growth rate of 5.3% from 2021 to 2027 (Chouhan et al., 2021). Rapid growth in this industry can make a market niche for algal cosmetics when combined with biofuel.
Microalgae are also a source of several minerals and vitamins like vitamin A, vitamins of the B group like B1, B2, B3, B5, B6, B8, B9, B12, vitamin C and E. Phytohormones like abscisic acid, gibberellins, auxin, cytokinin, ethylene, polyamines, salicylates, signal peptides, and brassinosteroids are produced by most of the microalgal lineages (Bajguz and Piotrowska-Niczyporuk, 2013; Galasso et al., 2019; Levasseur et al., 2020). Algae derived bioactive compounds have been suggested for treating COVID-19 disease (Chia et al., 2021). Approximately 73,000 algal species have been identified but few reached the commercial scale (Guiry, 2012). However, there is numerous germplasm that need to be explored for the production of valuable products. Despite the suitability of algae for biorefining, holding the functionality of the different compounds in the refinery process is a challenge. Research is needed to explore the compatibility of the compounds in down streaming processing and to reduce the materials and energy consumption in the process. Economics of microalgal downstream processing including cell disruption, extraction, purification, and biomass conversion must be evaluated for the sustainability of biorefinery process.
Commercial microalgae production was started with the cultivation Chlorella for the single-cell protein in the early 1960s in Japan, followed by Spirulina in the US, and then in China and Thailand (Lee, 1997; Borowitzka, 2013). In the 1980s, efforts were initiated to produce microalgal pigments, predominantly beta-carotene and astaxanthin, through the cultivation of Dunaliella sp. and Haematococcus sp. In the 1980s, commercial production of PUFAs, especially EPA and DHA, was started for nutraceuticals application. In the early of this century around 2005, a number of companies like Algenol, Cellana, Origin Oil, Aurora Biofuel, PetroAlgae, PowerFuel.de, Shell Oil, Solix Biofuel, Sapphire Energy, and Solazyme raised remarkable private sector investment with a promise of producing algal biofuel competitive with the fossil fuel (Waltz, 2009). In India, Reliance Industries invested with Algenol, United States to recycles carbon dioxide into fuels through its direct-to-ethanol process near Jamnagar petroleum refinery. Sapphire Energy used hydrothermal liquefaction technology to make “crude-like oil” that can be refined into gasoline or jet fuel. The estimated minimum price for algae biofuel was $2.1 per liter which weighs high than regular gasoline (Gu et al., 2020). Today, most algae companies except for ExxonMobil and Synthetic Genomics shifted their business model into high-value products. ExxonMobil and Synthetic Genomics reported the doubling of lipid production without compromising growth through genetic modification in Nannochloropsis gaditana using CRISPR–Cas9 genome editing techniques (Ajjawi et al., 2017). Their joint algae biofuel research program targets to produce 10,000 barrels of algae biofuel per day by 2025 using genetically modified strain. However, the phenotype stability of engineered strain in the field along with the concern of environmental risk growing often raised the question of the genetically engineering approach for microalgal biofuel research. The finding of the first field trial of genetically modified algae by researchers from the University of California San Diego and Sapphire Energy, United States was encouraging as genetically modified Acutodesmus dimorphus conserved the genetically modified phenotypes in field cultivation without impacting the phytoplankton communities in native lakes (Szyjka et al., 2017).
After few years from 2005 when microalgae did not reach the economic target, the potential of microalgae for biofuel was debated and called “hype” (Waltz, 2009). In a reply to the news feature “Biotech’s green gold?” in Nature Biotechnology (Waltz, 2009), Stephens and colleagues demonstrated microalgae are capable of producing ∼60–100 kl oil ha−1 y−1 on a practical conservative scale (Stephens et al., 2010). Algae-based transportation fuels have already demonstrated their ability to drive personal automobiles, fly commercial planes, and power Navy ships. In our view, it not about the potential of algae, it is all about technological readiness to compete with fossil fuel.
Although algal biofuel didn’t reach yet the economic target, Life cycle assessment (LCA) of algal biofuel from the pilot-scale facility of Sapphire Energy was found to have lower greenhouse gas (GHG) emissions than corn ethanol and petroleum fuels (Liu et al., 2013). Interestingly, Energy Return on Energy Investment (EROI) which is the key for measuring the sustainability of any energy technology was above one in their pilot-scale analysis and varied between one and four depending on the scale of production.
Microalgal biofuel remains in an early stage of development. In our view, we invested only a few years from capital injection to demonstrating large-scale commercial production. Venture capitalists should consider the challenges and barriers that need to be overcome before this technology is commercialized. Even, the demonstration plants (at <5 ha) that were used for estimating the cost analysis were well weighed below the size threshold for economic viability. To make “high volume, low-cost product” like biofuel, scaling up microalgal cultivation system to a commercial level is a key in the process development. Although microalgae are an excellent feedstock of multiple products, maintaining the stability of all chemicals with their bioavailability is critical challenge in adapting biorefinery approach. A substantial innovation is required in downstream processing steps like milder cell disruption technologies, solvents or supercritical fluid extraction to ensure the functionality of the products reserve in the process. Research should be carried out to find the appropriate sequence of products extraction from microalgal biomass in refinery process. As of now, current market values of algal nutraceutical are quite high when the global production of microalgae is inadequate. Apparently integrating biofuel systems with industrial commodities production looks economically sustainable but replacing only a part of fossil fuel with biofuel can make a surge of high-value products in the market and affect product prices. Awareness among people about algal products must be improved to reach a sustainable biorefinery. Improving downstream processing certainly is an essential step, but to produce enough biomass to feed the process is more critical in algal biofuel commercialization. Successful algae cultivation in the field demands a more ecological approach rather than industrial microbiology. Algae need to be considered as an agricultural crop, and robust agronomic and integrated pest management practices must be developed as cheaply as possible. Indeed, the 2018 Farm Bill classified algae as a crop in US policy and support the algae program. Research investment, policy development, and new scientific discoveries will pave the way for the development of viable microalgal biofuel platforms in the near future. In the meantime, valorizing high-value co-products is a feasible option for microalgal biofuel commercialization.
Each author contributed to the literature review and analysis and to the writing of the paper. SM was the research supervisor and conceptualized the manuscript. All authors have read and agreed to the published version of the manuscript.
The authors declare that the research was conducted in the absence of any commercial or financial relationships that could be construed as a potential conflict of interest.
All claims expressed in this article are solely those of the authors and do not necessarily represent those of their affiliated organizations, or those of the publisher, the editors and the reviewers. Any product that may be evaluated in this article, or claim that may be made by its manufacturer, is not guaranteed or endorsed by the publisher.
SM is thankful to the Department of Biotechnology, India for the Ramalingaswami Fellowship.
