- 1Soil Science Research Group, Agriculture Production Science Research and Development Division, Department of Agriculture, Ministry of Agriculture and Cooperative, Bangkok, Thailand
- 2Faculty of Environment and Resource Studies, Mahidol University, Bangkok, Thailand
- 3Division of Environmental Science and Engineering, Graduate School of Science and Engineering, Yamaguchi University, Yamaguchi, Japan
- 4Department of Biotechnology, Faculty of Technology, Khon Kaen University, Khon Kaen, Thailand
- 5Research Group for Development of Microbial Hydrogen Production Process From Biomass, Khon Kaen University, Khon Kaen, Thailand
- 6Academy of Science, Royal Society of Thailand, Bangkok, Thailand
The goal of this study was to evaluate the use of expanded clay as a support material for Thermoanaerobacterium thermosaccharolyticum KKU19 to produce hydrogen from oil palm trunk hydrolysate (OPT) and slaughterhouse wastewater (SHW) in a fixed-bed reactor (FBR) under non-sterile conditions. The effects of hydraulic retention time (HRT) on the performance of the FBR were also investigated. The FBR was operated at an OPT hydrolysate to SHW ratio of 2.55:1 (v:v), 60°C, initial pH 6.5, and 1.2 mg (as total volatile solids/g expanded clay) of T. thermosaccharolyticum KKU19 immobilized on expanded clay. A maximum hydrogen production rate (HPR) and hydrogen yield (HY) of 7.15 ± 0.22 L/L day and 234.45 ± 5.14 mL H2/g-COD, respectively, were obtained at an HRT of 6 h. Long-term operation of FBR at 6 h HRT indicated that expanded clay efficiently immobilizes T. thermosaccharolyticum KKU19, for which an HPR of 6.82 ± 0.56 L H2/L day, and an HY of 231.99 ± 19.59 mL H2/g-COD were obtained. Furthermore, the COD removal efficiency of 30% obtained under long-term operation was comparable to that under short-term operation at an HRT of 6 days. Butyric and acetic acids were the main soluble metabolite products, thereby indicating a butyrate–acetate type fermentation. Our findings indicate that expanded clay is an effective support material that contributes to the protection of microbial cells and can be used for long-term operation.
Introduction
The depletion of fossil fuels and severe environmental problems, particularly global warming, have driven the search for alternative energy sources (Vasiliadou et al., 2018; Kumar et al., 2019; Shetty et al., 2019). In this regard, hydrogen fits the bill perfectly, given its environmentally friendly characteristics, with water being the only by-product generated as a consequence of combustion with oxygen. Hydrogen has a high energy yield of 122 kJ/g, which is 2.75 times greater than that of hydrocarbon fuels (Cavalcante de Amorim et al., 2009). Biologically, hydrogen can be produced through photo-fermentation and dark fermentation processes. Compared with photo-fermentative hydrogen production, dark fermentative hydrogen production has the advantages of a higher hydrogen production rate (HPR), greater versatility regarding the substrates used, and can be performed in the absence of light (Xie et al., 2010). The hydrogen yield (HY) attained by dark fermentation can be enhanced by increasing hydrogen production through an acetic acid end-product reaction and by reducing or preventing butyric acid, lactic acid, and ethanol end-product reactions (O-Thong et al., 2008). Such modifications can be accomplished by operating a high-temperature fermentation process at temperatures greater than 60°C using thermophilic microorganisms as hydrogen producers. In this respect, it has been found that high temperatures increase the rate of biochemical processes, resulting in higher HY and fewer varieties of fermentation end-products than under mesophilic conditions (Prasertsan et al., 2009).
Hydrogen can be produced via dark fermentation using a diverse range of biomass. In Thailand, as a consequence of reduced productivity after 20–25 years, approximately 81 million m3 of relatively old palm trees are cut annually and discarded or burned at the plantation site (Department of Commercial Economics, 2010). The oil palm trunk (OPT) is an agricultural waste derived from the replanting of old oil palm trees, which comprises three main structural components, namely, cellulose (34.5%), hemicellulose (mainly xylan, 31.8%), and lignin (25.7%) (Kelly-Yong et al., 2007). These components can be liberated by acid hydrolysis and subsequently fermented by microorganisms to yield different chemicals. Dilute acid treatment of OPT yields a solution containing mainly glucose and xylose (Hniman et al., 2011), which can be used to produce hydrogen using different types of microorganisms. For example, Ren et al. (2008) have reported that the bacterium Thermoanaerobacterium thermosaccharolyticum strain W16 utilizes mixed xylose/glucose to produce hydrogen with an HY of 2.37 mol H2 mol/mol mixed-sugar, whereas Li et al. (2010) have demonstrated that an Δldh mutant of Thermoaotearoense produced an HY of 2.28 mol H2 mol/mol from mixed xylose/glucose. Given that the OPT hydrolysate comprises primarily cellulose, hemicellulose, and lignin, it has a high carbon content (carbohydrate) and a relatively low nitrogen content. Consequently, in order maximize hydrogen production, an appropriate nitrogen source is required for co-digestion with the OPT hydrolysate to obtain a suitable carbon-to-nitrogen ratio. For the purposes of co-digestion with OPT hydrolysate in the present study, we used slaughterhouse wastewater (SHW), which is considered a good potential source of nitrogen based on its primary composition of diluted and clotted blood, fat, and some manure.
