- 1School of Energy and Power Engineering, University of Shanghai for Science and Technology, Shanghai, China
- 2China Aviation Technology Hangzhou Co., Ltd., Hangzhou, China
- 3Environment and Sustainability Institute, University of Exeter, Penryn, United Kingdom
- 4China-UK Low Carbon College, Shanghai Jiao Tong University, Shanghai, China
Composites composed of paraffin wax and copper foam with porosity of 95 % and pore density of 15 ppi, 30 ppi, and 50 ppi were prepared by the melt impregnation method. Two building models of the same size were made using gypsum board. The roof of the experimental model was covered with 50ppi copper foam /paraffin composite phase change board, and the roof of the reference model was covered with foam insulation board. The heat transfer experiment was conducted with a constant heat flux. EnergyPlus software was also employed to simulate the energy saving effects of the building models. The size and material of the model established during the simulation are consistent with the experimental model. Experimental results showed that the phase change composite board delayed the highest indoor temperature for 1.5 h, and it also reduced the indoor maximum temperature by 1.2° and the indoor energy consumption by 21 %. The simulation results showed that the phase change composites board delayed the appearance of the highest indoor temperature by 1.3 h and reduced the highest indoor temperature by 1.1°C, and the maximum indoor energy consumption decreased by 19 %. The copper foam/paraffin composite material can effectively improve the thermal comfort and reduce the energy consumption of the building model.
Introduction
Phase change materials (PCMs) absorb or release large amounts of latent heat during phase transitions, thereby they are widely used in building energy saving, indoor warming, temperature adjustable textiles, military, and aerospace, etc. (Du et al., 2018; Zhang et al., 2018; Koohi-Fayegh and Rosen, 2020). Phase change heat storage materials mainly include inorganic phase change materials and organic phase change materials. Inorganic materials are mainly crystalline water and salts, molten salts, metals and alloys, etc. Inorganic materials have high heat storage density, fixed melting point, higher thermal conductivity, and they are generally neutral (Crespo et al., 2019; Lu et al., 2020), such as CaCl2 6H2O, Na2CO3 10H2O, NaSO4 5H2O. Crystal water and salts also suffer from problems such as supercooling and phase separation, affecting their widespread applications (Xu et al., 2018). Metals and metal alloys usually have high phase transition temperatures, which are characterized by large thermal conductivity and fast heat storage and exothermic speeds (Violidakis et al., 2020). Organic materials are mainly paraffin, fatty acids, and polyols (Wei et al., 2016). Compared with inorganic materials, organic materials have better molding effect during curing, and generally do not appear supercooling and phase separation phenomena. In addition, organic materials have stable performance, low toxicity and low cost. However, there are also problems in organic materials such as low thermal conductivity, small energy storage density, and easy volatility (Kenisarin, 2014; Liu et al., 2019; Qu et al., 2020; Duan et al., 2021).
Low thermal conductivity, slow heat storage and release rates limit the practical applications of PCMs. Therefore, intensive investigations have been carried out to improve the thermal conductivity of PCMs (Zhao et al., 2013; Badenhorst, 2019). Li et al. (2017) mixed the expanded graphite (EG) with capric acid (DA) to prepare a capric acid / expanded graphite composite phase change material (DA/EG-PCMS) with high stability and good thermal conductivity, the thermal conductivity of composites increased with the increase of expanded graphite. Zeng et al. (2008) added Ag nanoparticles to myristyl alcohol, and the thermal conductivity increased with the increase of Ag nanoparticles, and the phase transition temperature and latent heat decreased slightly. Wu et al. (2016) prepared nanocomposite PCM by mixing paraffin with multi-walled carbon nanotubes (MWCNTs). The results showed that as the loading of carbon nanofillers increased, the thermal conductivity of the nanocomposite PCM increased, and the phase change enthalpy gradually decreased. Yang et al. (2016) compounded copper foam and bottom fins into PCM in order to improve the heat transfer performance. High temperature water flowed through the copper tube as a heat transfer fluid (HTF). The temperature variations of the selected detected points inside PCM and the solid–liquid interface evolution in axial plane of symmetry for three samples, including that of pure paraffin, paraffin–copper foam composites without and with the bottom fin, were recorded under the different heating temperatures and flow rates of HTF. The experimental results showed that under the same operating conditions, the time required to completely melt the PCM in the composite material was 1/3 less than that of pure paraffin. Yang et al. (2019) designed a visual experiment test bench to investigate the influence of metal foam on the melting process of paraffin wax. Experimental results showed that the open cell metal foam could significantly improve the efficiency of thermal energy storage. Compared with pure PCM, the complete melting time of composite PCM was reduced by 64 %, and the temperature distribution was more uniform.
Nowadays, the energy consumption of buildings accounts for about 32 % of the total energy consumption, and the greenhouse gas emissions of commercial buildings and residential buildings have reached 30 % of the total emissions. Therefore, achieving building energy conservation has become the main goal of energy policies at home and abroad, and it is of great significance to alleviate the energy crisis and reduce environmental pollution (Uerge-Vorsatz et al., 2015). The application of heat storage technology in building energy conservation mainly has the following aspects. Firstly, low temperature heat storage materials are used for cold storage and adjusting the load of air conditioning, such as ice or water cold storage technology (Mohandes et al., 2020). Secondly, adding phase change materials to the building materials to increase the thermal inertia of the building and reduce the temperature fluctuations of the room, thereby reducing the air conditioning or heating load, and achieving the purpose of building energy saving. For instance, phase change materials are combined with building envelopes or building materials and applied to walls, floors, ceilings, and concrete (Zhou et al., 2012; Zhu et al., 2015; Fu et al., 2017; Saafi and Daouas, 2019). Thirdly, using phase change materials to absorb heat for heating, such as active or passive solar greenhouses often use phase change materials with a phase change temperature of 50–60°C (Xie et al., 2018).