Acién, F. G., Fernández, J. M., Magán, J. J., and Molina, E. (2012). Production Cost of a Real Microalgae Production Plant and Strategies to Reduce it. Biotechnol. Adv. 30, 1344–1353. doi:10.1016/j.biotechadv.2012.02.005
Adarme-Vega, T. C., Thomas-Hall, S. R., and Schenk, P. M. (2014). Towards Sustainable Sources for omega-3 Fatty Acids Production. Curr. Opin. Biotechnol. 26, 14–18. doi:10.1016/j.copbio.2013.08.003
Adey, W. H., Kangas, P. C., and Mulbry, W. (2011). Algal Turf Scrubbing: Cleaning Surface Waters with Solar Energy while Producing a Biofuel. Bioscience 61, 434–441. doi:10.1525/bio.2011.61.6.5
Ajjawi, I., Verruto, J., Aqui, M., Soriaga, L. B., Coppersmith, J., Kwok, K., et al. (2017). Lipid Production in Nannochloropsis Gaditana Is Doubled by Decreasing Expression of a Single Transcriptional Regulator. Nat. Biotechnol. 35, 647–652. doi:10.1038/nbt.3865
AlgoSource Natural Bioactive Extracts from Microalgae (2020). 2021. Available at: https://algosource.com/. [Accessed August 6, 2021].
Al-Jabri, H., Das, P., Khan, S., Thaher, M., and Abdulquadir, M. (2021). Treatment of Wastewaters by Microalgae and the Potential Applications of the Produced Biomass-A Review. Water 13, 27. doi:10.3390/w13010027
Amit, Nayak, J. K., Nayak, J. K., and Ghosh, U. K. (2020). Microalgal Remediation of Anaerobic Pretreated Pharmaceutical Wastewater for Sustainable Biodiesel Production and Electricity Generation. J. Water Process Eng. 35, 101192. doi:10.1016/j.jwpe.2020.101192
Ansari, F. A., Singh, P., Guldhe, A., and Bux, F. (2017). Microalgal Cultivation Using Aquaculture Wastewater: Integrated Biomass Generation and Nutrient Remediation. Algal Res. 21, 169–177. doi:10.1016/j.algal.2016.11.015
Arashiro, L. T., Boto-Ordóñez, M., Van Hulle, S. W. H., Ferrer, I., Garfí, M., and Rousseau, D. P. L. (2020). Natural Pigments from Microalgae Grown in Industrial Wastewater. Bioresour. Tech. 303, 122894. doi:10.1016/j.biortech.2020.122894
Ariede, M. B., Candido, T. M., Jacome, A. L. M., Velasco, M. V. R., de Carvalho, J. C. M., and Baby, A. R. (2017). Cosmetic Attributes of Algae - A Review. Algal Res. 25, 483–487. doi:10.1016/j.algal.2017.05.019
Arora, N., and Philippidis, G. P. (2021). Microalgae Strain Improvement Strategies: Random Mutagenesis and Adaptive Laboratory Evolution. Trends Plant Sci. S1360-1385 (21), 147–153. doi:10.1016/j.tplants.2021.06.005
Bacellar Mendes, L., and Vermelho, A. (2013). Allelopathy as a Potential Strategy to Improve Microalgae Cultivation. Biotechnol. Biofuels 6, 152. doi:10.1186/1754-6834-6-152
Bachchhav, M. B., Kulkarni, M. V., and Ingale, A. G. (2020). Process-intensified Extraction of Phycocyanin Followed by β-carotene from Spirulina Platensis Using Ultrasound-Assisted Extraction. Sep. Sci. Tech. 55, 932–944. doi:10.1080/01496395.2019.1580293
Bajguz, A., and Piotrowska-Niczyporuk, A. (2013). Synergistic Effect of Auxins and Brassinosteroids on the Growth and Regulation of Metabolite Content in the green Alga Chlorella Vulgaris (Trebouxiophyceae). Plant Physiol. Biochem. 71, 290–297. doi:10.1016/j.plaphy.2013.08.003
Baldev, E., Mubarak Ali, D., Pugazhendhi, A., and Thajuddin, N. (2021). Wastewater as an Economical and Ecofriendly green Medium for Microalgal Biofuel Production. Fuel 294, 120484. doi:10.1016/j.fuel.2021.120484
Barka, A., and Blecker, C. (2016). Microalgae as a Potential Source of Single-Cell Proteins. A. Review. Biotechnol. Agron. Soc. Environ. 20, 427–436. doi:10.25518/1780-4507.13132
Bélanger-Lépine, F., Tremblay, A., Huot, Y., and Barnabé, S. (2018). Cultivation of an Algae-Bacteria Consortium in Wastewater from an Industrial Park: Effect of Environmental Stress and Nutrient Deficiency on Lipid Production. Bioresour. Tech. 267, 657–665. doi:10.1016/j.biortech.2018.07.099
Becker, E. W. (2007). Micro-algae as a Source of Protein. Biotechnol. Adv. 25, 207–210. doi:10.1016/j.biotechadv.2006.11.002
Benemann, J. R., Miyamoto, K., and Hallenbeck, P. C. (1980). Bioengineering Aspects of Biophotolysis. Enzyme Microb. Tech. 2, 103–111. doi:10.1016/0141-0229(80)90064-2
Beyter, D., Tang, P.-Z., Becker, S., Hoang, T., Bilgin, D., Lim, Y. W., et al. (2016). Diversity, Productivity, and Stability of an Industrial Microbial Ecosystem. Appl. Environ. Microbiol. 82, 2494–2505. doi:10.1128/AEM.03965-15
Bhattacharya, M., and Goswami, S. (2020). Microalgae - A green Multi-Product Biorefinery for Future Industrial Prospects. Biocatal. Agric. Biotechnol. 25, 101580. doi:10.1016/j.bcab.2020.101580
Bigogno, C., Khozin-Goldberg, I., Boussiba, S., Vonshak, A., and Cohen, Z. (2002). Lipid and Fatty Acid Composition of the green Oleaginous Alga Parietochloris Incisa, the Richest Plant Source of Arachidonic Acid. Phytochemistry 60, 497–503. doi:10.1016/S0031-9422(02)00100-0
Bioeconomy (2020). Microalgae Can Produce More Than Just Fuel. 1–4. Available at: https://www.biooekonomie-bw.de/en/articles/news/microalgae-can-produce-more-than-just-fuel/. [Accessed August 7, 2021].
BIOPRO (2013). Microalgae Can Produce More Than Just Fuel. 1–4. Available at: https://www.biooekonomie-bw.de/en/articles/news/microalgae-can-produce-more-than-just-fuel. [Accessed June 27, 2021].