Although fermentative hydrogen production is typically carried out in a continuously stirred tank reactor, the operation of such reactors at a low hydraulic retention time (HRT) generally leads to a washout of biomass, which in turn causes operational instability and inefficient hydrogen production (Cavalcante de Amorim et al., 2009). Consequently, enhancing cell retention under a low HRT could play a pivotal role in achieving a stable and efficient HPR (Cavalcante de Amorim et al., 2009). Several strategies have been proposed to enhance biomass retention for high-rate continuous hydrogen production, including cell-entrapment and cell-attachment methods (Chang et al., 2002; Jo et al., 2008; Leite et al., 2008; Peixoto et al., 2011). Fixed-bed reactors (FBRs) with attached biofilms have been widely used as biological treatment systems for wastewater with high efficiency and low HRT. The main consideration with respect to achieving high-performance FBRs with attached biofilms is the support material used, which ideally should be inexpensive and insensitive to abrasion. In this regard, a number of support materials for biomass retention have been examined for hydrogen production in FBRs with attached biofilms, among which are activated carbon (Chang et al., 2002) and expanded clay (Chang et al., 2002; Leite et al., 2008; Cavalcante de Amorim et al., 2009), and previous research has demonstrated that expanded clay is insensitive to abrasion and has high rugosity for biomass immobilization (Chang et al., 2002; Leite et al., 2008; Cavalcante de Amorim et al., 2009).
The goal of the present study was to evaluate the use of expanded clay as a support material for T. thermosaccharolyticum KKU19 to produce hydrogen from OPT hydrolysate co-digested with SHW in an FBR under non-sterile conditions. In addition, we also sought to investigate the effects of HRT on FBR performance. The results of this study will pave the way toward the development of an efficient hydrogen production process based on the use of OPT and SHW under non-sterile thermophilic conditions.
Materials and Methods
Feedstock Preparation
The OPT used in the present study was collected from the Faculty of Agriculture Farm, Khon Kaen University, Khon Kaen, Thailand, and subsequently processed by chopping with a knife, grinding using a food blender, and passing through a 2-mm screen. The ground OPT was treated with 1.56% (w/v) H2SO4 and heating in a microwave (Samsung/M1817) for 7.50 min at a fixed power of 450 W, following the method described by Khamtib et al. (2011). After pretreatment, the solid residue was separated by filtration through a thin-layer cloth. The pH of the hydrolysate was adjusted to 10 with Ca(OH)2, and the resulting precipitate was removed by centrifugation at 5,000 rpm for 15 min and then re-acidified to pH 7 using H2SO4, followed by further centrifugation using the aforementioned speed and time (Pattra et al., 2008).
The SHW was obtained from Nong Kae Municipal Slaughterhouse, Khon Kaen, Thailand. SHW was ground by a food blender and then filtered through a thin-layer cloth to remove any coarse particles. The processed OPT hydrolysate and SHW were stored at −20°C until used.
Fixed-Bed Reactor Set-Up
A schematic diagram of the FBR is depicted in Figure 1. The reactor was constructed of glass tubes with an internal diameter of 45 mm and a height of 440 mm. The reactor had a total volume of 700 mL, with a working volume (without support materials) of 541 mL. The fixed bed occupies the full active volume and the substrate was fed from the bottom using a peristaltic pump. The FBR temperature was maintained at 60°C by recirculating the 70°C water from a water bath through a water jacket.
Support Matrix
Expanded clay with a particle diameter of 2.5–3.5 mm was used as a support material for the attachment of biomass in the bed zone of the reactor, which contained 1,016 g of sterile expanded clay/L. The bed porosity (void fraction of the bed) was 47%.
