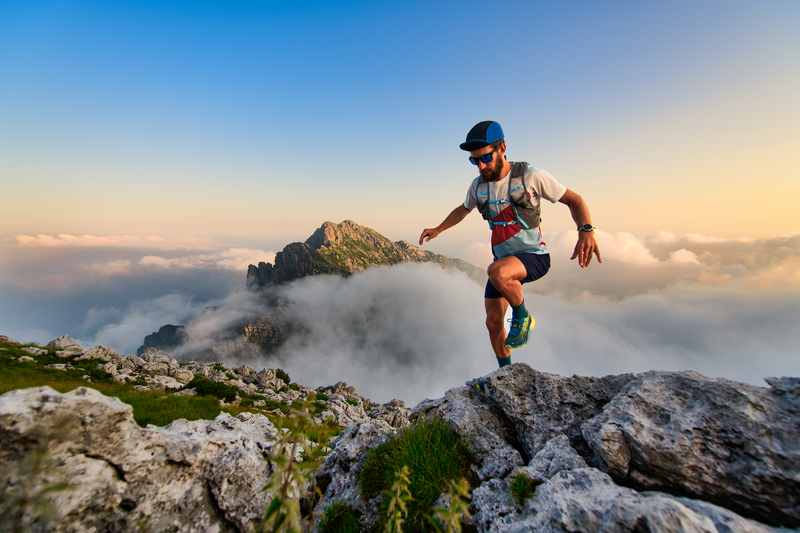
95% of researchers rate our articles as excellent or good
Learn more about the work of our research integrity team to safeguard the quality of each article we publish.
Find out more
PERSPECTIVE article
Front. Energy Res. , 05 August 2021
Sec. Sustainable Energy Systems
Volume 9 - 2021 | https://doi.org/10.3389/fenrg.2021.707867
This article is part of the Research Topic Perspectives of Chemicals Synthesis as a Green Alternative to Fossil Fuels View all 13 articles
The Paris Agreement set policy scenarios to address mitigating against the climate emergency, by reducing greenhouse gas emissions to limit the temperature increase to 1.5°C. There has been a drive toward electrifying transport, with battery electric vehicles (BEVs) at the forefront. Reliance on single-technology policy development can lead to consequential impacts, often not considered, or dismissed. Energy cannot be created or destroyed but can be transformed. While BEVs may represent zero tailpipe emissions, the battery energy must be sourced elsewhere. An ideal policy scenario will come from “renewable” sources; however, current global energy mixes require the electricity to come from carbon-burning point source emitters. Therefore, the emissions are deferred to low socioeconomic regions. The move to ban new internal combustion engine (ICE) vehicle sales has been accelerated. High BEV costs will preclude low-income groups from making purchases. Such groups typically rely on used cars for mobility. Without considered consequential policy analysis, transport underclasses may result, where private transport is only accessible by the wealthy. Synthetic fuels derived from CO2 represent a social bridge in the energy transition, also helping to accelerate toward net zero. The Covid-19 lockdown provided a unique opportunity to experience an environment with reduced transport-related emissions. Global studies allowed the consequential effects of pollution reduction to be studied. These are surprising and offer the opportunity for policies, driven by science, to be developed. Here, we consider the consequential effects of clean air policies, and how these can be used to propose dynamic responses to policy recommendations.