Borowitzka, M. A., and Vonshak, A. (2017). Scaling up Microalgal Cultures to Commercial Scale. Eur. J. Phycology 52, 407–418. doi:10.1080/09670262.2017.1365177
Borowitzka, M. A. (2013). “Energy from Microalgae: A Short History,” in Algae for Biofuels and Energy (Springer Netherlands), 1–15. doi:10.1007/978-94-007-5479-9_1
Branco-Vieira, M., San Martin, S., Agurto, C., Santos, M., Freitas, M., Mata, T., et al. (2018). Potential of Phaeodactylum Tricornutum for Biodiesel Production under Natural Conditions in Chile. Energies 11, 54. doi:10.3390/en11010054
Canter, C. E., Davis, R., Urgun-Demirtas, M., and Frank, E. D. (2014). Infrastructure Associated Emissions for Renewable Diesel Production from Microalgae. Algal Res. 5, 195–203. doi:10.1016/j.algal.2014.01.001
Cardinale, B. J., Wright, J. P., Cadotte, M. W., Carroll, I. T., Hector, A., Srivastava, D. S., et al. (2007). Impacts of Plant Diversity on Biomass Production Increase through Time Because of Species Complementarity. Proc. Natl. Acad. Sci. 104, 18123–18128. doi:10.1073/pnas.0709069104
Cheah, W. Y., Show, P. L., Yap, Y. J., Mohd Zaid, H. F., Lam, M. K., Lim, J. W., et al. (2020). Enhancing Microalga Chlorella Sorokiniana CY-1 Biomass and Lipid Production in palm Oil Mill Effluent (POME) Using Novel-Designed Photobioreactor. Bioengineered 11, 61–69. doi:10.1080/21655979.2019.1704536
Chen, P. H., and Quinn, J. C. (2021). Microalgae to Biofuels through Hydrothermal Liquefaction: Open-Source Techno-Economic Analysis and Life Cycle Assessment. Appl. Energ. 289, 116613. doi:10.1016/j.apenergy.2021.116613
Chen, Z., Shao, S., He, Y., Luo, Q., Zheng, M., Zheng, M., et al. (2020). Nutrients Removal from Piggery Wastewater Coupled to Lipid Production by a Newly Isolated Self-Flocculating Microalga Desmodesmus Sp. PW1. Bioresour. Tech. 302, 122806. doi:10.1016/j.biortech.2020.122806
Chia, W. Y., Kok, H., Chew, K. W., Low, S. S., and Show, P. L. (2021). Can Algae Contribute to the War with Covid-19? Bioengineered 12, 1226–1237. doi:10.1080/21655979.2021.1910432
Chisti, Y. (2007). Biodiesel from Microalgae. Biotechnol. Adv. 25, 294–306. doi:10.1016/j.biotechadv.2007.02.001
Chouhan, N., Himanshu, Vig., and Deshmukh, R. (2021). Cosmetics Market Size, Share, Industry Trends & Analysis 2021–2027. 338. Available at: https://www.alliedmarketresearch.com/cosmetics-market. [Accessed June 6, 2021].
Coleman, A. M., Abodeely, J. M., Skaggs, R. L., Moeglein, W. A., Newby, D. T., Venteris, E. R., et al. (2014). An Integrated Assessment of Location-dependent Scaling for Microalgae Biofuel Production Facilities. Algal Res. 5, 79–94. doi:10.1016/j.algal.2014.05.008
Craggs, R. J., McAuley, P. J., and Smith, V. J. (1997). Wastewater Nutrient Removal by marine Microalgae Grown on a Corrugated Raceway. Water Res. 31, 1701–1707. doi:10.1016/S0043-1354(96)00093-0
Cruce, J. R., and Quinn, J. C. (2019). Economic Viability of Multiple Algal Biorefining Pathways and the Impact of Public Policies. Appl. Energ. 233-234, 735–746. doi:10.1016/j.apenergy.2018.10.046
Dasgupta, C. N. (2015). “Algae as a Source of Phycocyanin and Other Industrially Important Pigments,” in Algal Biorefinery: An Integrated Approach (Springer International Publishing), 253–276. doi:10.1007/978-3-319-22813-6_12
Davis, R., Markham, J., Kinchin, C., Grundl, N., Tan, E., and Humbird, D. (2016). “Algal Biomass Production in Open Pond Systems and Processing through Dewatering for Downstream Conversion,” in Process Design and Economics for the Production of Algal Biomass (Golden: NREL). Available at: www.nrel.gov/publications.
Dawczynski, C., Dittrich, M., Neumann, T., Goetze, K., Welzel, A., Oelzner, P., et al. (2018). Docosahexaenoic Acid in the Treatment of Rheumatoid Arthritis: A Double-Blind, Placebo-Controlled, Randomized Cross-Over Study with Microalgae vs. sunflower Oil. Clin. Nutr. 37, 494–504. doi:10.1016/j.clnu.2017.02.021
Dawidziuk, A., Popiel, D., Luboinska, M., Grzebyk, M., Wisniewski, M., and Koczyk, G. (2017). Assessing Contamination of Microalgal Astaxanthin Producer Haematococcus Cultures with High-Resolution Melting Curve Analysis. J. Appl. Genet. 58, 277–285. doi:10.1007/s13353-016-0378-x
Deore, P., Karthikaichamy, A., Beardall, J., and Noronha, S. (2020). Non-photochemical Quenching, a Non-invasive Probe for Monitoring Microalgal Grazing: an Early Indicator of Predation by Oxyrrhis marina and Euplotes Sp. Appl. Phycology 1, 20–31. doi:10.1080/26388081.2019.1651218
DeRose, K., DeMill, C., Davis, R. W., and Quinn, J. C. (2019). Integrated Techno Economic and Life Cycle Assessment of the Conversion of High Productivity, Low Lipid Algae to Renewable Fuels. Algal Res. 38, 101412. doi:10.1016/j.algal.2019.101412
Efroymson, R. A., Pattullo, M. B., Mayes, M. A., Mathews, T. J., Mandal, S., and Schoenung, S. (2020). Exploring the Sustainability and Sealing Mechanisms of Unlined Ponds for Growing Algae for Fuel and Other Commodity-Scale Products. Renew. Sust. Energ. Rev. 121, 109708. doi:10.1016/j.rser.2020.109708
Fabris, M., Abbriano, R. M., Pernice, M., Sutherland, D. L., Commault, A. S., Hall, C. C., et al. (2020). Emerging Technologies in Algal Biotechnology: Toward the Establishment of a Sustainable, Algae-Based Bioeconomy. Front. Plant Sci. 11, 279. doi:10.3389/fpls.2020.00279
Figueroa-Torres, G. M., Wan Mahmood, W. M. A., Pittman, J. K., and Theodoropoulos, C. (2020). Microalgal Biomass as a Biorefinery Platform for Biobutanol and Biodiesel Production. Biochem. Eng. J. 153, 107396. doi:10.1016/j.bej.2019.107396
Fu, Y., Chen, T., Chen, S. H. Y., Liu, B., Sun, P., Sun, H., et al. (2021). The Potentials and Challenges of Using Microalgae as an Ingredient to Produce Meat Analogues. Trends Food Sci. Tech. 112, 188–200. doi:10.1016/j.tifs.2021.03.050
Galasso, C., Gentile, A., Orefice, I., Ianora, A., Bruno, A., Noonan, D. M., et al. (2019). Microalgal Derivatives as Potential Nutraceutical and Food Supplements for Human Health: A Focus on Cancer Prevention and Interception. Nutrients 11, 1226. doi:10.3390/nu11061226
Garrido-Cardenas, J. A., Manzano-Agugliaro, F., Acien-Fernandez, F. G., and Molina-Grima, E. (2018). Microalgae Research Worldwide. Algal Res. 35, 50–60. doi:10.1016/j.algal.2018.08.005
Georgianna, D. R., and Mayfield, S. P. (2012). Exploiting Diversity and Synthetic Biology for the Production of Algal Biofuels. Nature 488, 329–335. doi:10.1038/nature11479
Global Fucoxanthin Market (2020). Global Fucoxanthin Market 2020. Available at: https://www.marketresearchstore.com/market-insights/fucoxanthin-market-809578. [Accessed June 27, 2021].