In summary, phase change materials have great thermal storage and thermal insulation properties. Absorbing a large amount of heat through phase change can reduce indoor temperature fluctuations, thereby improving the comfort of the building and reducing the indoor energy consumption of the building. However, traditional organic phase change materials have low thermal conductivity and poor curing and molding effects. The traditional way of adding metal particles and nanomaterials to enhance the thermal conductivity will cause aggregation and precipitation after multiple melting and solidification cycles, and the application effect is poor as well. The emerging metal foam materials have the characteristics of light weight, high thermal conductivity, great skeleton structure and sound absorption, etc. Therefore, this article chooses to fill the organic phase change material paraffin wax into the foamed copper with a porous framework structure, which can not only improve the thermal conductivity of the composite material, but also facilitate the preparation of a fixed shape material for use in construction. This paper tested the thermal conductivity of copper foam /paraffin composites with the same porosity and different pore density. Experimental and numerical investigations were carried out to study the application of copper foam /paraffin phase change composite materials in building energy conservation. The aim of this paper is to investigate the indoor temperature control and energy saving effects of PCM composites in building models. It is hoped that greenhouse gas emissions from building energy consumption can be reduced by improving building materials.
Methodology
Three kinds of phase change materials composed of paraffin wax and copper foam with porosity of 95 % and pore density of 15 ppi, 30 ppi, and 50 ppi were fabricated by the melt impregnation method. The effect of copper foam on the thermal conductivity of composite materials was investigated. The heat transfer experiment was conducted using two cubic building models (PCM building model and reference building model) with the same dimension and a constant heat flux, respectively. The effects of copper foam/paraffin composite phase change materials on the building energy consumption of the building models were experimentally and numerically explored.
Experimental Approach
Preparation of Composite Materials
Considering the comfort of the building, this experiment chose a paraffin wax with a melting point of 27 °C produced by Shanghai Joule wax Co. Ltd, and its thermal stability was tested through repeated melting and solidification. The paraffin sample was melted in a constant temperature water bath at 70°C, and cooled in a refrigerator at 0°C. The sample was repeatedly melted and solidified by this method 50 cycles. The sample was tested for thermal properties using a differential scanning calorimeter (DSC). As shown in Figure 1, the test found that the melting point of paraffin wax is around 27°C, and the latent heat of fusion has not changed significantly after 50 cycles.
The phase change material is evenly filled into the voids of the copper foam skeleton to prepare a composite material, thereby improving the thermal conductivity of the phase change material and its curing molding effect. In this experiment, copper foam with a porosity of 95 %, pore densities of 15 ppi, 30 ppi, and 50 ppi, and paraffin wax of 27°C were selected to fabricated composite phase change materials respectively, as shown in Figure 2.
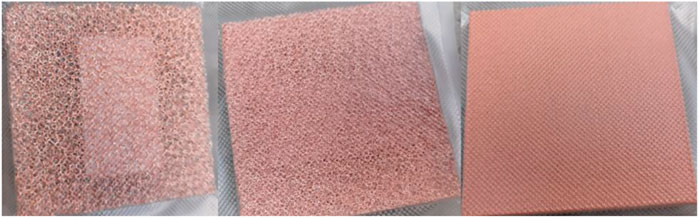
FIGURE 2. Copper foam with the same porosity of 95 % and various pore densities of 15 ppi, 30 ppi, and 50 ppi (from left to right).
As shown in the flowchart of Figure 3. Copper foam samples with a porosity of 95 % and pore densities of 15 ppi, 30 ppi, and 50 ppi were completely immersed in the molten paraffin liquid and placed in a refrigerator to solidify, respectively. It removed sample after it has completely solidified and removed excess paraffin by the cutting process. The prepared copper foam/paraffin composite phase change material is shown in Figure 4.
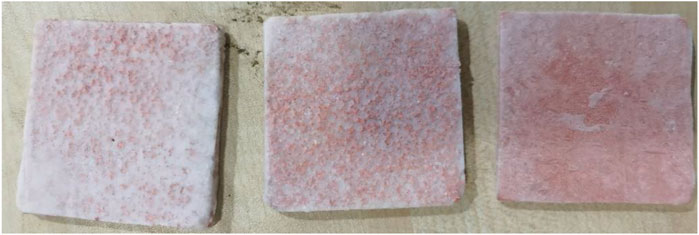
FIGURE 4. Three kinds of copper foam/paraffin PCM composites prepared with various pore densities of 15 ppi, 30 ppi, and 50 ppi (from left to right).
Thermal Conductivity of Composite Materials
In the experiment, the thermal conductivity instrument produced by Nanjing Dazhan Mechanical and Electrical Co., Ltd was employed to conduct thermal conductivity tests on 27°C paraffin, 15 ppi copper foam/paraffin, 30 ppi copper foam/paraffin, and 50 ppi copper foam/paraffin samples. The test principle is the transient plane heat source method. During the test, the nickel metal probe was clamped between two samples, the smooth surface of the sample was in contact with the probe, and two samples were clamped to reduce the contact thermal resistance. The average value of the five times thermal conductivity test at 27°C is 0.22 W/( m K), the test interval is 5 min between each test. The thermal conductivity of the composite material with 15 ppi copper foam is 0.79 W/( m K), and the thermal conductivity with 30 ppi copper foam is 1.20 W/( m K). After adding 50 ppi copper foam, the thermal conductivity of composites is 1.34 W/( m K). As shown in Figure 5, the thermal conductivity of paraffin wax can be significantly improved after the addition of copper foam. As the pore density of copper foam increases, the thermal conductivity also increases, but the increase rate decreases.
