- 1Department of Chemistry, Umeå University, Umeå, Sweden
- 2RISE Processum AB, Örnsköldsvik, Sweden
- 3SEKAB E-Technology AB, Örnsköldsvik, Sweden
Inhibitors formed during pretreatment impair lignocellulose bioconversion by making enzymatic saccharification and microbial fermentation less efficient, but conditioning of slurries and hydrolysates can improve fermentability and sometimes also enzymatic digestibility. Conditioning of pretreated softwood using four industrial reducing agents (sodium sulfite, sodium dithionite, sodium borohydride, and hydrogen) was compared with standard methods, such as overliming and treatment with activated charcoal. A dosage of approx. 1 mM sulfur oxyanion (sulfite or dithionite) per percent water-insoluble solids (WIS) in the slurry was found to result in good fermentability. Treatment of 10–20% WIS slurries with 15 mM sulfur oxyanion under mild reaction conditions (23°C, pH 5.5) resulted in sulfonation of the solid phase and saccharification improvements of 18–24% for dithionite and 13–16% for sulfite. Among the different conditioning methods studied, treatment of slurries with sodium sulfite was superior with respect to cost-efficient improvement of fermentability. Treatments of slurry or pretreatment liquid with 15 mM sulfite or dithionite resulted in 58–76% reduction of the content of formaldehyde. The comparison indicates that conditioning of pretreated biomass using sulfur oxyanions warrants further attention.
Introduction
Lignocellulosic biomass is an abundant renewable resource for production of advanced biofuels, green chemicals, and bio-based materials (Ragauskas et al., 2006; Galbe and Wallberg, 2019). The main organic constituents are cellulose, hemicelluloses, and lignin. Biochemical conversion of lignocellulose using hydrothermal pretreatment, enzymatic saccharification, microbial fermentation of sugars, and valorization of hydrolysis lignin is one of the most common approaches studied. As sugars are prominent intermediates, this is sometimes referred to as a sugar-platform process.
Hydrothermal pretreatment under acidic conditions primarily targets hemicelluloses and the main goal of the pretreatment is to disrupt the physical structure, decrease the particle size, and increase the susceptibility of the cellulose to enzymatic saccharification. Hydrothermal pretreatment is sometimes performed as steam explosion and sometimes acid catalysts are added to promote hydrolysis of hemicellulose (Gandla et al., 2018). Regardless of whether acid is added, the pretreatment will be acidic, as carboxylic acids are formed when hemicelluloses are degraded. Depending on the recalcitrance of the feedstock, the conditions that are utilized for pretreatment may be more or less severe. Typical conditions include temperatures of around 200°C and residence times of a few minutes (Gandla et al., 2018). Softwood, a common type of lignocellulosic biomass in boreal and temperate forests, contains a relatively high fraction of lignin and is comparatively recalcitrant (Galbe and Wallberg, 2019).
Enzymatic and microbial processes can be combined or separated. Common approaches include separate hydrolysis and fermentation (SHF) and simultaneous saccharification and fermentation (SSF) (Öhgren et al., 2007). More recently, hybrid hydrolysis and fermentation (HHF) has emerged as a common approach (Zhong et al., 2019). In HHF, enzymes are first added at temperatures of around 50°C, and, after a while, the temperature is lowered to 30°C, the microorganism is added, and enzymes and microorganism then act in concert. In SHF, the residual solids can be removed before the fermentation step, but in SSF and HHF there will be both a liquid and a solid phase during the fermentation. The efficiency of enzymatic saccharification is strongly affected by the consistency, which is sometimes determined as the content of WIS, water-insoluble solids. When WIS content or dry-matter content increases, enzymatic saccharification becomes increasingly challenging (Hoyer et al., 2009; Kristensen et al., 2009; Koppram et al., 2014).
Under severe acidic pretreatment conditions, hemicelluloses are degraded to monosaccharide sugars, but there will also be formation of by-products (Rasmussen et al., 2014; Jönsson and Martín, 2016). If concentrations are sufficiently high, these by-products may inhibit enzymes used for saccharification and microbes used for the fermentation process. Monosaccharides and phenols are important inhibitors of cellulolytic enzymes (Ximenes et al., 2010; Zhai et al., 2016). Aliphatic carboxylic acids (such as acetic acid, formic acid, and levulinic acid), aliphatic aldehydes (such as formaldehyde), benzoquinones (such as p-benzoquinone), furans [such as furfural and 5-hydroxymethylfurfural (HMF)], and phenylic substances (i.e., phenolic and non-phenolic aromatics) are important inhibitors of fermenting microorganisms (Jönsson and Martín, 2016).
There are many approaches to reduce problems with inhibition (Jönsson et al., 2013). One of the most commonly used is conditioning, which includes measures such as adjustment of pH, addition of essential nutrients for microorganisms, dilution, and various treatments removing or inactivating inhibitors. Conditioning targeting inhibitors of microorganisms is often referred to as detoxification. There are several comparative studies of different conditioning methods (Larsson et al., 1999a; Cantarella et al., 2004; Guo et al., 2013; Fernandes-Klajn et al., 2018), among which treatment with calcium hydroxide (overliming) and treatment with activated charcoal (Parajó et al., 1997; Guo et al., 2013; Fernandes-Klajn et al., 2018) are some of the more common. More recently, conditioning using reducing agents has emerged as one of the most promising approaches (Alriksson et al., 2011). Treatment with reducing agents is advantageous as it is compatible with conditions suitable for enzymes and microbes and does not require a separate process step, relatively low concentrations of industrial chemicals can be used, and certain reducing agents, such as the sulfur oxyanions sulfite and dithionite, have positive effects on both cellulolytic enzymes and fermenting microorganisms (Alriksson et al., 2011; Cavka et al., 2011; Cavka and Jönsson, 2013). Techno-economic studies suggest that using sodium sulfite for biochemical conversion of softwood is advantageous (Cavka et al., 2015a).
Studies of conditioning with reducing agents have so far mainly addressed treatments of hydrolysates, as in an SHF approach. Therefore, it is important to address SSF and HHF scenarios by treatment of slurries and investigate if treatment of slurries and hydrolysates are different. Another important issue regards the WIS content, which is related to the inhibitor concentrations. Using highly concentrated systems to get higher sugar and ethanol concentrations is expected to also affect inhibitor concentrations and required dosages of chemicals. Another issue concerns comparison of industrial reducing agents. Sodium sulfite, sodium dithionite, and sodium borohydride (Alriksson et al., 2011; Cavka and Jönsson, 2013) need to be compared, and hydrogenation, which is an industrially important approach, is also a possibility to consider.
In this study we addressed that lack of knowledge using pretreated sawdust from softwood, more specifically Norway spruce (Picea abies). The first investigation covered how the content of WIS in the range 10–20% (w/v) was correlated to sodium sulfite and sodium dithionite loadings in an HHF process. Second, an investigation was made how sulfur oxyanions affected the solid phase and the enzymatic saccharification. Third, a comparison was made using different conditioning methods, including sodium sulfite, sodium dithionite, sodium borohydride, alkali treatment, activated charcoal, and hydrogen, and including both experiments with slurries and pretreatment liquids. Fourth, a comparison was made between different reducing agents on basis of efficiency and cost. Fifth, the impact of the different conditioning methods on monosaccharides and inhibitors in slurry and hydrolysate was investigated. Investigations in this area help to elucidate fundamental aspects of efficient bioconversion processes, and provide guidance for the development of competitive industrial processes.
Materials and Methods
Pretreatment of Raw Material
The severity factor (SF) (Eq. 1) of the pretreatments was determined as the logarithm of the reaction ordinate developed by Overend and Chornet (1987) (Eq. 2), and the combined severity factor (CSF) of the acid-catalyzed pretreatments was calculated as outlined by Chum et al. (1990) (Eq. 3):
where t is the residence time of pretreatment in minutes, and Tr is the pretreatment temperature in °C.