González‐Camejo, J., Ferrer, J., Seco, A., and Barat, R. (2021). Outdoor Microalgae‐based Urban Wastewater Treatment: Recent Advances, Applications, and Future Perspectives. WIREs Water 8, e1518. doi:10.1002/wat2.1518
Gouveia, L., Batista, A. P., Sousa, I., Raymundo, A., and Bandarra, N. M. (2009). Microalgae in Novel Food Products.
Greene, J. M., Gulden, J., Wood, G., Huesemann, M., and Quinn, J. C. (2020). Techno-economic Analysis and Global Warming Potential of a Novel Offshore Macroalgae Biorefinery. Algal Res. 51, 102032. doi:10.1016/j.algal.2020.102032
Grivalský, T., Střížek, A., Přibyl, P., Lukavský, J., Čegan, R., Hobza, R., et al. (2021). Comparison of Various Approaches to Detect Algal Culture Contamination: a Case Study of Chlorella Sp. Contamination in a Phaeodactylum Tricornutum Culture. Appl. Microbiol. Biotechnol. 105, 5189–5200. doi:10.1007/S00253-021-11396-7
Gross, M., Jarboe, D., and Wen, Z. (2015). Biofilm-based Algal Cultivation Systems. Appl. Microbiol. Biotechnol. 99, 5781–5789. doi:10.1007/s00253-015-6736-5
Gu, X., Yu, L., Pang, N., Martinez-Fernandez, J. S., Fu, X., and Chen, S. (2020). Comparative Techno-Economic Analysis of Algal Biofuel Production via Hydrothermal Liquefaction: One Stage versus Two Stages. Appl. Energ. 259, 114115. doi:10.1016/j.apenergy.2019.114115
Guiry, M. D. (2012). How many Species of Algae Are There? J. Phycol. 48, 1057–1063. doi:10.1111/j.1529-8817.2012.01222.x
Hannon, M., Gimpel, J., Tran, M., Rasala, B., and Mayfield, S. (2010). Biofuels from Algae: Challenges and Potential. Biofuels 1, 763–784. doi:10.4155/bfs.10.44
Harmon, V. L., Wolfrum, E., Knoshaug, E. P., Davis, R., Laurens, L. M. L., Pienkos, P. T., et al. (2021). Reliability Metrics and Their Management Implications for Open Pond Algae Cultivation. Algal Res. 55, 102249. doi:10.1016/j.algal.2021.102249
Harvey, P. J., and Ben-Amotz, A. (2020). Towards a Sustainable Dunaliella salina Microalgal Biorefinery for 9-cis β-carotene Production. Algal Res. 50, 102002. doi:10.1016/j.algal.2020.102002
Hejazi, M. A., and Wijffels, R. H. (2004). Milking of Microalgae. Trends Biotechnol. 22, 189–194. doi:10.1016/j.tibtech.2004.02.009
Herrera, A., D’Imporzano, G., Acién Fernandez, F. G., and Adani, F. (2021). Sustainable Production of Microalgae in Raceways: Nutrients and Water Management as Key Factors Influencing Environmental Impacts. J. Clean. Prod. 287, 125005. doi:10.1016/j.jclepro.2020.125005
Hu, Q., Sommerfeld, M., Jarvis, E., Ghirardi, M., Posewitz, M., Seibert, M., et al. (2008). Microalgal Triacylglycerols as Feedstocks for Biofuel Production: Perspectives and Advances. Plant J. 54, 621–639. doi:10.1111/j.1365-313X.2008.03492.x
Illman, A. M., Scragg, A. H., and Shales, S. W. (2000). Increase in Chlorella Strains Calorific Values when Grown in Low Nitrogen Medium. Enzyme Microb. Tech. 27, 631–635. doi:10.1016/S0141-0229(00)00266-0
Nagi, G., Chetry, R., Singh, N., Sinha, A., and Shinde, O. A. (2021). Bioremediation of Coke Plant Wastewater from Steel Industry with Mixed Activated Sludge-Microalgal Consortium in Lab‐scale Semi‐continuous Mode. J. Chem. Technol. Biotechnol. 96, 2249–2256. doi:10.1002/jctb.6749
Kay, R. A., and Barton, L. L. (1991). Microalgae as Food and Supplement. Crit. Rev. Food Sci. Nutr. 30, 555–573. doi:10.1080/10408399109527556
Kazamia, E., Czesnick, H., Nguyen, T. T. V., Croft, M. T., Sherwood, E., Sasso, S., et al. (2012). Mutualistic Interactions between Vitamin B12-dependent Algae and Heterotrophic Bacteria Exhibit Regulation. Environ. Microbiol. 14, 1466–1476. doi:10.1111/j.1462-2920.2012.02733.x
Ketzer, F., Skarka, J., and Rösch, C. (2018). Critical Review of Microalgae LCA Studies for Bioenergy Production. Bioenerg. Res. 11, 95–105. doi:10.1007/s12155-017-9880-1
Khoo, K. S., Lee, S. Y., Ooi, C. W., Fu, X., Miao, X., Ling, T. C., et al. (2019). Recent Advances in Biorefinery of Astaxanthin from Haematococcus pluvialis. Bioresour. Tech. 288, 121606. doi:10.1016/j.biortech.2019.121606
Khrunyk, Y., Lach, S., Petrenko, I., and Ehrlich, H. (2020). Progress in Modern Marine Biomaterials Research. Mar. Drugs 18 (12), 589. doi:10.3390/md18120589
Kim, D., Kim, E. K., Koh, H. G., Kim, K., Han, J.-I., and Chang, Y. K. (2017). Selective Removal of Rotifers in Microalgae Cultivation Using Hydrodynamic Cavitation. Algal Res. 28, 24–29. doi:10.1016/j.algal.2017.09.026
Kim, S., Quiroz-Arita, C., Monroe, E. A., Siccardi, A., Mitchell, J., Huysman, N., et al. (2021). Application of Attached Algae Flow-Ways for Coupling Biomass Production with the Utilization of Dilute Non-point Source Nutrients in the Upper Laguna Madre, TX. Water Res. 191, 116816. doi:10.1016/j.watres.2021.116816
Kondaveeti, S., Abu-Reesh, I. M., Mohanakrishna, G., Bulut, M., and Pant, D. (2020). Advanced Routes of Biological and Bio-Electrocatalytic Carbon Dioxide (CO2) Mitigation toward Carbon Neutrality. Front. Energ. Res. 8, 94. doi:10.3389/fenrg.2020.00094
Koyande, A. K., Chew, K. W., Rambabu, K., Tao, Y., Chu, D.-T., and Show, P.-L. (2019). Microalgae: A Potential Alternative to Health Supplementation for Humans. Food Sci. Hum. Wellness 8, 16–24. doi:10.1016/j.fshw.2019.03.001
Kumar Singh, P., Bhattacharjya, R., Saxena, A., Mishra, B., and Tiwari, A. (2021). Utilization of Wastewater as Nutrient media and Biomass Valorization in marine Chrysophytes- Chaetoceros and Isochrysis. Energy Convers. Manag. X 10, 100062. doi:10.1016/j.ecmx.2020.100062
Lafarga, T., Sánchez‐Zurano, A., Morillas‐España, A., and Acién‐Fernández, F. G. (2021). Extremophile Microalgae as Feedstock for High‐value Carotenoids: A Review. Int. J. Food Sci. Technol. doi:10.1111/ijfs.15069
LaPanse, A. J., Krishnan, A., and Posewitz, M. C. (2021). Adaptive Laboratory Evolution for Algal Strain Improvement: Methodologies and Applications. Algal Res. 53, 102122. doi:10.1016/j.algal.2020.102122
Laurens, L. M. L. (2017). State of Technology Review – Algae Bioenergy. Available at: http://www.ieabioenergy.com/wp-content/uploads/2017/02/IEA-Bioenergy-Algae-report-update-Final-template-20170131.pdf.