In summary, the addition of copper foam can significantly increase the thermal conductivity of paraffin wax. Under the same porosity condition, as the pore density of copper foam increases, the thermal conductivity also increases, which is 3.59–6 times higher than that of pure paraffin wax. This is because as the pore density increases, the pore size of the copper foam decreases, and the diameter of the metal skeleton decreases, but the skeleton becomes dense, which facilitates heat conduction.
Experimental Apparatus and Method
Numerous phase change materials have been combined with building envelopes or building materials, such as walls, windows, ceilings, and concrete (de Gracia and Cabeza, 2015; Navarro et al., 2016; Amaral et al., 2020; Jouhara et al., 2020; Wang et al., 2020). The cooling and heating load of the roof and the south exterior wall for the building envelope are the highest. When the south exterior wall is placed, the phase change material is more likely to leak under the action of gravity, thereby the experiment chose to place the phase change material on the roof. Two cubic building models with windows and the same dimension were fabricated using gypsum board and the solar light was simulated by a constant heat flux. A composite phase change board of 50 ppi copper foam and paraffin was applied on the roof of the PCM building model, and a thermal insulation foam board was installed on the roof of the reference building model.
Three copper foams with the same porosity increase in weight and price as the pore density increases. Considering to apply it to building materials, 50 ppi copper foam/paraffin was selected for the heat transfer experiment. The paraffin wax and copper foam were made into composite materials by melt impregnation. The roof of the PCM model utilised a composite phase change board of 50 ppi metal foam and paraffin, and the roof of the reference model utilised an insulation foam board with a size of 300 × 300 × 5 mm. Phase change board and insulation foam board were sealed with tin foil to prevent leakage of phase change materials. Phase change heat storage insulation board and insulation foam board are shown in Figure 6. The size of the building model is 300 × 300 × 300 mm. The wall is composed of two gypsum boards. The south exterior wall has double-glazed windows with a size of 200 × 100 mm as shown in Figure 7. The roofs of the two models were covered with a composite phase change board and the other with a foam insulation board.
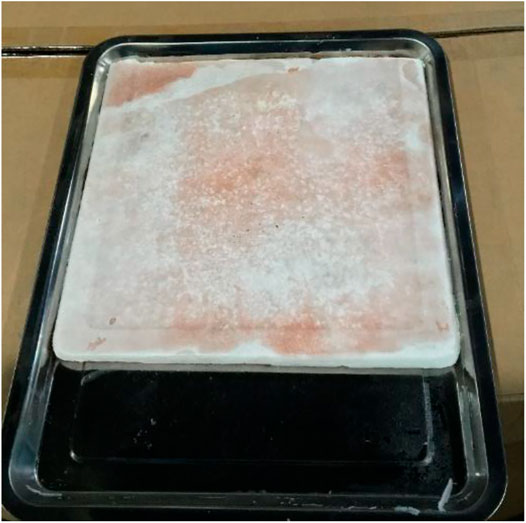
FIGURE 6. PCM composite board made of copper foam/paraffin with pore densities of 50 ppi and 300 × 300 × 5 mm.
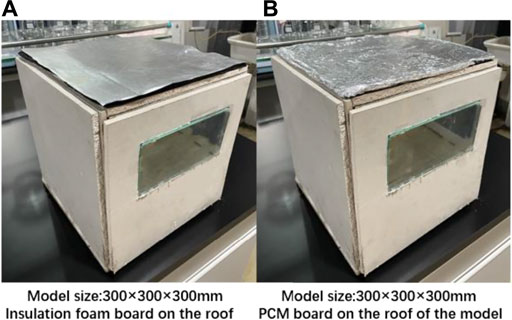
FIGURE 7. Two cubic building models with windows made of gypsum board: (A) with insulation foam on the roof, (B) with PCM board on the roof.
During the experiment, a stable heat source was applied to simulate the sunlight, with a constant heat flux of 800 W. The data acquisition board was employed for temperature acquisition during heating, and the acquisition frequency is every 5 s. Each building model has 1 measuring point outside and six measuring points inside. The external measurement point is located at the centre of the roof, and the six internal measurement points are located at the centre points of the east, west, south, north, floor and ceiling. The building models were placed in a well-insulated box, and the radiant heat source was directly face to the south wall which has a double-glazed window. The temperature data collected by the thermocouple generates a curve in real time on the computer, and the experiment is stopped when the temperature at each point becomes constant. The temperature collection process continually lasted for 6 hours. The flow chart of the test bench is shown in Figure 8. During the experiment, the temperature change inside the model will also be affected by the humidity change, but the humidity change is small and ignored. Due to the small experimental model, the wet load, lighting load, personnel load and envelope load (the gap of doors and windows) were ignored in the energy consumption calculation.