The pretreatment of sawdust from Norway spruce was performed by SEKAB E-Technology in the Biorefinery Demonstration Plant (BDP), Örnsköldsvik, Sweden. The demonstration unit has a pre-treatment capacity of approx. 2 ton of feedstock per day and is equipped with a continuous steam explosion pre-treatment reactor. The sawdust was impregnated with sulfur dioxide (approx. 0.5% w/w) prior to pretreatment and the subsequent pre-treatment was performed at a temperature, residence time and pH that resulted in a CSF of approx. 2.3 (temperature, 195°C; residence time, 9 min). The pretreated biomass was stored at 4°C until further use. The water-insoluble solids (WIS) was determined. The slurry was separated by vacuum filtration and then the solid phase was washed with approx. 1 L of deionized water and the dry-matter content was measured by using an HG63 moisture analyzer (Mettler-Toledo, Greifensee, Switzerland). The WIS content of the slurry was around 16.5% (w/v) and the pH was around 1.5.
Conditioning of Slurry
Prior to conditioning, the WIS content of the pretreated slurry was adjusted to 10, 15, and 20% (w/v) (see Experimental Design). The pH was adjusted to 5.5 using a 10 M aqueous solution of sodium hydroxide. Then the slurries were conditioned for 10 min at 23°C under magnetic mixing, using different methods as described in Section Experimental Design.
Saccharification and Fermentation
Hybrid hydrolysis and fermentation (HHF) experiments were performed using 250-ml Erlenmeyer flasks. Triplicates of each reaction mixture were included. After conditioning, the pH was, if needed, adjusted to 5.5. Enzymatic saccharification was initiated by addition of Cellic CTec2, procured from Sigma-Aldrich, at a dosage corresponding to 0.1 g enzyme preparation per gram of WIS. The flasks were incubated for 36 h at 50°C and 140 rpm in an orbital shaker (Kühner Lab-Therm LT-X, Adolf Kühner AG, Birsfelden, Switzerland). After 36 h, 1 mL nutrient solution [containing 150 g/L yeast extract, 75 g/L (NH4)2HPO4, 3.75 g/L MgSO4·7H2O, and 238.2 g/L NaH2PO4·H2O] and 1 mL yeast suspension was added to 48 mL of the enzymatically hydrolyzed slurry resulting in a total culture volume of 50 mL. The yeast was the industrial S. cerevisiae strain ethanol red (Fermentis, Marcq en Baroeul, France) and the initial yeast biomass concentration in each culture was 2 g/L. The flasks were sealed with parafilm (Pachiney Plastic Packaging Company, Chicago, IL, United States) and aluminum foil to prevent evaporation of ethanol during the process. The reaction mixture was then incubated for 60 h at 35°C and 170 rpm. Samples for analysis of glucose and ethanol were withdrawn after 0, 12, 24, 36, 48, and 60 h.
Experimental Design
Three series of experiments were performed. In the first series (A), the concentrations of sodium dithionite and sodium sulfite and the WIS content were varied (Table 1). The concentration ranges were selected on basis of previous experiments (Alriksson et al., 2011; Cavka et al., 2015b).
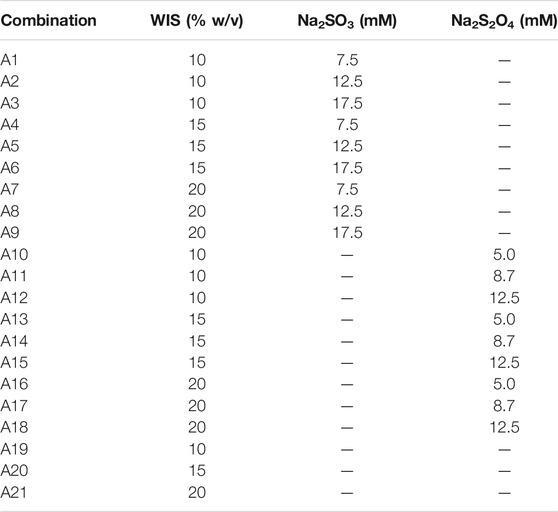
TABLE 1. Experimental conditions for Series A with varying concentrations of sodium dithionite and sodium sulfite and different content of WIS of pretreatment slurry.
The second experimental series (B) included six conditioning methods (Table 2). The WIS content was 15% (w/v). The sodium sulfite loading was determined on basis of the results from Series A. Loadings of sodium dithionite and sodium borohydride were decided based on molar masses, on weight, and on cost estimations. Treatment with calcium hydroxide (overliming) was performed by adjusting the pH of the slurry to 10.0 by adding calcium hydroxide. The reaction time was 1 h at 23°C with stirring. After that the pH was adjusted to 5.5 using a 10 M aqueous solution of sodium hydroxide. Treatment with activated charcoal (Norsk Turbokol, carbon size 0.4–0.8 mm) was performed by mixing different charges (1.25 g or 0.125 g activated charcoal per 50 ml slurry) of activated charcoal powder with the slurry (Table 2). Hydrogenation was performed by RISE Processum (Örnsköldsvik, Sweden) using a Hastelloy reactor from Büchi with a volume of 450 mL. Prior to hydrogenation, the pH of slurry was adjusted to 5.5 using a 10 M aqueous solution of sodium hydroxide. The reaction mixture contained slurry (B11 and C14) or filtrated liquid phase (C15). In each case, 300 g of material was weighed directly into the reactor. The catalyst, 3 g of 5% palladium on activated carbon paste type 395 (Johnson Matthey Plc, Hertfordshire, United Kingdom), was then added. The reactor was assembled and the stirring (1,000 rpm) was started. Hydrogen gas was added through the lid (from the top of the reactor without bubbling) to a pressure of 5.5 bar. The hydrogen gas line was held open during the reaction time to maintain the hydrogen gas pressure. The reaction time was 1 h. The pressure was decreased and the reactor was opened. The hydrotreated slurry was then used in the HHF experiments.
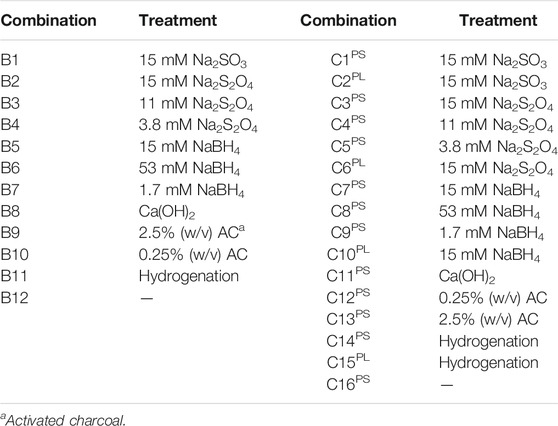
TABLE 2. Experimental conditions for Series B in which six conditioning methods for pretreatment slurry (15% WIS) were compared and experimental conditions for Series C including treatments of pretreatment slurry (PS) and pretreatment liquid (PL) with and without addition of sodium dithionite, sodium sulfite, sodium borohydride, calcium hydroxide, activated charcoal, and hydrogenation.
The third experimental series (C) consisted of treatments with sodium sulfite, sodium dithionite, sodium borohydride, alkali using calcium hydroxide, and hydrogenation of spruce pretreatment slurry (PS) and spruce pretreatment liquid (PL) (Table 2). The goal with the third experimental series was to compare the methods with regard to reactions with the solid and liquid phases.