The drive toward “net zero” required to meet the necessary challenges of the Paris Climate Accord (UNFCCC, 2015), proposed at the 21st United Nations Conference of Parties (COP21) and ratified at COP22, together with subsequent amendments (IPCC et al., 2018), requires significant technological and behavioral changes if the global temperature rise is to be limited to 1.5°C or lower. The accord requires significant reduction in anthropogenic greenhouse gas (GHG) emissions. Most efforts have been and are being concentrated on the energy sector; however, action is needed in the transport sector (CCC, 2019). Urban and national transport has been considered as the easiest to de-fossilize through a drive toward battery electric vehicles (BEVs). This is true for the reduction of tailpipe emissions (tank or socket to wheel) but becomes less so when emissions are considered over the complete life cycle of the vehicle emissions (well, or more accurately renewable energy source, to wheel) (Moro and Lonza, 2018; Brand et al., 2020; Carbon Brief, 2020; Dixon et al., 2020). Furthermore, we need to consider the consequential effects of focusing on a single aspect of the transport transition. Net zero technologies will only be successful if they are accompanied by net zero behaviors. What remains clear is the commitment to achieving net zero by 2050. Synthetic transport fuels may aid its acceleration until an EV transition is accomplished and legacy liquid fuel vehicles phased out. There have been a number of studies that consider different approaches to the decarbonization of future transport systems (Royal Society, 2019; Senecal and Leach, 2019; Senecal and Leach, 2021), but the Covid lockdown of 2020 has given a unique insight into what may happen if road transport emissions are significantly reduced.
Net zero is defined as the removal of unavoidable emissions from the atmosphere using technological or natural interventions (sequestration or offsetting). Also key to this is the need to avoid emissions or at least reduce them. A full understanding of the impact of fossil fuel replacement on emissions and the effect of conventional fuel prohibition on social justice is required. During the COVID-19 pandemic, an unintended consequence of lockdowns, and so reductions in road traffic, has been an insight into what a low-emission future may look like (Brimblecombe and Lai 2020; Wang et al., 2020). In this study, we consider what efforts can be made to accelerate the transition to net zero by using synthetic chemical fuels to power legacy internal combustion engines (ICEs) while BEVs become fully established.
It is clear that we must take a systemic approach rather than considering isolated policies, as unintended negative consequences must be avoided. In this respect, we consider the negative potential for increasing social injustice and the creation of a transport underclass if we do not consider the long-term consequences of ill-thought-out policy actions. By applying a theory of change approach, we propose a possible solution to a multifaceted approach to accelerate toward net zero.
Mobility is at the center of international economies. To ensure stable and prosperous futures, we need to have the capability to move people, food, and commodity goods locally, nationally, and internationally. The first law of thermodynamics states that in a closed system, energy cannot be created or destroyed and that it can only be converted from one form to another. We have used Earth’s fossil resources to create the energy we need, including power generation and mobility. The reason we used these sources is that they are dense in energy and are easily converted through combustion. However, this produces unwanted side products such as carbon dioxide (CO2), nitrogen and sulfur oxide gases (NOx and SOx), and particulate matter (PM), such as soot and nanoparticles. These side products are unfortunately harmful to the environment and health. Despite the development of cleaner combustion engines, emissions persist. Policy has driven technology development, including legislation to replace lead in petrol during the 1980s, to the United Kingdom policy incentives for diesel vehicles in 2001. While diesel fuel produces less of the greenhouse gas (GHG) carbon dioxide than petrol, there are increased NOx and PM emissions which can contribute to respiratory illnesses. The panacea of transport is the so-called zero-emission vehicle that is technically impossible to produce because of the first law of thermodynamics and the subject of many misconceptions.
Achieving a net zero emissions policy by 2050 currently seems ambitious (EU, 2019). However, predictions thirty years into the future are difficult, and while the Committee on Climate Change (CCC, 2019) set legally binding five-year carbon budgets, they concede that these contain inherent uncertainties. It is reasonable to suggest that battery technology and energy harvesting from solar and wind sources will undergo significant improvements (IEA, 2020a) and that car design and manufacture will result in lighter, more efficient performance and energy conversion. However, we will still be using legacy ICEs for the foreseeable future.