Simulation Method
In this paper, EnergyPlus 9.0.1 was employed to analyse the effect of phase change materials on the indoor temperature and energy consumption of the building model. In order to compare with the experimental results, the size and material of the model established during the simulation are consistent with the experimental model. The simulated space is a cubic building model with a double-glazed window, which is drawn by Sketchup 2018. As shown in Figure 9, the size of the building model is 300 × 300 × 300 mm. The weather file of Shanghai in China (CHN_Shanghai.Shanghai.583620_SWERA) was applied to simulate the building models. During the simulation, the indoor humidity was also set to be consistent with the weather file. The simulation span is 1 year, and the simulation objects are the temperature and energy consumption in the cubic model. The roof of the PCM building model utilised a 10 mm gypsum board and a 5 mm copper foam/paraffin composite phase change board. The reference building model employed a 10 mm gypsum board and a 5 mm insulation foam board on the roof. The other surfaces are made of adiabatic materials with low thermal conductivity, high density, and high specific heat capacity, neglecting the impact of these on the space heat transfer. The south wall of the building model has a 200 × 100 mm window with double-layer insulating glass. The thermophysical properties of materials are shown in Table 1. Consistent with the experimental model, the wet load, lighting load, personnel load and envelope load (the gap of doors and windows) were ignored in the simulation as well.
EnergyPlus utilised CondFD’s algorithm to simulate the heat transfer of PCM. According to the first-order fully implicit differential heat transfer Eq. 1, the temperature of each node at different times can be calculated iteratively. The variable material enthalpy-temperature function and the node temperature calculated by each iteration are derived according to Eq. 2, and the Cp obtained is also continuously updated with the node temperature. In the CondFD algorithm, each layer of material is divided into multiple micromolecular layers according to the algorithm’s time step (Δt) and the thermal diffusion coefficient of material, and the thickness of the micromolecule layer Δx can be calculated according to Eq. 3. The number of time steps per hour for the simulation is 20, Δt is 180 s, C is the spatial dispersion constant, and the default value is 3.
Where:
In order to validate the simulation model, it compared the experimental results with the simulation results. As the simulation considered outdoor weather and the experimental process simulated solar light in a closed space, the current research intends to compare the effects of temperature suppression and the energy consumption reduction between the simulation and experiment. As shown in Figure 10, this paper compared the experimental and simulated results to verify the reliability of EnergyPlus software. Figure 10A shows the heating process of the model with phase change. The red curve is the experimental value, and the black curve is the simulated value. The heating process is basically the same, and the maximum temperature difference in the process is 1.2°C. It is a process in which the temperature rises rapidly first, then the endothermic rise rate of the phase change material melts and becomes slower, and finally the temperature rises rapidly to the peak. Because the ordinate value is 24°C-30°C, the distance between the two curves is relatively long. Figure 10B is a model without phase change. The red curve is the experimental value, and the black curve is the simulated value. Compared with Figure 10A, the process of slowing down the melting endothermic rise of the phase change material is missing. The maximum temperature difference between the experimental value and the simulated value of the building model with phase change layer is 1.2°C, and the maximum temperature error is 4.3 %. The maximum temperature difference between the experimental value and the simulated value of the building model without phase change layer is 0.9°C, and the maximum temperature error is 3.2 %. The reason for the error may be that the boundary conditions of the light source and the humidity environment are different, but the error is relatively small. The error is less than 5%。Therefore, the building model developed by the EnergyPlus can be employed for thermal and energy performance analysis.
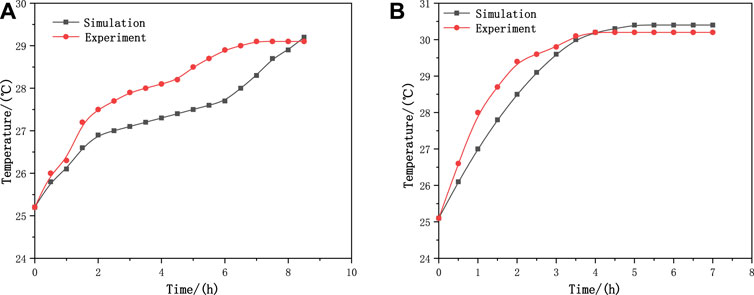
FIGURE 10. Experimental vs simulation results of indoor air temperature: with phase change (A) and without phase change (B).
Results and Discussion
Experiment
The temperature change curves of the inner roof, south wall, east wall, west wall, and north wall of the building model with and without phase change are shown in Figures 11–15.
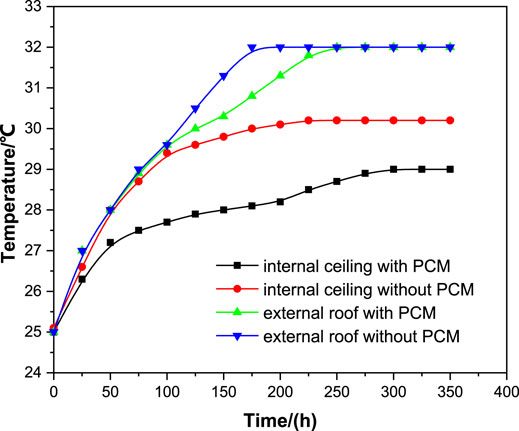
FIGURE 11. Temperature change curves of the internal ceiling and the external roof of the building model with and without phase change.
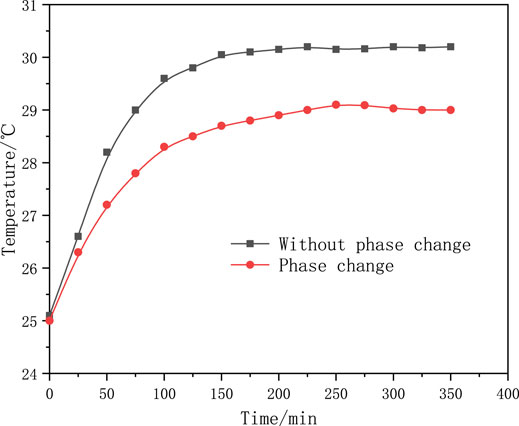
FIGURE 12. Temperature change curves of internal south wall of the building model with and without phase change.