Analysis of Glucose and Ethanol
Glucose and ethanol concentrations were determined using an HPLC instrument consisting of an Agilent (Santa Clara, CA, United States) 1260 Infinity system equipped with refractive index and diode array detectors, an autoinjector, and a column oven. The column was a Bio-Rad Aminex HPX-87H column (300 mm × 7.8 mm) equipped with a Bio-Rad 125-0131 Standard Cartridge Holder guard column (30 mm × 4.6 mm). The temperature of the column oven was set to 80°C and the temperature of the detector was set to 55°C. The injection volume was 10 µL and the flow rate was 0.6 mL/min. The vials that were used were 1.5 ml PP short Thread vials (32 × 11.6 mm) (Skandinaviska Genetec AB). The volume of the sample was 1.5 mL. The eluent consisted of a 0.005 M aqueous solution of H2SO4. The software was OpenLAB CDS Chem Station edition for LC and LC/MS Systems Rev.C.01.07(27).
Analytical Enzymatic Saccharification
Analytical enzymatic saccharification (Gandla et al., 2018) was used for evaluating the susceptibility of pretreated solids to enzymatic digestion. Prior to analytical enzymatic saccharification, slurries with 10, 15, and 20% (w/v) WIS were treated with 15 mM sodium sulfite or 15 mM sodium dithionite. Then, 50 mg (dry weight) of solid fraction washed with deionized water was suspended in 900 μL sodium citrate buffer (pH 5.2) and 50 μL of Cellic CTec2. Triplicates of each reaction mixture were prepared. The reaction mixtures were incubated in 2 mL microcentrifuge tubes in an Ecotron orbital incubator (Infors HT, Bottmingen, Switzerland) for 72 h. The temperature was set to 45°C, and the shaking to 170 rpm. The glucose released after 72 h was quantified by HPLC as previously described.
Determination of Sulfur Content
Untreated slurry and slurry treated with sodium dithionite or sodium sulfite was analyzed with respect to the sulfur content. After washing the solids with cold deionized water, the dried solid fractions were analyzed by RISE Research Institutes of Sweden AB (Borås, Sweden) using method SS-EN ISO 16994:2016 (Solid biofuels—Determination of total content of sulfur and chlorine).
Determination of Sugars and Microbial Inhibitors in the Liquids
The concentrations of HMF and furfural were determined using an Agilent 1,200 series HPLC system with diode-array detector and a 3 × 50 mm, 1.8 μm Zorbax RRHT SB-C 18 column. The temperature was set to 40°C. Isocratic elution was performed using an aqueous solution of 0.1% formic acid and 0.1% acetonitrile at a flow rate of 0.5 mL/min.
High-performance anion-exchange chromatography (HPAEC) with pulsed amperometric detection (PAD) was used to analyze monosaccharides. Prior to analysis all samples were diluted with ultra-pure water and filtered through 0.2 μm nylon membranes (Millipore). The separation system consisted of a separation column (4 × 250 mm), a guard column (4 × 50 mm) (both CarboPac PA1, Dionex), and an ICS-5000 system (Dionex, Sunnyvale, CA, United States). Elution was performed with ultra-pure water at a flow rate of 1 mL/min for 25 min. To amplify signal strength, post-column addition was made using a flow rate of 0.5 mL/min with a 300 mM aqueous solution of NaOH. Column regeneration was made during 11 min with a mixture consisting of 60% of the 300 mM NaOH solution and 40% of a 200 mM NaOH solution with 170 mM sodium acetate. Equilibration was made with ultra-pure water for 3 min. PAD was performed using a gold electrode with Gold Standard PAD waveform and Ag/AgCl as reference electrode.
Determination of aliphatic carboxylic acids (formic acid, acetic acid, and levulinic acid) was performed by MoRe Research (Örnsköldsvik, Sweden). The method used was HPAEC with a Dionex Ultimate 3000 system.
After derivatization with 2,4-dinitrophenylhydrazine (DNPH), ultra-high performance liquid chromatography-electrospray ionization-triple quadrupole-mass spectrometry (UHPLC-ESI-QqQ-MS) was used for determination of formaldehyde, p-benzoquinone, vanillin, coniferyl aldehyde, syringaldehyde, 4-hydroxybenzaldehyde, and acetovanillone. An Agilent 1290 Infinity system coupled to a 6490 triple-quadrupole mass spectrometer (QqQ-MS) was used with a 2.1 mm × 150 mm XTerra MS C18 column (Waters, Milford, MA, United States). The MS parameters were set as follows: gas temperature 290°C, gas flow 20 L/min, nebulizer 30 psi, sheath gas temperature 400°C, sheath gas flow 12 L/min, capillary voltage −3,000 V, and nozzle voltage −2,000 V. Eluents consisted of 1) a 0.1% (v/v) aqueous solution of formic acid, and 2) a 75:25 (v/v) mixture of acetonitrile and 2-propanol with 0.1% formic acid. Gradient elution was performed as previously described (Stagge et al., 2015).
The total concentration of phenolics was determined by using Folin-Ciocalteu’s reagent (Singleton et al., 1999). Vanillin was used as calibration standard. Analyses was performed using triplicates. The color generated after 2 h at ∼23°C was read at 760 nm in a microtiter plate in a Spectra Max i3X (Molecular Devices, LLC, San Jose, CA, United States).
The Total Aromatic Content (TAC) and the Total Carboxylic Acid Content (TCAC) were determined according to Wang et al. (2018). TAC, which is based on absorbance at 280 nm, was measured using a UV1800 spectrophotometer (Shimadzu, Kyoto, Japan). TCAC was measured by titrating from pH 2.8 to pH 7.0 using a 200 mM aqueous solution of NaOH.
Techno-Economic Evaluation
Three reducing agents, sodium sulfite, sodium dithionite, and sodium borohydride, were compared with regard to effect and economy. The comparison was centered on the dosage 15 mM (2.0 g/L) sodium sulfite and 15% (w/v) WIS, and covered molarity, mass, and estimated cost (Table 3). Thus, comparison on basis of molarity included 15 mM sodium dithionite and 15 mM sodium borohydride. Comparison on basis of mass included 2.0 g/L sodium dithionite (11 mM) and 2.0 g/L sodium borohydride (53 mM). The cost of treatment with 15 mM sodium sulfite (which was estimated to 0.013 USD per L of slurry) was estimated to correspond to the cost for treatment with 3.8 mM sodium dithionite or 1.7 mM sodium borohydride.
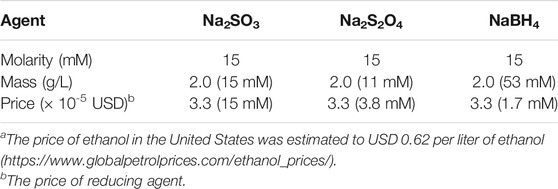
TABLE 3. Scheme for comparison of loadings of reducing agents (sodium sulfite, sodium dithionite, and sodium borohydride) based on molarity, mass, and cost (using 15 mM Na2SO3 as the reference case).a
Results and Discussion
Effects of Sulfur Oxyanions in Relation to the Content of Water-Insoluble Solids
Several previous studies have addressed conditioning of hydrolysates using reducing agents (Alriksson et al., 2011; Cavka and Jönsson, 2013; Cavka et al., 2015a,b), but conditioning of slurries and comparisons of conditioning of slurries and hydrolysates have not been well investigated. The first experimental series was devoted to how varying WIS content and concentrations of sulfite and dithionite affect the fermentability of spruce slurries (Table 1). Ethanol formation and residual glucose after 24 and 36 h of fermentation with S. cerevisiae are shown in Figure 1 for A1–A12 and A19-A21. Data on ethanol formation and residual glucose show good agreement (Figure 1). Without treatment, there was no glucose consumption or ethanol formation (A19–A21, Figure 1). Slurries with 15% or 20% WIS that were treated with 5.0–12.5 mM sodium dithionite (A13–A18) showed no ethanol formation or glucose consumption and are therefore not included in Figure 1. For A1-A12, treatments with the highest concentrations of sulfite (10–20% WIS, 17.5 mM) and dithionite (10% WIS, 12.5 mM) showed good results already after 24 h (Figure 1A). Also A2 (10% WIS, 12.5 sulfite) showed good results after 24 h. After 36 h, also A1 (10% WIS, 7.5 mM sulfite), A5 (15% WIS, 12.5 mM sulfite), and A11 (10% WIS, 8.7 mM dithionite) showed good results.