The fear is that a radical move toward abolishing ICEs will result in a transport social underclass where lower socioeconomic groups become transport impoverished. Taking the United Kingdom as an example, we may be able to find some solace in the rationale behind a transition. The United Kingdom government has said it will ban the sale of new petrol (gasoline) and diesel vehicles by 2030, bringing forward its initial ambition by ten years. It will also ban the sale of new hybrid vehicles by 2035. Many from low-income communities rely on cheap, used vehicles to reach their place of employment. The new legislation will prohibit new ICE vehicle sales, not used vehicles. Petrol vehicles typically have a life of 10 years or 100,000 miles and diesels 20 years or 200,000 miles, with an average vehicle life of 13.9 years (SMMT, 2020). This would give an upper limit of twenty years to the vehicle lifetime. By 2050 all ICE vehicles will have naturally reached the end of their life, and only BEVs will exist on the used car market, which looks like a reasonable transition strategy. However, it relies on rapid improvement in battery technologies and social interventions, as the current battery lifetime will limit their long-term viability in a low-cost used BEV market. Interventions will need to address the reconditioning of batteries for resale. Robust policies need to be in place at the local and national levels to develop mass transit systems that allow convenient, clean, and cheap travel with efficient interconnectivity. Logan et al. (2020) have carried out a recent analysis of such a transition in the United Kingdom that points the way to possible solutions.
Synthetic fuels have a role to play in accelerating defossilization of transport in the period between 2030 and 2050. Furthermore, the current policy does not cover commercial heavy goods vehicles, so synthetic liquid fuels to replace diesel will play an increasingly important role (Styring et al., 2021). We have used a theory of change approach (Figure 1) to evaluate the transition to fully electric mobility, identifying gaps and hot spots in the process and policy development. Direct air capture (DAC) of CO2 emissions from the atmosphere is one method to achieve net zero; however, it is currently expensive and not available at scale (McQueen et al., 2021).
We see that in focusing on transport as a pathway to net zero, BEVs fuelled by 100% renewable energy sources are seen as the solution. While the tailpipe CO2 emissions will be zero in this scenario, there will still be production and end-of-life decommissioning emission embodied in the system.
The internal combustion engine is not the problem; the fuel is. The human body requires fuel to survive. If we use the wrong fuel, the body responds accordingly, a feature that is clearly shown in the link between junk food and obesity. If the ICE has been developed over decades to become more and more efficient, then maybe the engine is not the problem but the fuels we use are. The Royal Society (2019) published a policy briefing that considered alternative fuels for transport. Hydrocarbons such as petrol, diesel, and kerosene can be made synthetically using waste carbon–containing feedstock, which reduces fossil-carbon consumption. CO2 is still produced, but this is now second-life carbon (Styring et al. 2021). However, synthetic hydrocarbons still produce particulate emissions and NOx due to their combustion chemistry. Dimethyl ether (DME) is a direct replacement or drop-in fuel for diesel engines, needing a small modification to the fuel delivery system and the injection timing (Styring and Dowson, 2021). A typical diesel engine such as that found in a Ford Mondeo will emit around 250 g CO2 per km traveled, while Ford has reported that if DME is used instead, the emissions drop to 3 g per km (Lee et al., 2016; Willems, 2018). As the emissions associated with the manufacture and decommissioning of the vehicle are essentially the same as those of the conventional diesel vehicle, the life-cycle emission profile is considerably better than even a BEV’s. Ellingsen et al. (2016) stated the true emissions of a BEV over the cradle-to-grave analysis, which means that to meet net zero criteria, a medium-size car needs to drive 70,000 km before tailpipe emission reduction is met. This is the crossover point or breakthrough, where the embedded and use emissions from a BEV first equal and then fall below the emissions for a conventional and equivalent ICE vehicle. The study demonstrates that only mini (Class A) cars show favorable life-cycle emission reduction relative to fossil-fueled cars and that a small diesel car shows better full-life emission characteristics than larger BEVs. If we relate DME emissions to the Ellingsen life cycle evaluation, then the crossover point is significantly reduced, so less mileage is required before the DME vehicle shows improvement over the BEV equivalent.
The 2020 Covid-19 lockdown and consequential reduction in traffic emissions provided an insight into future transport scenarios. While expected reductions in atmospheric CO2 and NO2 levels were observed, there were unexpected increases in PM levels. This was observed by groups in China (Brimblecombe and Lai 2020;Wang et al., 2020), where the lockdown was particularly strict, and by us in Sheffield, United Kingdom (Alam et al., 2021), using a Flow 2 sensor (PlumeLabs 2021) mounted on a bicycle, as shown in Figure 2.