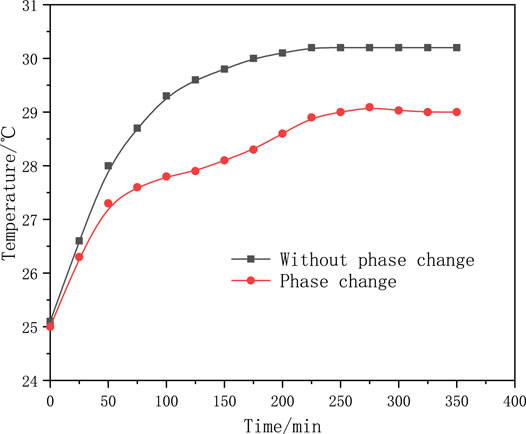
FIGURE 13. Temperature change curves of internal east wall of the building model with and without phase change.
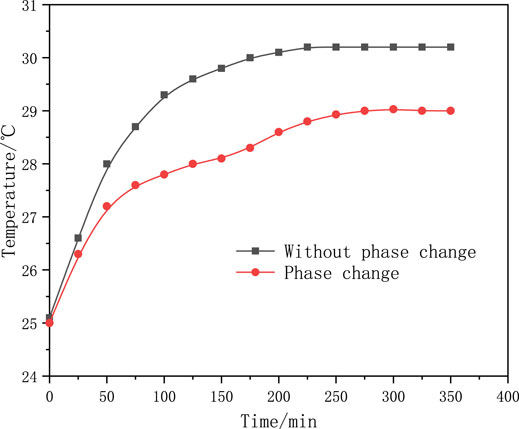
FIGURE 14. Temperature change curves of internal west wall of the building model with and without phase change.
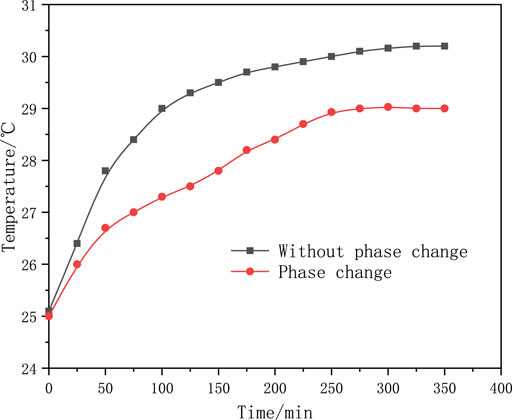
FIGURE 15. Temperature change curves of internal north wall of the building model with and without phase change.
Figure 11 is the temperature change curves of the internal ceiling and external roof of the building models with and without phase change materials. The comparison found that the curve of each acquisition point of the external roof of the PCM model and the reference model was basically the same, indicating that the heating conditions of the two models were basically the same during the experiment. However, there is obvious lag in the temperature rise of the PCM model due to the melting process of the phase change material. It can be seen that the internal temperature change of the PCM model rises to 26.5 °C and begins to decrease. At this time, the phase change material absorbs heat and melts. As the heat transfer of the phase change material still exists during the melting process, and the heat transfer process of the other walls have not changed, the indoor temperature of the building model will continue to increase. However, compared with the reference model without phase transition, the temperature change was significantly reduced. The results show that compared with the traditional thermal insulation foam board, the roof heat storage insulation board made of copper foam/paraffin delayed the appearance of the highest indoor temperature by 1.5 h, and it reduced the highest indoor temperature by 1.2°C.
Figure 12 is the temperature change curve of the south wall inside the building model. As the south wall of the building model has a double-glazed window and the radiant heat source is located in the south of the building model, the temperature change curves with and without phase change are relatively close to each other. Figures 13, 14 are the temperature change curves of the east and west walls of the building model, respectively. As the heating conditions of the east and west walls are similar, the temperature change curves are basically the same. Compared with the south wall, the temperature growth rate of the east and west walls is slower. Figure 15 is the temperature change curves of the north wall inside the building model, which is equivalent to the shaded surface of the actual building, thereby as the outside temperature increases, the temperature rise rate of the north wall is the slowest. Figure 16 is a comparison of temperature changes at the centre of the floor inside the building model with or without phase change materials.
According to the requirements of thermal comfort and in the design of the central air conditioning system, for general civil buildings, such as residential, office and commercial, the indoor design temperature of summer is generally in the range of 25–28°C. In this paper, the design temperature is 25°C, and the temperature collected during the experiment is employed to obtain the hourly cooling load of the building model, as shown in Figure 17. As the indoor and outdoor temperatures increase, the indoor cooling load also increases, but the model with phase change composites board has a certain hysteresis. This is attributed to the temperature increase rate is slower during the melting process of the phase change material. As the temperature tends to be constant, the cooling load required indoors also tends to be constant. Compared with traditional insulation foam board, the building model with copper foam/paraffin composites board can effectively reduce the energy consumption by up to 21 % and have a great energy saving effect.
In summary, the appearance of the maximum internal temperature of the building model using the copper foam /paraffin composite phase change insulation board was delayed by 1.5 h, the maximum indoor temperature was reduced by 1.2°C, and the indoor energy consumption was reduced by up to 21%. Therefore, the use of copper foam /paraffin composite phase change materials can significantly reduce indoor temperature fluctuations and reduce indoor energy consumption.
Simulation
From the simulation results of July 1st in a summer design day, the indoor temperature of the gypsum board structure, the insulation foam board structure and the phase change material board structure are compared, as shown in Figure 18.