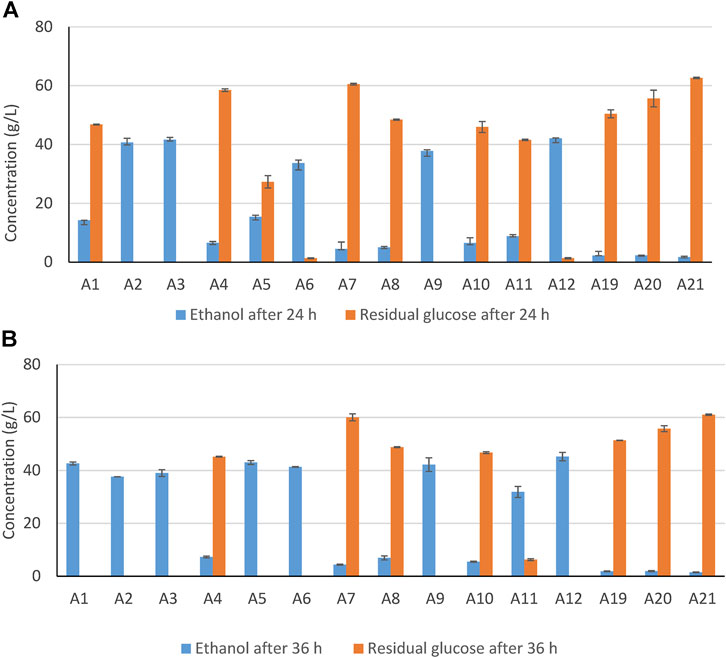
FIGURE 1. Concentrations of ethanol and residual glucose after 24 h (A) and 36 h (B) of HHF of Norway spruce slurry with sodium sulfite (A1–A9), with sodium dithionite (A10–A12), and without addition (A19–A21). Error bars indicate standard deviations.
It is noteworthy that A1 performed slightly better than A11, and A5 (15% WIS, 12.5 mM sulfite) performed clearly better than A15 (15% WIS, 12.5 mM dithionite). This is surprising, as previous work has indicated that dithionite have given a stronger effect than similar concentrations of sulfite (Alriksson et al., 2011). Conditioning works in an optimal way if the reagents are selective for the most toxic inhibitors. If there are side reactions, the reagents may be consumed without providing the desired effect. The difference between the potency of sulfite and dithionite in previous experiments with hydrolysates and the slurries in Series A in this work could potentially be attributed to that dithionite tend to be involved in side reactions if it is a slurry but not if it is a hydrolysate. Therefore, it is a possibility that dithionite was involved in side reactions with lignin in the solid phase. That would, however, be somewhat surprising considering the low concentrations used (5.0–12.5 mM) and the very mild reaction conditions (23°C and pH 5.5 for a few minutes).
Figure 2 shows the relationship between the concentration of reducing agent (sulfite or dithionite), the WIS content, and the fermentability expressed as glucose consumption during 24 h of fermentation. The graph indicates that a drastic improvement of fermentability occurred when the ratio of reducing agent (in mM)/WIS content (in %) reached the range 0.8–0.9. When that ratio was >1 the fermentability did not increase further than that observed for one of the treatments performed at ratio 0.9 (Figure 2). Thus, treatments with ratios as high as 1.8, as for one of the data points in Figure 2, clearly include utilization of excessive amounts of reducing agent.
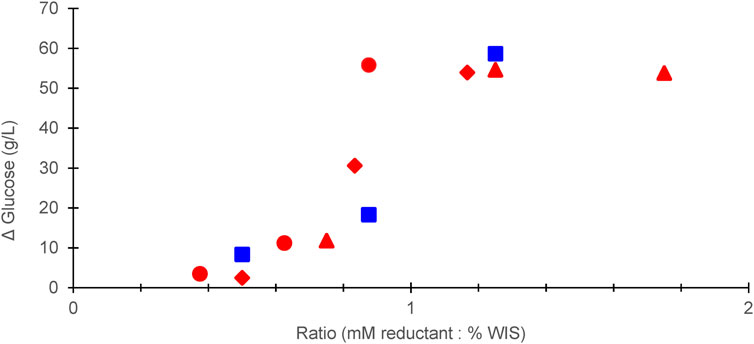
FIGURE 2. Glucose concentration after 24 h of enzymatic saccharification in relation to dosage of reductant expressed as the ratio of the reductant (in mM) to the WIS concentration (in %, w/v). The data indicate: sodium sulfite addition in 10% (w/v) (red triangles), 15% (w/v) (red diamonds), or 20% (w/v) (red circles) WIS slurry; sodium dithionite addition in 10% (w/v) (blue squares) WIS slurry.
Conditioning with reducing agents should not be mixed up with chemical-intensive processes carried out under harsher reaction conditions. The acid sulfite pulping process is typically carried out at 125–145°C for up to 7 h (Mboowa, 2021). The use of large amounts of cooking chemicals makes recovery of chemicals necessary. SPORL pretreatment involved sodium bisulfite loadings of 3–12%, and digester temperatures of 160–165°C (Zhou et al., 2018). Sulfite post-treatment involved sulfite loadings of 8–16% and temperatures in the range 70–160°C (Zhong et al., 2019).
Sodium dithionite is used in the pulp and paper industry for reductive bleaching of mechanical pulp. The treatment results in reduction of aldehydes and ketones to alcohols, and of quinones to hydroquinones (Rapson, 1969). Dithionite charges in the range 0.4–1.2% on air-dried pulp and temperatures in the range 50–70°C have been reported (Isoaho et al., 2019).
Effects of Sulfur Oxyanions on Solid Phase
Reactions with dithionite and sulfite could result in sulfonation of lignin derivatives (Cavka et al., 2011). As sulfonation would render lignin more hydrophilic, it could prevent catalytically non-productive adsorption of cellulolytic enzymes and thereby promote saccharification of cellulose. Analytical enzymatic saccharification and sulfur content determination (Table 4) were carried out to understand if there was a difference between dithionite and sulfite with respect to sulfonation reactions with lignin in the solid phase of the slurry. The experiment showed statistically significantly (p ≤ 0.01) higher glucose concentrations for all treatments with sodium sulfite or sodium dithionite (Table 4). Treatments with equimolar (15 mM) concentrations of sulfite and dithionite consistently resulted in larger saccharification improvements after dithionite treatment (18–24%) than after sulfite treatment (13–16%).