FIGURE 2. Emission data during United Kingdom Lockdown 3. Wind was blowing at 9 mph from the southwest. Light traffic and no obvious wood fires, except wood-burning stoves in Dore (Flow 2 data). The circular pictograms refer to the species analysis as given in the chemical labels at the side of each figure.
PM2.5s are particles with diameters less than 2.5 µm and are not only breathed in by humans but respired; taken into the lung capillaries and distributed around the body. While such particles are themselves hazardous to health, they are also able to carry nanoparticles (PM0.1 and below), which include viruses, into the body, thereby increasing their risk to health. The GPS-emission plots shown in Figure 2 were recorded during the first United Kingdom lockdown. Further details are reported in the Supplementary Material. The combined emission data (A) show three hot spots for hazardous emissions, as depicted by the color of the data. Green is safe, while purple is hazardous to health, with intermediate shades depicting a range of hazards (see the Supplementary Material for color coding). The nitrogen oxide data (E) and volatile organic compound (VOC) data (F) show very low levels of emissions, which correlates well with there being very little road traffic. Three hot spots relate to PM emissions, particularly PM10 and PM1. As the NO2 levels are low, these cannot be associated with road traffic, and two of the sites (Ecclesall and Millhouses) are suburban, while the third (Dore) is semirural, suggesting that the emission is not agricultural. Some of the emissions in Dore are due to wood-burning stoves, but the link between each of the sites was the presence of local bakeries which were allowed to remain open during the lockdown. So, in this period, the emissions were linked to cooking and heating activities rather than transport-related emissions. An explanation for similar observations of high PM levels at low NO2 emission levels was also being developed in China, where lockdown restrictions were even more severe (Brimblecombe and Lai, 2020; Wang et al., 2020). Under normal conditions, solar radiation reacts with oxygen to produce ozone, which in turn reacts with NOx to yield other products. In the absence of any significant amounts of NOx from vehicle exhausts, the ozone reacts with volatile organic compounds such as methane and cooking emissions in the atmosphere to produce particulate matter (PM) which increases the atmospheric concentration. These studies are continuing to aid an understanding of the atmospheric chemistry, which changes as the emission portfolio is altered through interventions. Particulate emissions are also associated with non-combustion vehicle emissions such as brake and tire abrasion in BEVs; however, there were no significant correlations between the observed PM emissions and vehicular activity during the lockdown.
Many political manifestos aspire to achieve net zero using a suite of technological and policy interventions. However, these generally make assumptions that technologies already exist or are close to commercialization, while the maturity of these may be overestimated. A common proposal is that CCS technologies will be available at scale by 2050. While such facilities have been operating in Norway for over fifty years, the global capacity, the majority of which is enhanced fossil oil recovery, is currently around 40 million tonnes per annum. By contrast, total global emissions are around 1,000 times higher (Global CCS Institute, 2021). The European Union policy document “A Cleaner Planet for All” shows that widespread CCS will not be available until around 2050 and still not at a scale to make a significant impact on global emissions (EU, 2019). A second assumption is that EVs will decarbonize the transport sector. There will always be embedded emissions from vehicle production and disposal at the end of life and electricity generation from fossil resources until a 100% renewable electricity grid is achieved, and so CCS will be necessary to remove these emissions. A third policy involves carbon offsetting through the planting of trees and indeed forests, which is the so-called afforestation. We have already considered the first two assumptions, but what about the third?