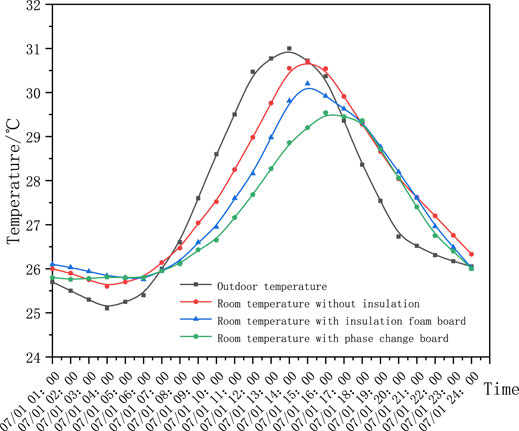
FIGURE 18. Simulation results of the indoor temperature with various materials in a summer design day.
It can be seen from Figure 18 that the lowest outdoor temperature of 25.1°C in the summer design day occurs at 4:30 am in the morning, and then the temperature starts to rise, it approaches a peak temperature of 31°C at 14:00 pm in the afternoon. The indoor temperature of the three materials changes with the outdoor temperature, but there is hysteresis. The indoor temperature without insulation layer reached a peak temperature of 30.7°C at 14:48 pm. The indoor temperature peak with the insulation foam board is 30.2°C, which also appeared at 14:48 pm due to the insulation foam board cannot absorb the heat. The indoor temperature with the phase change material board reached a peak temperature of 29.6°C at 15:20 pm.
By comparing the peaks of the temperature curves in the figure, it can be found that the maximum indoor temperature of the insulation foam board is reduced by 0.6°C compared to the pure gypsum board structure, and the maximum indoor temperature of the phase change material board is reduced by 1.2°C compared to the gypsum board structure. Both materials have a certain suppression effect on the indoor temperature. However, from the time when the peak appears, it can be seen that the phase change material board has an excellent delaying effect on the peak temperature. The indoor temperature peak of the insulation foam board structure is delayed by 0.8 h compared to the outdoor temperature peak, and the indoor temperature peak of the phase change material board is delayed by 1.3 h compared to the outdoor temperature peak.
Figures 19, 20 are the monthly cooling load and monthly heating load of the building models under different enclosure structures, respectively. It can be seen from the figure that during the air conditioning season from May to October, the building model with phase change material panels can significantly reduce the indoor energy consumption by up to 19 %. However, in other months, as the outdoor temperature is not high enough to melt the phase change material, the indoor energy consumption of the building models with the insulation foam board and the phase change material board is not much different.
In the heat transfer experiment, compared with the traditional thermal insulation foam board, the phase change composites board made of copper foam/paraffin delayed the appearance of the highest indoor temperature by 1.5 h and reduced the highest indoor temperature by 1.2°C, and the maximum indoor energy consumption decreased by 21 %.
In the simulation process, the phase change composites board delayed the appearance of the highest indoor temperature by 1.3 h and reduced the highest indoor temperature by 1.1°C, and the maximum indoor energy consumption decreased by 19 %. Therefore, the experimental and simulation results agreed fairly well, which also validated the reliability of the simulation model. Phase change composite materials is able to suppress the indoor temperature fluctuation and reduce the indoor energy consumption.
Conclusion
In this paper, a series of phase change composite materials were fabricated by the melting impregnation method. From the perspective of economy and practicability, 50 ppi copper foam/paraffin PCM composite was selected for constructing the building models. The heat transfer experiment was conducted for the PCM model and the reference model, respectively. In addition, the EnergyPlus software was employed to simulate the indoor temperature and energy consumption for the building models with various materials.
The Following Conclusions can Be Drawn
1) Copper foam/paraffin composite materials can improve the thermal conductivity of composite materials. In the case of the same porosity, the thermal conductivity of the composite material increases with the increase of the pore density. Copper foam can increase the thermal conductivity of composite materials by 3–6 times, depending on the structure of the copper foam. As the pore density increases, the thermal conductivity of the composite material increases more slowly.
2) In the heat transfer experiment, the indoor temperature of the PCM building model was delayed by 1.5 h compared with the reference building model. It also reduced the indoor temperature by up to 1.2°C and the maximum indoor energy consumption by 21 %. Therefore, PCM composites can offer a great energy saving effect.
3) The results of the numerical simulation are basically consistent with the experiment. The architectural model with copper foam/paraffin composite phase change materials on the roof has a peak time of temperature in 15:20 pm, while the peak time of outdoor temperature is 14:00 pm. The maximum indoor temperature was reduced by 1.1 °C and delayed by 1.3 h, and the indoor energy consumption was reduced by up to 19 %.
In summary, copper foam can be utilised as a supporting material to improve the heat transfer performance of composite materials, copper foam/paraffin composite materials can effectively reduce the indoor temperature fluctuations and building energy consumption, as well as improve the thermal comfort of the building. Therefore, copper foam/paraffin composite materials have great application prospect in the field of building energy saving.
In order to make the composite phase change material have more extensive applications in the field of building energy saving, it is necessary to further study the energy saving characteristics of the composite phase change board loaded with different environmental climates or different building envelope structures. The copper foam /paraffin composite phase change board is used as a wall structure to cover the roof. The strength and durability of its structure have yet to be tested. Different types of shaped carrier substrates can be explored.
Data Availability Statement
The original contributions presented in the study are included in the article/supplementary material, further inquiries can be directed to the corresponding authors.