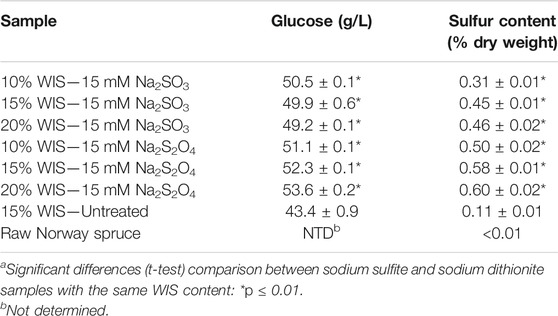
TABLE 4. Released glucose after 72 h of analytical enzymatic saccharification of buffered reaction mixtures containing 50 mg of pretreated solids originating from slurries conditioned with sodium dithionite or sodium sulfite, and sulfur content of solid fractions.a
Raw wood of Norway spruce contained negligible levels of sulfur, whereas the pretreated spruce wood contained low levels (0.11% w/w). All treatments with sulfite and dithionite resulted in statistically significant (p ≤ 0.01) increases of the sulfur content. Within each series with sulfite or dithionite, the sulfur content increased with the WIS content. However, the sulfur levels were consistently higher for dithionite-treated material (0.50–0.60%) than for sulfite-treated material (0.31–0.46%). Thus, the results of the sulfur content analysis agree with the results from analytical enzymatic saccharification and show that sulfonation occurs even under very mild reaction conditions (23°C, pH 5.5, 10 min, 15 mM). Reactions of sulfur oxyanions with the solid phase would be side reactions with regard to alleviating microbial inhibition, but this set of experiments demonstrates that the reagent is not lost in vain but becomes useful in another way; by promoting enzymatic saccharification.
Comparison of Effects and Costs of Conditioning Methods
Treatment with industrial reducing agents, such as sodium sulfite (Alriksson et al., 2011), sodium dithionite (Alriksson et al., 2011), and sodium borohydride (Cavka and Jönsson, 2013), can be compared with standard methods such as overliming and treatment with activated charcoal. Furthermore, hydrogenation is commonly used in industrial scale, and could be a potential alternative to previously studied reducing agents. An experimental series (B) was performed with slurries and different conditioning methods. The series was conducted with 15% WIS and 15 mM sodium sulfite as reference case, i.e., a (mM): (% WIS) ratio of 1.0. This series also included a comparison of sodium sulfite, sodium dithionite, and sodium borohydride based on molar concentration (15 mM), mass concentration (2 g/L), and estimated cost (3.3 × 10−5 USD per treated unit), as outlined in Table 3 and with 15 mM sodium sulfite as the reference case.
The results of the fermentation experiment for Series B are shown in Figure 3. Only the reference case with 15 mM sodium sulfite (B1) and the highest concentration of sodium borohydride (53 mM, B6) resulted in high ethanol titer. Treatments with the two highest concentrations of dithionite (15 mM, B2; 11 mM B3) and the highest loading of activated charcoal (2.5%, B9) resulted in at least some ethanol. The lowest concentration of dithionite (B4), the two lowest concentrations of borohydride (B5, B7), overliming (B8), the lowest loading of activated charcoal (B10), and hydrogenation (B11) were inefficient.
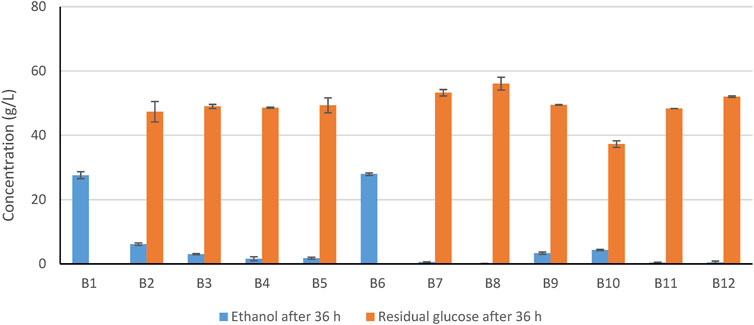
FIGURE 3. Concentrations of ethanol and residual glucose after 36 h of HHF of Norway spruce slurry using different methods (Table 2). Error bars indicate standard deviations.
The comparison of the three reducing agents (Figure 3; Table 3) used for treatment of the softwood slurry indicates that with regard to concentration based on molarity (15 mM), sodium sulfite was better than sodium dithionite, which in turn was better than sodium borohydride. With regard to concentration based on mass (2 g/L), sodium sulfite and sodium borohydride performed equally well. That resulted in sodium sulfite being superior with regard to lowest estimated cost. The treatment cost was estimated to 2% of the market price of ethanol.
The fact that some methods were inefficient in this comparative study does in no way imply that they are useless. For instance, overliming is one of the best studied conditioning methods and previous studies show that effects can be very good and that problems such as sugar degradation can be minimized through optimization of the conditions or by using alternative forms of alkali (Larsson et al., 1999a; Alriksson et al., 2006; Fernandes-Klajn et al., 2018). Activated charcoal has been shown to remove HMF, furfural, and phenolics, and can sometimes give good effects (Parajó et al., 1997; Guo et al., 2013; Fernandes-Klajn et al., 2018).
Comparison of Chemical Effects of Conditioning Methods
To better understand the underpinning chemistry behind the different effects of the conditioning methods and also to understand different effects on hydrolysates and slurries, a third experimental series, C, was conducted (Table 2). A drawback with some conditioning methods, such as overliming, is that they are not selective for inhibitors but also affect monosaccharide sugars. Therefore, potential effects on the five main monosaccharides were investigated (Table 5). As expected for pretreated softwood prior to enzymatic saccharification (Table 5), mannose (24.5 g/L) and glucose (21.6 g/L) were predominant, xylose was present in intermediate concentration (10.3 g/L), and galactose (4.0 g/L) and arabinose (2.3 g/L) concentrations were low. Data consistently show that for each monosaccharide C8PS, C9PS, C12PS, and C13PS always exhibited the lowest values. Compared to the control (C16PS), these four treatments resulted in 12–16% less glucose, 11–17% less xylose, 12–18% less galactose, 12–18% less mannose, and 13–17% less arabinose. Again, data are very consistent, and, regardless of the monosaccharide analyzed, the decreases were always in the interval 11–18%. For the sum of monosaccharides the decreases compared to the control were: C8PS, 17%; C9PS, 12%; C12PS, 13%; C13PS, 15%. Thus, treatments with sodium borohydride and activated charcoal, especially in large dosages, resulted in a substantial decrease in the content of monosaccharide sugars. All other methods exhibited values that were always within 10% of those of the control and sometimes no difference could be detected. The effect of sodium borohydride (Table 5) can be attributed to reduction of the five aldose sugars to alditol products (Abdel-Akher et al., 1951). Hydrogen would be expected to give the same effect (C15, C16), but hydrolysates and slurries are complex mixtures and competing reactions likely occur.
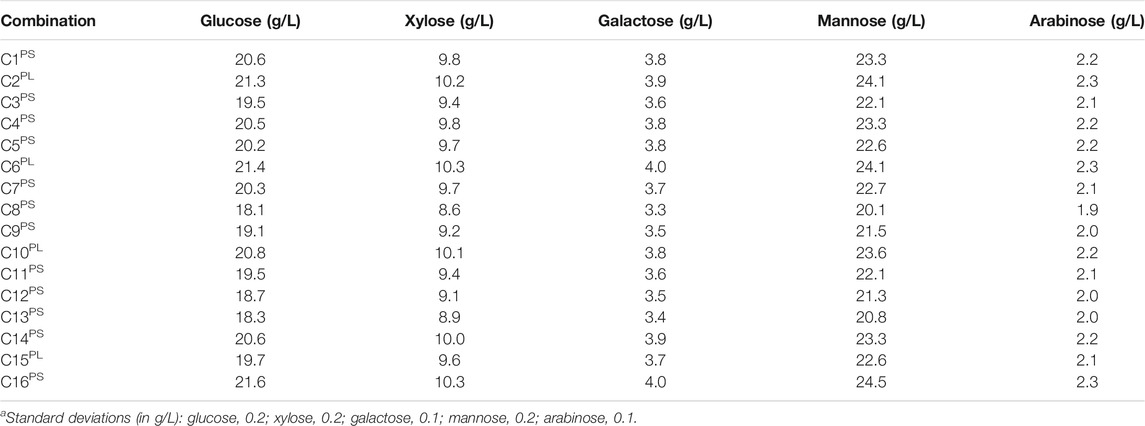
TABLE 5. Concentration of monosaccharides in the pretreated slurry (PS) and hydrolysate (PL) with and without addition of sodium dithionite, sodium sulfite, sodium borohydride, calcium hydroxide, activated charcoal, and hydrogenation.a
Figure 4 shows the concentrations of by-products in the liquid phase of pretreated spruce (C16) and the effects of the different conditioning methods on formation of inhibitory by-products (C1–C15). Acetic acid was the predominant aliphatic acid and accounted for 62% of the TCAC. Together with formic acid and levulinic acid, the share was 81% of the TCAC. When the concentrations of aliphatic carboxylic acids are above 100 mM, they can be expected to have an inhibitory effect on S. cerevisiae (Larsson et al., 1999b).