Policy makers often propose planting trees as a solution to offset CO2 emissions. Whilst trees can mitigate some CO2 emissions, there is a lack of discussion on the extent of this capability. Various factors affect a tree’s ability to absorb CO2, such as tree species, age, and climate. To estimate the amount of CO2 sequestrated per year per tree, numeric tables which give values based on the tree’s age and species (US Department of Energy, 1988) can be used. A single tree can sequestrate 1.66 kg CO2/year in its first year of growth. Whilst the sequestration rate does increase past the first year, so does tree mortality. This decreases the net CO2 stored in two ways; when a tree is dead, it can no longer absorb CO2, and second, on decomposition, it emits CO2 and, more problematically, methane, which has 24 times the GHG potential of CO2.
To explain the scale on which trees would need to be planted to offset emissions, a scenario is proposed where an average diesel car with a CO2 emission of 122.1 gCO2/km (DFT, 2015, DVLA) travels 10,000 miles annually, emitting approximately 1,965 kg CO2. It would take 1,180 trees to offset these emissions each year. Currently, there are over 38 million United Kingdom registered cars, so over 45 billion trees would need to be planted annually to offset United Kingdom private car emissions alone. Furthermore, with growing vehicle ownership, this number could rise even further (DFT, 2020). To plant that many trees, a land area of 22.5 million Ha would be needed; the United Kingdom land area is approximately 24.3 million Ha (Office for National Statistics, 2021).
There also need to be policy interventions that incentivize behavioral change. Technology alone cannot solve the problem without public acceptance. If a social underclass is to be avoided, there needs to be a balance between mandatory legislation and responsible fuel use. The electrification policy needs to include provisions for the transition of legacy vehicles including low carbon fuel subsidies. Together with policy interventions and financial easing of the transition, there also need to be fiscal measures in place to incentivize defossilization while at the same time disincentivizing fossil carbon use.
Many technologies in their early development cycles require significant investment above that of the counterfactual to encourage technological, consumer, and behavioral change. This is certainly the case in the transport sector, where fossil fuels are relatively inexpensive but also attract state subsidies to maintain a security of supply. It is reasonable to propose that as the transition away from fossil-based resources takes effect, there should also be a transition away from conventional supply chains to the new, greener technologies. Furthermore, the VAT on synthetic, low-carbon fuels could be subject to zero sales tax, at least in the early years of transition, while at the same time applying VAT at the upper rate to fossil carbon fuels (PGES, 2021). There is also a strong need for a robust and realistic carbon tax or emission tax to be applied to the full range of emitters, from point source energy and industrial sources to transport and domestic emissions. The current carbon price does not deter emitters, and the International Energy Agency (IEA) have suggested that a higher value is needed (IEA, 2020b).
We need to reduce emissions if we are to avoid irreversible climate change. This can only be achieved by using a multifaceted approach where policy, technology development, financial incentives, and behavioral change work together. It is reasonable to conclude that the 2050 net zero policy is achievable in principle, but we should be aware that paradigm technology shifts are required.
• Net zero as an aspiration can only be achieved by radical change in attitudes, behaviors, and technologies. This includes taking a more active approach to transport such as walking and cycling and the introduction of more interconnected mass transit systems.
• BEVs are not zero-emission vehicles over the complete life cycle. They can produce zero tailpipe emissions, but the other emissions are transferred to other locations, often in low socioeconomic areas of states. While not covered in detail, we should also be aware of embodied emission, including increased production and decommissioning costs, and increased brake and tire wear.
• BEVs will not provide the answer to road transport emissions if they are treated as an isolated technology. Full systemic life cycle assessment is needed from the cradle to the grave, not just the mobility emissions.
• When considering a whole system, the interdependency of different policy interventions should be considered. Unintended consequences should be included in a risk analysis of mitigation strategies, including changes in atmospheric chemistry that are promoted.
• It is unlikely that afforestation as a carbon offsetting policy will be effective given the area of land required and the timescales involved. A transition of subsidies, away from fossil fuels and toward low-carbon technologies, is required, and this needs to be accompanied by a transition in the skill sets of workforces to ensure a stable economy. Synthetic fuels provide a route to accelerate a transition.
• Fiscal interventions will be essential (reduction in the VAT for low-carbon fuels and a sensible carbon tax), coupled with financial support, if a socially just low-carbon transport revolution is to be realized.