Lee, P. A., and White, R. L. (2019). Agronomic Practices for Photoautotrophic Production of Algae Biomass. Grand Challenges Algae Biotechnology,Grand Challenges Biol. Biotechnol., 111–156. doi:10.1007/978-3-030-25233-5_4
Lee, Y.-K. (1997). “Commercial Production of Microalgae in the Asia-Pacific Rim,” in Journal of Applied Phycology (Springer), 9, 403–411. doi:10.1023/A:1007900423275
Levasseur, W., Perré, P., and Pozzobon, V. (2020). A Review of High Value-Added Molecules Production by Microalgae in Light of the Classification. Biotechnol. Adv. 41, 107545. doi:10.1016/j.biotechadv.2020.107545
Li, J., Zhu, D., Niu, J., Shen, S., and Wang, G. (2011). An Economic Assessment of Astaxanthin Production by Large Scale Cultivation of Haematococcus pluvialis. Biotechnol. Adv. 29, 568–574. doi:10.1016/j.biotechadv.2011.04.001
Li, S., Ji, L., Shi, Q., Wu, H., and Fan, J. (2019). Advances in the Production of Bioactive Substances from marine Unicellular Microalgae Porphyridium Spp. Bioresour. Tech. 292, 122048. doi:10.1016/j.biortech.2019.122048
Lin, J.-H., Lee, D.-J., and Chang, J.-S. (2015). Lutein Production from Biomass: Marigold Flowers versus Microalgae. Bioresour. Tech. 184, 421–428. doi:10.1016/j.biortech.2014.09.099
Liu, X., Saydah, B., Eranki, P., Colosi, L. M., Greg Mitchell, B., Rhodes, J., et al. (2013). Pilot-scale Data Provide Enhanced Estimates of the Life Cycle Energy and Emissions Profile of Algae Biofuels Produced via Hydrothermal Liquefaction. Bioresour. Tech. 148, 163–171. doi:10.1016/j.biortech.2013.08.112
Liu, J., Danneels, B., Vanormelingen, P., and Vyverman, W. (2016). Nutrient Removal from Horticultural Wastewater by Benthic Filamentous Algae Klebsormidium sp., Stigeoclonium Spp. And Their Communities: From Laboratory Flask to Outdoor Algal Turf Scrubber (ATS). Water Res. 92, 61–68. doi:10.1016/j.watres.2016.01.049
Liyanaarachchi, V. C., Premaratne, M., Ariyadasa, T. U., Nimarshana, P. H. V., and Malik, A. (2021). Two-stage Cultivation of Microalgae for Production of High-Value Compounds and Biofuels: A Review. Algal Res. 57, 102353. doi:10.1016/j.algal.2021.102353
Lotan, A. (2012). Biologic Sunscreen Composition. Available at: https://patents.google.com/patent/WO2012093388A2/en. [Accessed June 7, 2021].
Mandal, S., Shurin, J. B., Efroymson, R. A., and Mathews, T. J. (2018a). Functional Divergence in Nitrogen Uptake Rates Explains Diversity-Productivity Relationship in Microalgal Communities. Ecosphere 9. doi:10.1002/ecs2.2228
Mandal, S., Shurin, J. B., Efroymson, R. A., and Mathews, T. J. (2018b). Heterogeneity in Nitrogen Sources Enhances Productivity and Nutrient Use Efficiency in Algal Polycultures. Environ. Sci. Technol. 52, 3769–3776. doi:10.1021/acs.est.7b05318
Market Data Forecast 2021 | Industry Reports & Business Intelligence Available at: https://www.marketdataforecast.com/. [Accessed June 4, 2021].
Market Research 2021 Informatics Market Research Reports and Industry Analysis Available at: http://www.marketresearch.com/Life-Sciences-c1594/Biotechnology-c57/Informatics-c63/. [Accessed June 27, 2021].