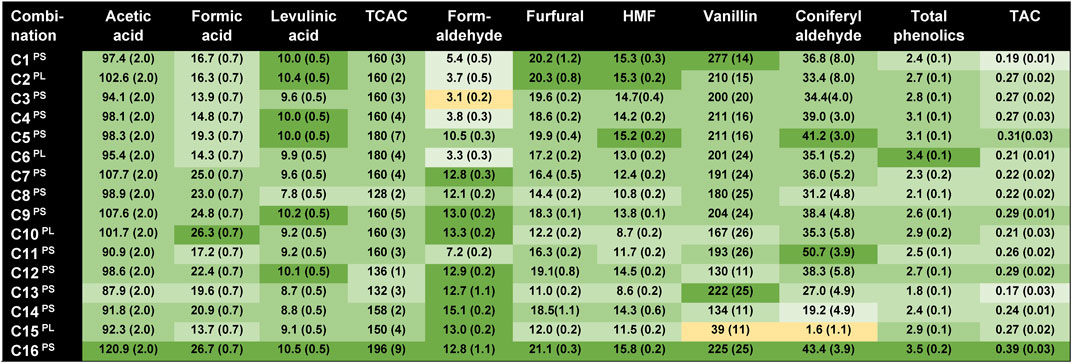
FIGURE 4. Measurements of bioconversion inhibitors in the pretreated slurry (PS) and hydrolysate (PL) with and without sodium dithionite, sodium sulfite, sodium borohydride, calcium hydroxide, activated charcoal, and hydrogenation. Concentrations of bioconversion inhibitors are indicated in mM, except for vanillin and coniferyl aldehyde (μM), total phenolics (g/L with vanillin as calibration standard), and TAC (UV absorption at 280 nm with a dilution factor of 500). The concentrations are mean values of three replicates, with standard deviations indicated in parentheses. Effects are color-coded in relation to C16PS (untreated reference), with lighter colors reflecting lower concentrations in relation to C16PS: (dark green) 95% or higher, (medium green) 75–94%, (light green) 50–74%, (very light green) 25–49%, and (yellow) < 25%.
The concentration of formaldehyde was exceptionally high, 12.8 mM (Figure 4). Cavka et al. (2015b) examined six pretreatment liquids from various feedstocks, found formaldehyde concentrations of up to 4.4 mM, and found that there were some inhibitory effects already at 1 mM. The concentrations of the carbohydrate-derived heteroaromatics furfural (21.1 mM, 2.03 g/L) and HMF (15.8 mM, 2.00 g/L) were relatively low. Due to low molar toxicity, high concentrations of furfural (31 mM) and HMF (39 mM) may be required for inhibitory effects (Wang et al., 2020).
Lignocellulosic hydrolysates contain a multitude of aromatic substances (Du et al., 2010), and the two ones included in Figure 4, the phenolics vanillin and coniferyl aldehyde, merely serve as examples. Vanillin and coniferyl aldehyde are guaiacyl-type phenolics derived from the guaiacyl lignin of the softwood raw material. p-Benzoquinone, 4-hydroxybenzaldehyde, syringaldehyde, p-coumaraldehyde, and acetovanillone were detectable, but they are not included, as the initial concentrations were very low. Vanillin, coniferyl aldehyde, and other phenolics are a part of the total phenolics and the TAC (Figure 4). Apart from phenolics, the TAC value also covers non-phenolic aromatics and heteroaromatics, such as furans.
The effects on by-products (Figure 4) on slurries and pretreatment liquids (hemicellulose hydrolysates) were fundamentally different from the effects on monosaccharides (Table 5). Effects on aliphatic acids and TCAC were generally small. Treatments with activated charcoal, particularly using high dosage (2.5%), resulted in comparatively large reductions of the concentrations of acids. The sulfur oxyanions, sulfite and dithionite, caused decreases in the concentration of formic acid that exceeded 25%.
The effects on formaldehyde were highly variable (Figure 4). Treatments with sulfite and dithionite decreased the concentration of formaldehyde to less than 50% of the original, except C5 for which the dithionite concentration was only 3.8 mM. The treatments of slurry or pretreatment liquid with 15 mM sulfite or dithionite resulted in 58–76% reduction of the original concentration of formaldehyde. The highest concentration of sodium borohydride (C8) and overliming (C11) also caused some decrease in the concentration of formaldehyde, whereas the other seven methods had no or negligible effects. It is particularly noteworthy that whereas sulfite and dithionite had large effects on formaldehyde, the other two reducing agents, sodium borohydride and hydrogenation, were not efficient. It is expected that there are similarities between sodium borohydride and hydrogenation, as sodium borohydride will form hydrogen gas when it comes into contact with water. Due to vigorous gas formation and bubbling leading to release of hydrogen gas into air, the sodium borohydride treatment was difficult to control.
The largest reductions (>25%) of the concentrations of furan aldehydes were observed for higher concentrations of sodium borohydride (C8, C10), high loading of activated charcoal (C13), and hydrogenation of pretreatment liquid (C15). The effects of sulfite and dithionite on the furan aldehydes were small, and always <25%. This observation agrees with previous studies of the effects of sodium sulfite and dithionite on spruce hydrolysates (Alriksson et al., 2011; Guo et al., 2013). In contrast, treatment with sodium borohydride and hydrogenation had large effects on furan aldehydes, especially in reaction mixtures with pretreatment liquid (C10 and C15). For C10, addition of 15 mM sodium borohydride resulted in a roughly equimolar decrease of furan aldehydes, as the combined concentration of furfural and HMF dropped from 37 to 21 mM. As seen previously (Table 5), reagents such as sodium borohydride may also be partially consumed by reactions in which aldose sugars are reduced to sugar alcohols. Thus, reactions with sugars and furans might explain the poor effect on formaldehyde of sodium borohydride and hydrogen. As furan aldehydes in C7 and C14 were less affected than in C10 and C15, the results also suggest that treatments with sodium borohydride and hydrogenation lead to further side reactions when solids are present in the reaction mixtures.
Hydrogenation was the most efficient approach for reducing the concentrations of vanillin and coniferyl aldehyde (Figure 4). Hydrogenation of pretreatment liquid (C15) even reduced the levels to <25%. This pattern was not reflected in the values for total phenolics (Figure 4). The reason for this is that hydrogenation (as well as other treatments with reducing agents, such as sodium borohydride) may reduce phenolic aldehydes to alcohols, which are less toxic (Larsson et al., 2000). However, even if alcohols are less toxic than their aldehyde counterparts they are still phenolics and would still be included in the values for total phenolics. Some treatments with sodium borohydride (C7, C8), overliming (C11), treatment with high loading of activated charcoal (C13), and hydrogenation of slurry (C14) reduced the level of total phenolics with <25%. The effects of alkaline conditions on inhibitors have been studied previously (Jönsson et al., 2013). Together with treatment with dithionite (C3), overliming was found to be the method that had broadest effect on inhibitor concentrations, causing clearly reduced levels of all individual inhibitors and groups of inhibitors investigated (Figure 4).