• Care must be taken so that a transport underclass is not created. Small vehicle defossilization will most likely be achieved using BEVs, but synthetic low-emission fuels can help bridge the gap between ICEs and new electrified mobility in the transition period between 2030 and 2050.
• While it is acknowledged that BEVs will provide the long-term solution for private ground transportation, long-haul road transport, aviation, and maritime vessels will prove more difficult to defossilize, and so alternative ICE fuels based on recycled waste carbon feedstock may prove to be lower carbon options to accelerate the transition to net zero.
The data analyzed in this study is subject to the following licenses/restrictions: emissions data used in some of the article are available in a Faraday Discussion. Details will be made available on request. Requests to access these datasets should be directed to cC5zdHlyaW5nQHNoZWZmaWVsZC5hYy51aw==.
PS led the analysis of the policy and the theory of change. ED conducted the offsetting analysis, and EP assisted in the air quality analysis.
The authors declare that the research was conducted in the absence of any commercial or financial relationships that could be construed as a potential conflict of interest.
All claims expressed in this article are solely those of the authors and do not necessarily represent those of their affiliated organizations, or those of the publisher, the editors and the reviewers. Any product that may be evaluated in this article, or claim that may be made by its manufacturer, is not guaranteed or endorsed by the publisher.
We thank the Engineering and Physical Sciences Research Council for funding the research under the UKRI Interdisciplinary Centre for Circular Chemical Economy programme (EP/V011863/1).
The Supplementary Material for this article can be found online at: https://www.frontiersin.org/articles/10.3389/fenrg.2021.707867/full#supplementary-material
Alam, M. S., Bloss, W., Brean, J., Brimblecombe, P., Chan, C., Chen, Y., et al. (2021). General Discussion: Aerosol Formation and Growth; VOC Sources and Secondary Organic Aerosols. Faraday Discuss. 226, 479–501. doi:10.1039/D1FD90011K
Brand, C., Anable, J., Ketsopoulou, I., and Watson, J. (2020). Road to Zero or Road to Nowhere? Disrupting Transport and Energy in a Zero Carbon World. Energy Policy 139, 111334. doi:10.1016/j.enpol.2020.111334
Brimblecombe, P., and Lai, Y. (2020). Diurnal and Weekly Patterns of Primary Pollutants in Beijing under COVID-19 Restrictions. Faraday Discuss. 226, 138–148. Advanced article. doi:10.1039/d0fd00082e
Carbon Brief (2020). Factcheck: How Electric Vehicles Help to Tackle Climate Change. London: Carbon Brief. Available at: www.carbonbrief.org/factcheck-how-electric-vehicles-help-to-tackle-climate-change (Accessed May 8, 2021).
CCC (2019). Climate Change Committee. London: Committee on Climate Change Available at: www.theccc.org.uk/wp-content/uploads/2019/05/Net-Zero-The-UKs- contribution-to-stopping-global-warming.pdf (Accessed March 8, 2021).
DFT (2020). Department for Transport. (2020). Vehicle Licensing Statistics: July to September 2020 Data Tables London: Department for Transport. [Data set]. Available from: www.gov.uk/government/statistics/vehicle- licensing-statistics-july-to-september-2020 (Accessed February 17, 2021).
DFT (2015). Department for Transport. DVLA (2015). Average CO2 Emissions of Newly Registered Cars . [Data set] London: Department for Transport. Available from: https://www.gov.uk/government/publications/new-car-carbon-dioxide-emissions#history (Accessed February 17, 2021).