Mattsson, L., Sörenson, E., Capo, E., Farnelid, H. M., Hirwa, M., Olofsson, M., et al. (2021). Functional Diversity Facilitates Stability under Environmental Changes in an Outdoor Microalgal Cultivation System. Front. Bioeng. Biotechnol. 9, 290. doi:10.3389/fbioe.2021.651895
McBride, R. C., and Merrick, D. S. (2014). Innovations in Open Pond Algae Agriculture for Biofuel Production. Ind. Biotechnol. 10, 162–163. doi:10.1089/ind.2013.1614
McBride, R. C., Lopez, S., Meenach, C., Burnett, M., Lee, P. A., Nohilly, F., et al. (2014). Contamination Management in Low Cost Open Algae Ponds for Biofuels Production. Ind. Biotechnol. 10, 221–227. doi:10.1089/ind.2013.0036
Mitchell, S. A., and Richmond, A. (1987). The Use of Rotifers for the Maintenance of Monoalgal Mass Cultures ofSpirulina. Biotechnol. Bioeng. 30, 164–168. doi:10.1002/bit.260300205
Molino, A., Mehariya, S., Di Sanzo, G., Larocca, V., Martino, M., Leone, G. P., et al. (2020). Recent Developments in Supercritical Fluid Extraction of Bioactive Compounds from Microalgae: Role of Key Parameters, Technological Achievements and Challenges. J. CO2 Utilization 36, 196–209. doi:10.1016/j.jcou.2019.11.014
Morales-Oyervides, L., Oliveira, J., Sousa-Gallagher, M., Méndez-Zavala, A., and Montañez, J. C. (2017). Assessment of the Dyeing Properties of the Pigments Produced by Talaromyces Spp. JoF 3, 38. doi:10.3390/jof3030038
Morocho-Jácome, A. L., Ruscinc, N., Martinez, R. M., de Carvalho, J. C. M., Santos de Almeida, T., Rosado, C., et al. (2020). (Bio)Technological Aspects of Microalgae Pigments for Cosmetics. Appl. Microbiol. Biotechnol. 104, 9513–9522. doi:10.1007/s00253-020-10936-x
Mutanda, T., Naidoo, D., Bwapwa, J. K., and Anandraj, A. (2020). Biotechnological Applications of Microalgal Oleaginous Compounds: Current Trends on Microalgal Bioprocessing of Products. Front. Energ. Res. 8, 299. doi:10.3389/fenrg.2020.598803
Narala, R. R., Garg, S., Sharma, K. K., Thomas-Hall, S. R., Deme, M., Li, Y., et al. (2016). Comparison of Microalgae Cultivation in Photobioreactor, Open Raceway Pond, and a Two-Stage Hybrid System. Front. Energ. Res. 4, 29. doi:10.3389/FENRG.2016.00029
Nauroth, J. M., Liu, Y. C., Van Elswyk, M., Bell, R., Hall, E. B., Chung, G., et al. (2010). Docosahexaenoic Acid (DHA) and Docosapentaenoic Acid (DPAn-6) Algal Oils Reduce Inflammatory Mediators in Human Peripheral Mononuclear Cells In Vitro and Paw Edema In Vivo. Lipids 45, 375–384. doi:10.1007/s11745-010-3406-3
M. U., N., Mehar, J. G., Mudliar, S. N., and Shekh, A. Y. (2019). Recent Advances in Microalgal Bioactives for Food, Feed, and Healthcare Products: Commercial Potential, Market Space, and Sustainability. Compr. Rev. Food Sci. Food Saf. 18, 1882–1897. doi:10.1111/1541-4337.12500
Newby, D. T., Mathews, T. J., Pate, R. C., Huesemann, M. H., Lane, T. W., Wahlen, B. D., et al. (2016). Assessing the Potential of Polyculture to Accelerate Algal Biofuel Production. Algal Res. 19, 264–277. doi:10.1016/j.algal.2016.09.004
Niizawa, I., Espinaco, B. Y., Leonardi, J. R., Heinrich, J. M., and Sihufe, G. A. (2018). Enhancement of Astaxanthin Production from Haematococcus pluvialis under Autotrophic Growth Conditions by a Sequential Stress Strategy. Prep. Biochem. Biotechnol. 48, 528–534. doi:10.1080/10826068.2018.1466159
Noreña-Caro, D., and Benton, M. G. (2018). Cyanobacteria as Photoautotrophic Biofactories of High-Value Chemicals. J. CO2 Utilization 28, 335–366. doi:10.1016/j.jcou.2018.10.008
Nwoba, E. G., Ogbonna, C. N., Ishika, T., and Vadiveloo, A. (2020). “Microalgal Pigments: A Source of Natural Food Colors,” in Microalgae Biotechnology for Food, Health and High Value Products (Springer Singapore), 81–123. doi:10.1007/978-981-15-0169-2_3
Oswald, W. J., and Golueke, C. G. (1960). Biological Transformation of Solar Energy. Adv. Appl. Microbiol. 2, 223–262. doi:10.1016/S0065-2164(08)70127-8
Park, J. B. K., Craggs, R. J., and Shilton, A. N. (2011). Wastewater Treatment High Rate Algal Ponds for Biofuel Production. Bioresour. Tech. 102, 35–42. doi:10.1016/j.biortech.2010.06.158
Pate, R., Klise, G., and Wu, B. (2011). Resource Demand Implications for US Algae Biofuels Production Scale-Up. Appl. Energ. 88, 3377–3388. doi:10.1016/j.apenergy.2011.04.023
Patnaik, R., Singh, N. K., Bagchi, S. K., Rao, P. S., and Mallick, N. (2019). Utilization of Scenedesmus Obliquus Protein as a Replacement of the Commercially Available Fish Meal under an Algal Refinery Approach. Front. Microbiol. 10, 2114. doi:10.3389/fmicb.2019.02114
Pattullo, M. B., Mayes, M. A., Mandal, S., Mathews, T. J., Dunlap, J., Perfect, E., et al. (2019). Soil Sealing by Algae: An Alternative to Plastic Pond Liners for Outdoor Algal Cultivation. Algal Res. 38, 101414. doi:10.1016/j.algal.2019.101414
Pereira, A. G., Otero, P., Echave, J., Carreira-Casais, A., Chamorro, F., Collazo, N., et al. (2021). Xanthophylls from the Sea: Algae as Source of Bioactive Carotenoids. Mar. Drugs 19, 188. doi:10.3390/md19040188
Phung Hai, T. A., Neelakantan, N., Tessman, M., Sherman, S. D., Griffin, G., Pomeroy, R., et al. (2020). Flexible Polyurethanes, Renewable Fuels, and Flavorings from a Microalgae Oil Waste Stream. Green. Chem. 22, 3088–3094. doi:10.1039/d0gc00852d
Posadas, E., Moralesdel, M. d. M. M., Gomez, C., Acién, F. G., and Muñoz, R. (2015). Influence of pH and CO2 Source on the Performance of Microalgae-Based Secondary Domestic Wastewater Treatment in Outdoors Pilot Raceways. Chem. Eng. J. 265, 239–248. doi:10.1016/j.cej.2014.12.059
Pulz, O., and Gross, W. (2004). Valuable Products from Biotechnology of Microalgae. Appl. Microbiol. Biotechnol. 65, 635–648. doi:10.1007/s00253-004-1647-x
Qu, F., Jin, W., Zhou, X., Wang, M., Chen, C., Tu, R., et al. (2020). Nitrogen Ion Beam Implantation for Enhanced Lipid Accumulation of Scenedesmus Obliquus in Municipal Wastewater. Biomass and Bioenergy 134, 105483. doi:10.1016/j.biombioe.2020.105483