Author Contributions
GZ and YD developed idea of the paper and provided funding for the reaerach. WL and XY interpreted the results. YG and ZL wrote the manuscript. All authors contributed to the discussion of the results and the manuscript editing.
Funding
This work was supported by the National Natural Science Foundation of China (No. 51976126 and No. 52076139), and Youth Eastern Scholar Program of Shanghai Municipal Education Commission.
Conflict of Interest
Author ZL was employed by the company China Aviation Technology Hangzhou Co., Ltd.
The remaining authors declare that the research was conducted in the absence of any commercial or financial relationships that could be construed as a potential conflict of interest.
Publisher’s Note
All claims expressed in this article are solely those of the authors and do not necessarily represent those of their affiliated organizations, or those of the publisher, the editors and the reviewers. Any product that may be evaluated in this article, or claim that may be made by its manufacturer, is not guaranteed or endorsed by the publisher.
References
Amaral, C., Silva, T., Mohseni, F., Amaral, J. S., Amaral, V. S., Marques, P. A. A. P., et al. (2020). Experimental and Numerical Analysis of the thermal Performance of Polyurethane Foams Panels Incorporating Phase Change Material. Energy 213, 119213. doi:10.1016/j.energy.2020.119213
Badenhorst, H. (2019). A Review of the Application of Carbon Materials in Solar thermal Energy Storage. Solar Energy 192, 35–68. doi:10.1016/j.solener.2018.01.062
Crespo, A., Barreneche, C., Ibarra, M., and Platzer, W. (2019). Latent thermal Energy Storage for Solar Process Heat Applications at Medium-High Temperatures - A Review. Solar Energy 192, 3–34. doi:10.1016/j.solener.2018.06.101
de Gracia, A., and Cabeza, L. F. (2015). Phase Change Materials and thermal Energy Storage for Buildings. Energy and Buildings 103, 414–419. doi:10.1016/j.enbuild.2015.06.007
Du, K., Calautit, J., Wang, Z., Wu, Y., and Liu, H. (2018). A Review of the Applications of Phase Change Materials in Cooling, Heating and Power Generation in Different Temperature Ranges. Appl. Energ. 220, 242–273. doi:10.1016/j.apenergy.2018.03.005
Duan, S., Li, H., Zhao, Z., and Wang, L. (2021). Investigation on Heating Performance of an Integrated Phase Change Material Trombe wall Based on State Space Method. J. Energ. Storage 38, 102460. doi:10.1016/j.est.2021.102460
Fu, L., Wang, Q., Ye, R., Fang, X., and Zhang, Z. (2017). A Calcium Chloride Hexahydrate/expanded Perlite Composite with Good Heat Storage and Insulation Properties for Building Energy Conservation. Renew. Energ. 114, 733–743. doi:10.1016/j.renene.2017.07.091
Jouhara, H., Żabnieńska-Góra, A., Khordehgah, N., Ahmad, D., and Lipinski, T. (2020). Latent thermal Energy Storage Technologies and Applications: A Review. Int. J. Thermofluids 5-6, 100039. doi:10.1016/j.ijft.2020.100039
Kenisarin, M. M. (2014). Thermophysical Properties of Some Organic Phase Change Materials for Latent Heat Storage. A Review. Solar Energy 107, 553–575. doi:10.1016/j.solener.2014.05.001
Koohi-Fayegh, S., and Rosen, M. A. (2020). A Review of Energy Storage Types, Applications and Recent Developments. J. Energ. Storage 27, 101047. doi:10.1016/j.est.2019.101047
Li, Y., Yan, H., Wang, Q., Wang, H., and Huang, Y. (2017). Structure and thermal Properties of Decanoic Acid/expanded Graphite Composite Phase Change Materials. J. Therm. Anal. Calorim. 128 (3), 1313–1326. doi:10.1007/s10973-016-6068-4
Liu, Z., Zhang, S., Hu, D., Zhang, Y., Lv, H., Liu, C., et al. (2019). Paraffin/red Mud Phase Change Energy Storage Composite Incorporated gypsum-based and Cement-Based Materials: Microstructures, thermal and Mechanical Properties. J. Hazard. Mater. 364, 608–620. doi:10.1016/j.jhazmat.2018.10.061
Lu, W., Liu, G., Xiong, Z., Wu, Z., and Zhang, G. (2020). An Experimental Investigation of Composite Phase Change Materials of Ternary Nitrate and Expanded Graphite for Medium-Temperature thermal Energy Storage. Solar Energy 195, 573–580. doi:10.1016/j.solener.2019.11.102
Mohandes, B., Acharya, S., Moursi, M. S. E., Al-Sumaiti, A. S., Doukas, H., and Sgouridis, S. (2020). Optimal Design of an Islanded Microgrid with Load Shifting Mechanism between Electrical and Thermal Energy Storage Systems. IEEE Trans. Power Syst. 35 (4), 2642–2657. doi:10.1109/tpwrs.2020.2969575
Navarro, L., de Gracia, A., Niall, D., Castell, A., Browne, M., McCormack, S. J., et al. (2016). Thermal Energy Storage in Building Integrated thermal Systems: A Review. Part 2. Integration as Passive System. Renew. Energ. 85, 1334–1356. doi:10.1016/j.renene.2015.06.064