Dixon, J., Bukhsh, W., Edmunds, C., and Bell, K. (2020). Scheduling Electric Vehicle Charging to Minimise Carbon Emissions and Wind Curtailment. Renew. Energ. 161, 1072e1091. doi:10.1016/j.renene.2020.07.017
Ellingsen, L. A. W., Singh, B., and Strømman, A. H. (2016). “The Size and Range Effect: Lifecycle Greenhouse Gas Emissions of Electric Vehicles,” Environ. Res. Lett. 11, 054010. doi.org/doi:10.1088/1748-9326/11/5/054010
EU (2019). Communication from the Commission to the European Parliament, the European Council, the Council, the European Economic and Social Committee Belgium: European Union, Brussels. Available from: https://eur-lex.europa.eu/legal- content/EN/TXT/?uri=CELEX:52018DC0773 (Accessed March 8, 2021).
Global CCS Institute (2021). Global Carbon Capture and Storage Institute Australia: Global CCS Institute, Melbourne. Available from: https://www.globalccsinstitute.com/resources/global-status-report/ (Accessed March 8, 2021).
IEA (2020a). International Energy Agency Paris: IEA. Available at: www.iea.org/reports/world-energy-outlook-2020/achieving-net-zero-emissions-by-2050 (Accessed March 8, 2021).
IEA (2020b). Price Level of Selected Carbon Pricing Instruments Covering the Power Sector. as of April 2020. Paris: IEA. Available from: www.iea.org/data-and-statistics/charts/price-level-of-selected-carbon-pricing-instruments-covering-the-power-sector-as-of-april-2020 (Accessed March 8, 2021).
Lee, U., Han, J., Wang, M., Ward, J., Hicks, E., Goodwin, D., et al. (2016). Well-to-Wheels Emissions of Greenhouse Gases and Air Pollutants of Dimethyl Ether from Natural Gas and Renewable Feedstocks in Comparison with Petroleum Gasoline and Diesel in the United States and Europe. SAE Int. J. Fuels Lubr. 9 (3), 546–557. doi:10.4271/2016-01-2209
Logan, K. G., Nelson, J. D., and Hastings, A. (2020). Electric and Hydrogen Buses: Shifting from Conventionally Fuelled Cars in the UK. Transportation Res. D: Transport Environ. 85, 102350. doi:10.1016/j.trd.2020.102350
McQueen, N., Gomes, K. V., McCormick, C., Blumanthal, K., Pisciotta, M., and Wilcox, J. (2021). A Review of Direct Air Capture (DAC): Scaling up Commercial Technologies and Innovating for the Future. Prog. Energ. 3, 032001. doi:10.1088/2516-1083/abf1ce
Moro, A., and Lonza, L. (2018). Electricity Carbon Intensity in European Member States: Impacts on GHG Emissions of Electric Vehicles. Transportation Res. Part D: Transport Environ. 64, 5–14. doi:10.1016/j.trd.2017.07.012
Office for National Statistics (2021). Standard Area Measurements (2020) for Administrative Areas in the United Kingdom. [Data Set] Office for National Statistics London: Office for National Statistics. Available at: https://geoportal.statistics.gov.uk/datasets/e919e5a1647f426595650429937b7ad5 Available from: geoportal.statistics.gov.uk/datasets/standard-area-measurements-2019-for-administrative-areas-in-the-united-kingdom (Accessed February 15, 2021).
PGES (2021). All Party Parliamentary Group for Energy Studies, “What Are the Energy Policies that Will Drive an Independent UK to Net Zero while Fuelling the Economy?”. Energy Policy Document London: The All-Party Parliamentary Group for Energy Studies (PGES). Available from: https://pges.org.uk/wp- content/uploads/2021/03/PGES-Inquiry-Report-FINAL.pdf (Accessed March 9, 2021).
PlumeLabs (2021). PlumeLabs Paris: Plume Labs. https://plumelabs.com/en/ (Accessed March 8, 2021).
Royal Society, (2019). Synthetic Transport Fuels, Policy Briefing. London, England: The Royal Society.
Senecal, K., and Leach, F. (2021). Racing toward Zero: The Untold Story of Driving Green. USA: SAE International. ISBN 978-1-4686-0146-6.