Rahman, K. M. (2020). Food and High Value Products from Microalgae: Market Opportunities and Challenges. In Microalgae Biotechnology For Food, Health And High Value Products. Springer Singapore, 3–27. doi:10.1007/978-981-15-0169-2_1
Rajvanshi, M., and Sayre, R. (2020). Recent Advances in Algal Biomass Production, 0–36. London, United Kingdom: IntechOpen. doi:10.5772/INTECHOPEN.94218
Randhir, A., Laird, D. W., Maker, G., Trengove, R., and Moheimani, N. R. (2020). Microalgae: A Potential Sustainable Commercial Source of Sterols. Algal Res. 46, 101772. doi:10.1016/j.algal.2019.101772
Randrianarison, G., and Ashraf, M. A. (2017). Microalgae: a Potential Plant for Energy Production. Geology. Ecology, Landscapes 1, 104–120. doi:10.1080/24749508.2017.1332853
Rao, A., Briskey, D., Nalley, J. O., and Ganuza, E. (2020). Omega-3 Eicosapentaenoic Acid (Epa) Rich Extract from the Microalga Nannochloropsis Decreases Cholesterol in Healthy Individuals: A Double-Blind, Randomized, Placebo-Controlled, Three-Month Supplementation Study. Nutrients 12 (6), 1869. doi:10.3390/nu12061869
Rastogi, R. P., Sonani, R. R., Madamwar, D., and Incharoensakdi, A. (2016). Characterization and Antioxidant Functions of Mycosporine-like Amino Acids in the Cyanobacterium Nostoc Sp. R76DM. Algal Res. 16, 110–118. doi:10.1016/j.algal.2016.03.009
Ratledge, C. (2010). “Single Cell Oils for the 21st Century,” in Single Cell Oils Microb. Second Ed (Urbana, IL: Algal Oils), 3–26. doi:10.1016/B978-1-893997-73-8.50005-0
Reese, K. L., Fisher, C. L., Lane, P. D., Jaryenneh, J. D., Moorman, M. W., Jones, A. D., et al. (2019). Chemical Profiling of Volatile Organic Compounds in the Headspace of Algal Cultures as Early Biomarkers of Algal Pond Crashes. Sci. Rep. 9, 1–10. doi:10.1038/s41598-019-50125-z
Richmond, A., Lichtenberg, E., Stahl, B., and Vonshak, A. (1990). Quantitative Assessment of the Major Limitations on Productivity of Spirulina Platensis in Open Raceways. J. Appl. Phycol. 2, 195–206. doi:10.1007/BF02179776
Ritala, A., Häkkinen, S. T., Toivari, M., and Wiebe, M. G. (2017). Single Cell Protein-State-Of-The-Art, Industrial Landscape and Patents 2001-2016. Front. Microbiol. 8, 2009. doi:10.3389/fmicb.2017.02009
Roccuzzo, S., Couto, N., Karunakaran, E., Kapoore, R. V., Butler, T. O., Mukherjee, J., et al. (2020). Metabolic Insights into Infochemicals Induced Colony Formation and Flocculation in Scenedesmus Subspicatus Unraveled by Quantitative Proteomics. Front. Microbiol. 11, 792. doi:10.3389/fmicb.2020.00792
Ruiz, J., Olivieri, G., De Vree, J., Bosma, R., Willems, P., Reith, J. H., et al. (2016). Towards Industrial Products from Microalgae. Energy Environ. Sci. 9, 3036–3043. doi:10.1039/c6ee01493c
Ruiz-Rodriguez, A., Reglero, G., and Ibañez, E. (2010). Recent Trends in the Advanced Analysis of Bioactive Fatty Acids. J. Pharm. Biomed. Anal. 51, 305–326. doi:10.1016/j.jpba.2009.05.012
Ryckebosch, E., Bruneel, C., Termote-Verhalle, R., Goiris, K., Muylaert, K., and Foubert, I. (2014). Nutritional Evaluation of Microalgae Oils Rich in omega-3 Long Chain Polyunsaturated Fatty Acids as an Alternative for Fish Oil. Food Chem. 160, 393–400. doi:10.1016/j.foodchem.2014.03.087
Saad, M. G., Dosoky, N. S., Zoromba, M. S., and Shafik, H. M. (2019). Algal Biofuels: Current Status and Key Challenges. Energies 12, 1920. doi:10.3390/en12101920
Saini, R. K., and Keum, Y.-S. (2018). Carotenoid Extraction Methods: A Review of Recent Developments. Food Chem. 240, 90–103. doi:10.1016/j.foodchem.2017.07.099
Sato, T., Qadir, M., Yamamoto, S., Endo, T., and Zahoor, A. (2013). Global, Regional, and Country Level Need for Data on Wastewater Generation, Treatment, and Use. Agric. Water Manage. 130, 1–13. doi:10.1016/j.agwat.2013.08.007
Schipper, K., Al-Jabri, H. M. S. J., Wijffels, R. H., and Barbosa, M. J. (2021). Techno-economics of Algae Production in the Arabian Peninsula. Bioresour. Tech. 331, 125043. doi:10.1016/j.biortech.2021.125043
Schultz, H. BGG to Double Capacity at Astaxanthin Farm in Southwestern China. 2020. Available at: https://www.nutraingredients-usa.com/Article/2020/04/22/BGG-to-double-capacity-at-astaxanthin-farm-in-southwestern-China?utm_source=copyright&utm_medium=OnSite&utm_campaign=copyright. [Accessed June 27, 2021].
Sero, E. T., Siziba, N., Bunhu, T., and Shoko, R. (2021). Isolation and Screening of Microalgal Species, Native to Zimbabwe, with Potential Use in Biodiesel Production. All Life 14, 256–264. doi:10.1080/26895293.2021.1911862
Shukla, M., and Kumar, S. (2018). Algal Biorefineries for Biofuels and Other Value-Added Products). 305–341. doi:10.1007/978-3-319-67678-4_14
Shurin, J. B., Abbott, R. L., Deal, M. S., Kwan, G. T., Litchman, E., Mcbride, R. C., et al. (2013). Industrial-strength Ecology: Trade-Offs and Opportunities in Algal Biofuel Production. Ecol. Lett. 16, 1393–1404. doi:10.1111/ele.12176
Silva, S. C., Ferreira, I. C. F. R., Dias, M. M., and Barreiro, M. F. (2020). Microalgae-derived Pigments: A 10-year Bibliometric Review and Industry and Market Trend Analysis. Molecules 25, 3406. doi:10.3390/molecules25153406
Simkovsky, R., Daniels, E. F., Tang, K., Huynh, S. C., Golden, S. S., and Brahamsha, B. (2012). Impairment of O-Antigen Production Confers Resistance to Grazing in a Model Amoeba-Cyanobacterium Predator-Prey System. Proc. Natl. Acad. Sci. 109, 16678–16683. doi:10.1073/pnas.1214904109
Smith, V. H., and Crews, T. (2014). Applying Ecological Principles of Crop Cultivation in Large-Scale Algal Biomass Production. Algal Res. 4, 23–34. doi:10.1016/j.algal.2013.11.005
Smith, V. H., Sturm, B. S. M., deNoyelles, F. J., and Billings, S. A. (2010). The Ecology of Algal Biodiesel Production. Trends Ecol. Evol. 25, 301–309. doi:10.1016/j.tree.2009.11.007
Spolaore, P., Joannis-Cassan, C., Duran, E., and Isambert, A. (2006). Commercial Applications of Microalgae. J. Biosci. Bioeng. 101, 87–96. doi:10.1263/jbb.101.87
Stephens, E., Ross, I. L., King, Z., Mussgnug, J. H., Kruse, O., Posten, C., et al. (2010). An Economic and Technical Evaluation of Microalgal Biofuels. Nat. Biotechnol. 28, 126–128. doi:10.1038/nbt0210-126