Qu, Y., Wang, S., Zhou, D., and Tian, Y. (2020). Experimental Study on thermal Conductivity of Paraffin-Based Shape-Stabilized Phase Change Material with Hybrid Carbon Nano-Additives. Renew. Energ. 146, 2637–2645. doi:10.1016/j.renene.2019.08.098
Saafi, K., and Daouas, N. (2019). Energy and Cost Efficiency of Phase Change Materials Integrated in Building Envelopes under Tunisia Mediterranean Climate. Energy 187, 115987. doi:10.1016/j.energy.2019.115987
Uerge-Vorsatz, D., Cabeza, L. F., Serrano, S., Barreneche, C., and Petrichenko, K. (2015). Heating and Cooling Energy Trends and Drivers in Buildings. Renew. Sustain. Energ. Rev. 41, 85–98. doi:10.1016/j.rser.2014.08.039
Violidakis, I., Zeneli, M., Atsonios, K., Strotos, G., Nikolopoulos, N., and Karellas, S. (2020). Dynamic Modelling of an Ultra High Temperature PCM with Combined Heat and Electricity Production for Application at Residential Buildings. Energy and Buildings 222, 16. doi:10.1016/j.enbuild.2020.110067
Wang, H., Lu, W., Wu, Z., and Zhang, G. (2020). Parametric Analysis of Applying PCM Wallboards for Energy Saving in High-Rise Lightweight Buildings in Shanghai. Renew. Energ. 145, 52–64. doi:10.1016/j.renene.2019.05.124
Wei, H., Xie, X., Li, X., and Lin, X. (2016). Preparation and Characterization of Capric-Myristic-Stearic Acid Eutectic Mixture/modified Expanded Vermiculite Composite as a Form-Stable Phase Change Material. Appl. Energ. 178, 616–623. doi:10.1016/j.apenergy.2016.06.109
Wu, S. Y., Tong, X., Nie, C. D., Peng, D. Q., Gong, S. G., and Wang, Z. Q. (2016). The Effects of Various Carbon Nanofillers on the thermal Properties of Paraffin for Energy Storage Applications. J. Therm. Anal. Calorim. 124 (1), 181–188. doi:10.1007/s10973-015-5153-4
Xie, J., Wang, W., Sang, P., and Liu, J. (2018). Experimental and Numerical Study of thermal Performance of the PCM wall with Solar Radiation. Construction Building Mater. 177, 443–456. doi:10.1016/j.conbuildmat.2018.05.123
Xu, Y., Li, M.-J., Zheng, Z.-J., and Xue, X.-D. (2018). Melting Performance Enhancement of Phase Change Material by a Limited Amount of Metal Foam: Configurational Optimization and Economic Assessment. Appl. Energ. 212, 868–880. doi:10.1016/j.apenergy.2017.12.082
Yang, J., Yang, L., Xu, C., and Du, X. (2016). Experimental Study on Enhancement of thermal Energy Storage with Phase-Change Material. Appl. Energ. 169, 164–176. doi:10.1016/j.apenergy.2016.02.028
Yang, X., Wei, P., Cui, X., Jin, L., and He, Y.-L. (2019). Thermal Response of Annuli Filled with Metal Foam for thermal Energy Storage: An Experimental Study. Appl. Energ. 250, 1457–1467. doi:10.1016/j.apenergy.2019.05.096
Zeng, J. L., Liu, Y. Y., Cao, Z. X., Zhang, J., Zhang, Z. H., Sun, L. X., et al. (2008). Thermal Conductivity Enhancement of MWNTs on the PANI/tetradecanol Form-Stable PCM. J. Therm. Anal. Calorim. 91 (2), 443–446. doi:10.1007/s10973-007-8545-2
Zhang, G., Cui, G., Dou, B., Wang, Z., and Goula, M. A. (2018). An Experimental Investigation of Forced Convection Heat Transfer with Novel Microencapsulated Phase Change Material Slurries in a Circular Tube under Constant Heat Flux. Energ. Convers. Manage. 171, 699–709. doi:10.1016/j.enconman.2018.06.029
Zhao, G., Xu, X., Qiu, L., Zheng, X., and Tang, D. (2013). Study on the Heat Conduction of Phase-Change Material Microcapsules. J. Therm. Sci. 22 (3), 257–260. doi:10.1007/s11630-013-0621-2
Zhou, D., Zhao, C. Y., and Tian, Y. (2012). Review on thermal Energy Storage with Phase Change Materials (PCMs) in Building Applications. Appl. Energ. 92, 593–605. doi:10.1016/j.apenergy.2011.08.025
Keywords: thermal energy storage, phase change materials (PCMs), copper foam, indoor thermal comfort, energy saving
Citation: Zhang G, Guo Y, Liu Z, Lu W, Yan X and Du Y (2021) Experimental and Numerical Investigation of Composite Phase Change Materials for Building Energy Saving. Front. Energy Res. 9:721487. doi: 10.3389/fenrg.2021.721487
Received: 07 June 2021; Accepted: 16 August 2021;
Published: 31 August 2021.
Edited by:
Mostafa. S. Shadloo, Institut national des sciences appliquees de Rouen, FranceReviewed by:
Liang Li, University of Hertfordshire, United KingdomLong Zhang, Beijing Institute of Technology, China
Copyright © 2021 Zhang, Guo, Liu, Lu, Yan and Du. This is an open-access article distributed under the terms of the Creative Commons Attribution License (CC BY). The use, distribution or reproduction in other forums is permitted, provided the original author(s) and the copyright owner(s) are credited and that the original publication in this journal is cited, in accordance with accepted academic practice. No use, distribution or reproduction is permitted which does not comply with these terms.
*Correspondence: Guanhua Zhang, Z3Vhbmh1YXpoYW5nQHVzc3QuZWR1LmNu; Yanping Du, eWFucGluZy5kdUBzanR1LmVkdS5jbg==