Senecal, P. K., and Leach, F. (2019). Diversity in Transportation: Why a Mix of Propulsion Technologies Is the Way Forward for the Future Fleet. Results Eng. 4, 100060. doi:10.1016/j.rineng.2019.100060
SMMT (2020). Average Vehicle Age London: The Society of Motor Manufacturers and Traders. Avaialable at: www.smmt.co.uk/industry-topics/sustainability/average-vehicle-age/ (Accessed May 8, 2021).
Styring, P., and Dowson, G. R. M. (2021). Oxygenated Transport Fuels from Carbon Dioxide : Driving towards Net Zero. Johnson Matthey Technology Rev. 65, 170–179. doi:10.1595/205651321x16063027322661
Styring, P., Dowson, G. R. M., and Tozer, I. O. (2021). Synthetic Fuels Based on Dimethyl Ether as a Future Non-fossil Fuel for Road Transport from Sustainable Feedstocks. Front. Energ. Res. 9, 663331. doi:10.3389/fenrg.2021.663331
UNFCCC (2015). United Nations Framework Convention on Climate Change Bonn: United Nations Framework Convention on Climate Change. Available at: unfccc.int/sites/default/files/english_paris_agreement.pdf. (Accessed 8, March2021).
US Department of Energy (1988). Method for Calculating Sequestration by Trees in Urban and Suburban Settings. Washington, DC: United States Department of Energy, Energy Information Administration. Available from: www3.epa.gov/climatechange/Downloads/method-calculating-carbon-sequestration-trees-urban-and-suburban-settings.pdf (Accessed March 8, 2021).
IPCC (2018). “Summary for Policymakers,” in Global Warming of 1.5°C. An IPCC Special Report on the Impacts of Global Warming of 1.5°C above Pre-industrial Levels and Related Global Greenhouse Gas Emission Pathways, in the Context of Strengthening the Global Response to the Threat of Climate Change, Sustainable Development, and Efforts to Eradicate Poverty. Editors V. Masson-Delmotte, P. Zhai, H. O. Pörtner, D. Roberts, J. Skea, P. R. Shuklaet al. (Geneva, Switzerland: World Meteorological Organization), 32. Available at: www.ipcc.ch/sr15/faq/faq-chapter-1/ (Accessed March 8, 2021).(eds.)].
Wang, Y., Wen, Y., Wang, Y., Zhang, S., Zhang, K. M., Zheng, H., et al. (2020). Four-Month Changes in Air Quality during and after the COVID-19 Lockdown in Six Megacities in China. Environ. Sci. Technol. Lett. 7, 802–808. doi:10.1021/acs.estlett.0c00605
Keywords: synthetic fuel, policy development, electric vehicle, ICE, net zero carbon, social impact
Citation: Styring P, Duckworth EL and Platt EG (2021) Synthetic Fuels in a Transport Transition: Fuels to Prevent a Transport Underclass. Front. Energy Res. 9:707867. doi: 10.3389/fenrg.2021.707867
Received: 11 May 2021; Accepted: 09 July 2021;
Published: 05 August 2021.
Edited by:
Luis Puigjaner, Universitat Politecnica de Catalunya, SpainReviewed by:
Veera Gnaneswar Gude, Mississippi State University, United StatesCopyright © 2021 Styring, Duckworth and Platt. This is an open-access article distributed under the terms of the Creative Commons Attribution License (CC BY). The use, distribution or reproduction in other forums is permitted, provided the original author(s) and the copyright owner(s) are credited and that the original publication in this journal is cited, in accordance with accepted academic practice. No use, distribution or reproduction is permitted which does not comply with these terms.
*Correspondence: Peter Styring, cC5zdHlyaW5nQHNoZWZmaWVsZC5hYy51aw==
Disclaimer: All claims expressed in this article are solely those of the authors and do not necessarily represent those of their affiliated organizations, or those of the publisher, the editors and the reviewers. Any product that may be evaluated in this article or claim that may be made by its manufacturer is not guaranteed or endorsed by the publisher.
Research integrity at Frontiers
Learn more about the work of our research integrity team to safeguard the quality of each article we publish.