Many of the methods had a large impact on TAC (Table 5). Two of the methods, C1 (15 mM sodium sulfite) and C13 (high loading of activated charcoal), even reduced the TAC level with >50%.
Due to the unexpectedly large difference between sodium sulfite and sodium dithionite in experiments with slurry (Series A and B), the chemical effects of reducing agents in slurry and liquid were compared in Series C. The most obvious differences were observed for hydrogenation, for which reductions of aldehydes, such as furfural, HMF, vanillin, and coniferyl aldehyde, were larger for the pretreatment liquid than for the slurry. In several cases, there was a similar trend for the other reductants, although differences were smaller. Large differences between treatments of slurry and liquid suggest the occurrence of side reactions involving the solid phase and relatively poor selectivity for targeting microbial inhibitors in the liquid phase. Better selectivity can tentatively be achieved with somewhat weaker reducing agents, as stronger reducing agents may react with a wider array of substances in complex media. Such differences would be more difficult to detect in model experiments in which only one inhibitor is present at the time, indicating the need for comparative experiments with hydrolysates and slurries, as in this work.
Conclusion
The comparison of different conditioning methods for pretreated softwood, including four different industrial reducing agents, resulted in several new findings. Previous results had suggested that equimolar dosage of dithionite was superior to sulfite with regard to alleviation of microbial inhibition, but results with slurry indicated the opposite both with regard to effects and treatment costs. Chemical and biochemical analyses revealed that the sulfur oxyanions, and particularly dithionite, caused sulfonation of the solid phase of the pretreated biomass even when using low concentrations and very mild reaction conditions, something that had a positive effect on enzymatic saccharification. The sulfur oxyanions were found to efficiently reduce the concentration of formaldehyde in pretreated biomass, a phenomenon that has not been reported previously. That phenomenon is not self-evident considering that reaction patterns in authentic hydrolysates sometimes differ from what is observed in synthetic medium with only one inhibitor present at the time. The findings suggest that further research is warranted with regard to utilization of sulfur oxyanions to achieve optimal performance of enzyme preparations and microorganisms in pretreated lignocellulosic biomass.
Data Availability Statement
The original contributions presented in the study are included in the article/Supplementary Material, further inquiries can be directed to the corresponding author.
Author Contributions
Conceptualization, DI, BA, AC, and LJ; methodology, DI, SS, BA, and AC; validation, DI and SS; formal analysis, DI and SS; investigation, DI and SS; resources, BA, AC, and LJ; writing—original draft preparation, DI; writing—review and editing, LJ; visualization, DI and LJ; supervision, BA and LJ; project administration, LJ; funding acquisition, BA, AC, and LJ. All authors read and approved the manuscript.
Funding
This work was supported by Swedish Energy Agency (P41285-1, P47516-1), Kempe Foundations, and the strategic research environment Bio4Energy (www.bio4energy.se).
Conflict of Interest
Four of the authors (DI, BA, AC, and LJ) are co-inventors of patents/patent applications in the area biochemical conversion of biomass. One of the authors (AC) is an employee at SEKAB E-Technology AB, a company that is a technology provider in the biomass conversion area.
The remaining author declares that the research was conducted in the absence of any commercial or financial relationships that could be construed as a potential conflict of interest.
Publisher’s Note
All claims expressed in this article are solely those of the authors and do not necessarily represent those of their affiliated organizations, or those of the publisher, the editors and the reviewers. Any product that may be evaluated in this article, or claim that may be made by its manufacturer, is not guaranteed or endorsed by the publisher.
Acknowledgments
We thank SEKAB E-Technology for supplying the pretreated Norway spruce. We are grateful to Jonna Almqvist and David Blomberg Saitton at RISE Processum for their help with the hydrogenation experiment.
References
Abdel-Akher, M., Hamilton, J. K., and Smith, F. (1951). The Reduction of Sugars With Sodium Borohydride. J. Am. Chem. Soc. 73, 4691–4692. doi:10.1021/ja01156a501
Alriksson, B., Cavka, A., and Jönsson, L. J. (2011). Improving the Fermentability of Enzymatic Hydrolysates of Lignocellulose Through Chemical In-Situ Detoxification With Reducing Agents. Bioresour. Technology. 102, 1254–1263. doi:10.1016/j.biortech.2010.08.037
Alriksson, B., Sjöde, A., Nilvebrant, N.-O., and Jönsson, L. J. (2006). Optimal Conditions for Alkaline Detoxification of Dilute-Acid Lignocellulose Hydrolysates. Appl. Biochem. Biotechnol. 130, 599–611. doi:10.1385/abab:130:1:599
Cantarella, M., Cantarella, L., Gallifuoco, A., Spera, A., and Alfani, F. (2004). Comparison of Different Detoxification Methods for Steam-Exploded Poplar Wood as a Substrate for the Bioproduction of Ethanol in SHF and SSF. Process Biochem. 39, 1533–1542. doi:10.1016/s0032-9592(03)00285-1
Cavka, A., Alriksson, B., Ahnlund, M., and Jönsson, L. J. (2011). Effect of Sulfur Oxyanions on Lignocellulose-Derived Fermentation Inhibitors. Biotechnol. Bioeng. 108, 2592–2599. doi:10.1002/bit.23244
Cavka, A., and Jönsson, L. J. (2013). Detoxification of Lignocellulosic Hydrolysates Using Sodium Borohydride. Bioresour. Technology. 136, 368–376. doi:10.1016/j.biortech.2013.03.014
Cavka, A., Martín, C., Alriksson, B., Mörtsell, M., and Jönsson, L. J. (2015a). Techno-Economic Evaluation of Conditioning With Sodium Sulfite for Bioethanol Production From Softwood. Bioresour. Technology. 196, 129–135. doi:10.1016/j.biortech.2015.07.051
Cavka, A., Stagge, S., and Jönsson, L. J. (2015b). Identification of Small Aliphatic Aldehydes in Pretreated Lignocellulosic Feedstocks and Evaluation of Their Inhibitory Effects on Yeast. J. Agric. Food Chem. 63, 9747–9754. doi:10.1021/acs.jafc.5b04803
Chum, H. L., Johnson, D. K., Black, S. K., and Overend, R. P. (1990). Pretreatment-Catalyst Effects and the Combined Severity Parameter. Appl. Biochem. Biotechnol. 24-25, 1–14. doi:10.1007/bf02920229
Du, B., Sharma, L. N., Becker, C., Chen, S.-F., Mowery, R. A., van Walsum, G. P., et al. (2010). Effect of Varying Feedstock-Pretreatment Chemistry Combinations on the Formation and Accumulation of Potentially Inhibitory Degradation Products in Biomass Hydrolysates. Biotechnol. Bioeng. 107, 430–440. doi:10.1002/bit.22829
Fernandes-Klajn, F., Romero-García, J. M., Díaz, M. J., and Castro, E. (2018). Comparison of Fermentation Strategies for Ethanol Production from Olive Tree Pruning Biomass. Ind. Crops Prod. 122, 98–106. doi:10.1016/j.indcrop.2018.05.063
Galbe, M., and Wallberg, O. (2019). Pretreatment for Biorefineries: a Review of Common Methods for Efficient Utilisation of Lignocellulosic Materials. Biotechnol. Biofuels. 12, 294. doi:10.1186/s13068-019-1634-1
Gandla, M., Martín, C., and Jönsson, L. J. (2018). Analytical Enzymatic Saccharification of Lignocellulosic Biomass for Conversion to Biofuels and Bio-Based Chemicals. Energies. 11, 2936. doi:10.3390/en11112936
Guo, X., Cavka, A., Jönsson, L. J., and Hong, F. (2013). Comparison of Methods for Detoxification of Spruce Hydrolysate for Bacterial Cellulose Production. Microb. Cel Fact. 12, 93. doi:10.1186/1475-2859-12-93
Hoyer, K., Galbe, M., and Zacchi, G. (2009). Production of Fuel Ethanol From Softwood by Simultaneous Saccharification and Fermentation at High Dry Matter Content. J. Chem. Technol. Biotechnol. 84, 570–577. doi:10.1002/jctb.2082
Isoaho, J. P., Soininen, J. K., and Alén, R. J. (2019). Chemistry of Dithionite Bleaching. Part 1. Effect of Bleaching Variables. Appita J. 72, 197–210.