Suarez Ruiz, C. A., Emmery, D. P., Wijffels, R. H., Eppink, M. H., and van den Berg, C. (2018). Selective and Mild Fractionation of Microalgal Proteins and Pigments Using Aqueous Two-phase Systems. J. Chem. Technol. Biotechnol. 93, 2774–2783. doi:10.1002/jctb.5711
Sun, Z., Wang, X., and Liu, J. (2019). Screening of Isochrysis Strains for Simultaneous Production of Docosahexaenoic Acid and Fucoxanthin. Algal Res. 41, 101545. doi:10.1016/j.algal.2019.101545
Szyjka, S. J., Mandal, S., Schoepp, N. G., Tyler, B. M., Yohn, C. B., Poon, Y. S., et al. (2017). Evaluation of Phenotype Stability and Ecological Risk of a Genetically Engineered Alga in Open Pond Production. Algal Res. 24, 378–386. doi:10.1016/j.algal.2017.04.006
Tanticharoen, M., Reungjitchachawali, M., Boonag, B., Vonktaveesuk, P., Vonshak, A., and Cohen, Z. (1994). Optimization of γ-linolenic Acid (GLA) Production inSpirulina Platensis. J. Appl. Phycol. 6, 295–300. doi:10.1007/BF02181942
Vadlamani, A., Viamajala, S., Pendyala, B., and Varanasi, S. (2017). Cultivation of Microalgae at Extreme Alkaline pH Conditions: A Novel Approach for Biofuel Production. ACS Sust. Chem. Eng. 5, 7284–7294. doi:10.1021/acssuschemeng.7b01534
Varshney, P., Mikulic, P., Vonshak, A., Beardall, J., and Wangikar, P. P. (2015). Extremophilic Micro-algae and Their Potential Contribution in Biotechnology. Bioresour. Tech. 184, 363–372. doi:10.1016/j.biortech.2014.11.040
Wang, X., and Zhang, X. (2013). Separation, Antitumor Activities, and Encapsulation of Polypeptide from Chlorella Pyrenoidosa. Biotechnol. Prog. 29, 681–687. doi:10.1002/btpr.1725
Wang, A., Yan, K., Chu, D., Nazer, M., Lin, N. T., Samaranayake, E., et al. (2020). “Microalgae as a Mainstream Food Ingredient: Demand and Supply Perspective,” in Microalgae Biotechnology for Food, Health and High Value Products (Springer Singapore), 29–79. doi:10.1007/978-981-15-0169-2_2
Whalon, M. E., Mota-Sanchez, D., and Hollingworth, R. M. (2008). “Analysis of Global Pesticide Resistance in Arthropods,” in Global Pesticide Resistance in Arthropods (Wallingford, Oxfordshire, United Kingdom: CABI Publishing), 5–31. doi:10.1079/9781845933531.0005
WijffelsWijffels, R. H. J. M. B., and Barbosa, M. J. (2010). An Outlook on Microalgal Biofuels. Science 329, 796–799. doi:10.1126/science.1189003
Wiley, P., Harris, L., Reinsch, S., Tozzi, S., Embaye, T., Clark, K., et al. (2013). Microalgae Cultivation Using Offshore Membrane Enclosures for Growing Algae (OMEGA). Jsbs 03, 18–32. doi:10.4236/jsbs.2013.31003
Winckelmann, D., Bleeke, F., Thomas, B., Elle, C., and Klöck, G. (2015). Open Pond Cultures of Indigenous Algae Grown on Non-arable Land in an Arid Desert Using Wastewater. Int. Aquat. Res. 7, 221–233. doi:10.1007/s40071-015-0107-9
Wirth, R., Pap, B., Böjti, T., Shetty, P., Lakatos, G., Bagi, Z., et al. (2020). Chlorella Vulgaris and its Phycosphere in Wastewater: Microalgae-Bacteria Interactions during Nutrient Removal. Front. Bioeng. Biotechnol. 8, 1108. doi:10.3389/fbioe.2020.557572
Wood, D. A. (2021). Microalgae to Biodiesel - Review of Recent Progress. Bioresour. Tech. Rep. 14, 100665. doi:10.1016/j.biteb.2021.100665
Wynn, J., Behrens, P., Sundararajan, A., Hansen, J., and Apt, K. (2010). “Production of Single Cell Oils by Dinoflagellates,” in Single Cell Oils: Microbial and Algal Oils. Second Edition (Elsevier), 115–129. doi:10.1016/B978-1-893997-73-8.50010-4
Yao, S., Lyu, S., An, Y., Lu, J., Gjermansen, C., and Schramm, A. (2019). Microalgae-bacteria Symbiosis in Microalgal Growth and Biofuel Production: a Review. J. Appl. Microbiol. 126, 359–368. doi:10.1111/jam.14095
Yates, C. M., Calder, P. C., and Ed Rainger, G. (2014). Pharmacology and Therapeutics of omega-3 Polyunsaturated Fatty Acids in Chronic Inflammatory Disease. Pharmacol. Ther. 141, 272–282. doi:10.1016/j.pharmthera.2013.10.010
Yi, X., Li, J., Xu, W., Zhou, H., Smith, A. A., Zhang, W., et al. (2015). Shrimp Shell Meal in Diets for Large Yellow Croaker Larimichthys Croceus: Effects on Growth, Body Composition, Skin Coloration and Anti-oxidative Capacity. Aquaculture 441, 45–50. doi:10.1016/j.aquaculture.2015.01.030
Younes, G., Rasoul-Amini, S., and Morowvat, M. H. (2011). Algae for the Production of SCP. Bioproc. Sci. Tech., 163–184.
Keywords: biofuels, biorefinery, grazers, microalgae, integrated pest management
Citation: Nagi GK, Minhas AK, Gaur S, Jain P and Mandal S (2021) Integration of Algal Biofuels With Bioremediation Coupled Industrial Commodities Towards Cost-Effectiveness. Front. Energy Res. 9:735141. doi: 10.3389/fenrg.2021.735141
Received: 02 July 2021; Accepted: 11 August 2021;
Published: 25 August 2021.
Edited by:
Héctor A. Ruiz, Universidad Autónoma de Coahuila, MexicoReviewed by:
Vijay Kumar Garlapati, Jaypee University of Information Technology, IndiaCopyright © 2021 Nagi, Minhas, Gaur, Jain and Mandal. This is an open-access article distributed under the terms of the Creative Commons Attribution License (CC BY). The use, distribution or reproduction in other forums is permitted, provided the original author(s) and the copyright owner(s) are credited and that the original publication in this journal is cited, in accordance with accepted academic practice. No use, distribution or reproduction is permitted which does not comply with these terms.
*Correspondence: Shovon Mandal, c2hvdm9uLm1hbmRhbF9jQHRlcmkucmVzLmlu
Disclaimer: All claims expressed in this article are solely those of the authors and do not necessarily represent those of their affiliated organizations, or those of the publisher, the editors and the reviewers. Any product that may be evaluated in this article or claim that may be made by its manufacturer is not guaranteed or endorsed by the publisher.
Research integrity at Frontiers
Learn more about the work of our research integrity team to safeguard the quality of each article we publish.