Jönsson, L. J., Alriksson, B., and Nilvebrant, N.-O. (2013). Bioconversion of Lignocellulose: Inhibitors and Detoxification. Biotechnol. Biofuels. 6, 16. doi:10.1186/1754-6834-6-16
Jönsson, L. J., and Martín, C. (2016). Pretreatment of Lignocellulose: Formation of Inhibitory By-Products and Strategies for Minimizing Their Effects. Bioresour. Technology. 199, 103–112. doi:10.1016/j.biortech.2015.10.009
Koppram, R., Tomás-Pejó, E., Xiros, C., and Olsson, L. (2014). Lignocellulosic Ethanol Production at High-Gravity: Challenges and Perspectives. Trends Biotechnol. 32, 46–53. doi:10.1016/j.tibtech.2013.10.003
Kristensen, J. B., Felby, C., and Jørgensen, H. (2009). Yield-Determining Factors in High-Solids Enzymatic Hydrolysis of Lignocellulose. Biotechnol. Biofuels. 2, 11. doi:10.1186/1754-6834-2-11
Larsson, S., Reimann, A., Nilvebrant, N.-O., and Jönsson, L. J. (1999a). Comparison of Different Methods for the Detoxification of Lignocellulose Hydrolyzates of Spruce. Appl. Biochem. Biotechnol. 77-79, 91–103. doi:10.1007/978-1-4612-1604-9_9
Larsson, S., Palmqvist, E., Hahn-Hägerdal, B., Tengborg, C., Stenberg, K., Zacchi, G., et al. (1999b). The Generation of Fermentation Inhibitors during Dilute Acid Hydrolysis of Softwood. Enzyme Microb. Technology. 24, 151–159. doi:10.1016/s0141-0229(98)00101-x
Larsson, S., Quintana-Sáinz, A., Reimann, A., Nilvebrant, N.-O., and Jönsson, L. J. (2000). Influence of Lignocellulose-Derived Aromatic Compounds on Oxygen-Limited Growth and Ethanolic Fermentation by Saccharomyces cerevisiae. Appl. Biochem. Biotechnol. 84-86, 617–632. doi:10.1385/abab:84-86:1-9:617
Mboowa, D. (2021). A Review of the Traditional Pulping Methods and the Recent Improvements in the Pulping Processes. Biomass Conv. Bioref. doi:10.1007/s13399-020-01243-6
Öhgren, K., Bura, R., Lesnicki, G., Saddler, J., and Zacchi, G. (2007). A Comparison Between Simultaneous Saccharification and Fermentation and Separate Hydrolysis and Fermentation Using Steam-Pretreated Corn Stover. Process Biochem. 42, 834–839. doi:10.1016/j.procbio.2007.02.003
Overend, R. P., and Chornet, E. (1987). Fractionation of Lignocellulosics by Steam-Aqueous Pretreatments. Phil. Trans. R. Soc. Lond. A321, 523–536. doi:10.1098/rsta.1987.0029
Parajó, J. C., Domínguez, H., and Domínguez, J. M. (1997). Xylitol Production from Eucalyptus wood Hydrolysates Extracted With Organic Solvents. Process Biochem. 32, 599–604. doi:10.1016/s0032-9592(97)00016-2
Ragauskas, A. J., Williams, C. K., Davison, B. H., Britovsek, G., Cairney, J., Eckert, C. A., et al. (2006). The Path Forward for Biofuels and Biomaterials. Science. 311, 484–489. doi:10.1126/science.1114736
Rasmussen, H., Sørensen, H. R., and Meyer, A. S. (2014). Formation of Degradation Compounds from Lignocellulosic Biomass in the Biorefinery: Sugar Reaction Mechanisms. Carbohydr. Res. 385, 45–57. doi:10.1016/j.carres.2013.08.029
Singleton, V. L., Orthofer, R., and Lamuela-Raventós, R. M. (1999). Analysis of Total Phenols and Other Oxidation Substrates and Antioxidants by Means of Folin-Ciocalteu Reagent. Methods Enzymol. 299, 152–178. doi:10.1016/s0076-6879(99)99017-1
Stagge, S., Cavka, A., and Jönsson, L. J. (2015). Identification of Benzoquinones in Pretreated Lignocellulosic Feedstocks and Inhibitory Effects on Yeast. AMB Express. 5, 62. doi:10.1186/s13568-015-0149-9
Wang, L.-Q., Cai, L.-Y., and Ma, Y.-L. (2020). Study on Inhibitors from Acid Pretreatment of Corn Stalk on Ethanol Fermentation by Alcohol Yeast. RSC Adv. 10, 38409–38415. doi:10.1039/d0ra04965d
Wang, Z., Wu, G., and Jönsson, L. J. (2018). Effects of Impregnation of Softwood With Sulfuric Acid and Sulfur Dioxide on Chemical and Physical Characteristics, Enzymatic Digestibility, and Fermentability. Bioresour. Technology. 247, 200–208. doi:10.1016/j.biortech.2017.09.081
Ximenes, E., Kim, Y., Mosier, N., Dien, B., and Ladisch, M. (2010). Inhibition of Cellulases by Phenols. Enzyme Microb. Technology. 46, 170–176. doi:10.1016/j.enzmictec.2009.11.001
Zhai, R., Hu, J., and Saddler, J. N. (2016). What Are the Major Components in Steam Pretreated Lignocellulosic Biomass that Inhibit the Efficacy of Cellulase Enzyme Mixtures?. ACS Sustainable Chem. Eng. 4, 3429–3436. doi:10.1021/acssuschemeng.6b00481
Zhong, N., Chandra, R., and Saddler, J. N. (2019). Sulfite Post-Treatment to Simultaneously Detoxify and Improve the Enzymatic Hydrolysis and Fermentation of a Steam-Pretreated Softwood lodgepole Pine Whole Slurry. ACS Sustainable Chem. Eng. 7, 5192–5199. doi:10.1021/acssuschemeng.8b06092
Keywords: lignocellulose bioconversion, enzymatic saccharification, hybrid hydrolysis and fermentation, conditioning, detoxification, inhibitor, sodium sulfite, sodium dithionite
Citation: Ilanidis D, Stagge S, Alriksson B, Cavka A and Jönsson LJ (2021) Comparison of Efficiency and Cost of Methods for Conditioning of Slurries of Steam-Pretreated Softwood. Front. Energy Res. 9:701980. doi: 10.3389/fenrg.2021.701980
Received: 28 April 2021; Accepted: 29 July 2021;
Published: 09 August 2021.
Edited by:
Richa Kothari, Central University of Jammu, IndiaReviewed by:
Sivakumar Nallusamy, Sultan Qaboos University, OmanZhaoxian Xu, Nanjing University of Science and Technology, China
Copyright © 2021 Ilanidis, Stagge, Alriksson, Cavka and Jönsson. This is an open-access article distributed under the terms of the Creative Commons Attribution License (CC BY). The use, distribution or reproduction in other forums is permitted, provided the original author(s) and the copyright owner(s) are credited and that the original publication in this journal is cited, in accordance with accepted academic practice. No use, distribution or reproduction is permitted which does not comply with these terms.
*Correspondence: Leif J. Jönsson, bGVpZi5qb25zc29uQHVtdS5zZQ==