Microorganism Preparation and Microbial Immobilization
The hydrogen-producer T. thermosaccharolyticum KKU19 (GenBank Accession No. JN020648) was isolated from hot spring sediment and identified based on 16S rRNA gene sequencing in our previous study (Khamtib and Reungsang, 2012). Stock cultures of T. thermosaccharolyticum KKU19 were maintained on agar slants [basal anaerobic (BA) medium (Angelidaki and Sanders, 2004) solidified with 3% (w/v) Gelrite (Kelo)] at 4°C and sub-cultured monthly. For the production of an inoculum, bacteria were cultivated in BA medium at 60°C for 24 h prior to use.
For the immobilization experiment, sterilized expanded clay was packed into the reactor. Sterile BA medium (225 mL) containing 20.00 g-chemical oxygen demand (COD)/L of mixed glucose-xylose (1:1) as a carbon source and 25 mL of T. thermosaccharolyticum KKU19 culture (density of 106 cells/mL) were fed into the FBR, and the reactor was then flushed with nitrogen gas through a 0.2 mm cellulose acetate air filter for 10 min to ensure anaerobic conditions. The reactor was initially operated in batch mode at 60°C for 24 h, and thereafter, fresh sterile BA medium containing 20.00 g COD/L of mixed glucose-xylose (1:1) was fed into the reactor and recirculated for 5 days to ensure adequate contact time between the fermentation broth and support materials for adhesion of the biomass (Leite et al., 2008). At the end of the recirculation period, the expanded clay was retrieved from the FBR to determine the concentration of the attached cells.
Start-Up and Operation of the FBR
During the 14-days start-up process at an HRT of 12 h, the FBR was fed with sterile medium containing 21.81 ± 1.56 g/L of total sugar in OPT hydrolysate supplemented with the following inorganic nutrients (all in g/L): 125 K2HPO4, 100 MgCl2⋅6H2O, 15 MnSO4⋅6H2O, 2.5 FeSO4⋅7H2O, 13.3 CuSO4⋅5H2O, and 1 mL/L of 0.125 CoCl2⋅5H2O solution. Following the startup period, the FBR was operated in continuous hydrogen production mode under non-sterile conditions at 60°C and an initial pH 6.5, using OPT hydrolysate and SHW at a ratio of 2.55:1 (v:v) (22.50 ± 3.41 g-COD/L) as the carbon source supplemented with 13.33 mg CuSO4/L (Khamtib and Reungsang, 2014). On reaching a steady state, as indicated by a constant HPR (variation within ±10% for 10 days), the HRT was reduced step-wise from 8 to 6, 4, and 2 h.
Analytical Methods
The volume of biogas produced was recorded daily using a gas meter based on the water displacement method (Owen et al., 1979). The hydrogen content in the biogas produced was determined using a gas chromatograph (GC-2014; Shimadzu, Tokyo, Japan) equipped with a thermal conductivity detector (TCD) and a 2-m stainless-steel column packed with Shin carbon (50/80 mesh), following the method described by Fangkum and Reungsang (2011). The concentrations of volatile fatty acids and alcohols in the effluent were measured by high-performance liquid chromatography (LC-10AD; Shimadzu, Kyoto, Japan) using an Aminex HPX-87H column (Bio-Rad Laboratories, Hercules, CA, United States), as described by Fangkum and Reungsang (2011). Total chemical oxygen demand, soluble chemical oxygen demand, total solids, total volatile solids, volatile suspended solids, and alkalinity were measured using standard methods (American Public Health Association, 1995), and carbohydrate and total nitrogen concentrations were determined using a colorimetric method (Dubois et al., 1956) and the persulfate method (Ameel et al., 1993), respectively.
Results and Discussion
The Effects of Hydraulic Retention Time on Hydrogen and Soluble Metabolite Product Production From the Co-digestion of Oil Palm Trunk Hydrolysate and Slaughterhouse Wastewater in a Fixed-Bed Reactor
The total carbohydrate concentration in the OPT hydrolysate was 19,218 ± 896 mg/L, whereas the total nitrogen concentration was relatively low (23 ± 1 mg/L) (Table 1). Main compositions in the OPT hydrolysate are (all in g/L): glucose, 8.95; xylose, 8.29; arabinose, 4.57; and acetic acid, 2.61 as previously reported in our research (Khamtib et al., 2011). SHW contains a higher total nitrogen content (1,560 ± 210 mg/L) than the OPT hydrolysate while the OPT hydrolysate contains a higher total carbohydrate concentration than the SHW (Table 1). A high nitrogen concentration in SHW is from the diluted and clotted blood and manure. Therefore, the co-digestion of OPT and SHW provided a balanced ratio of carbon to nitrogen which could enhance the production of hydrogen.
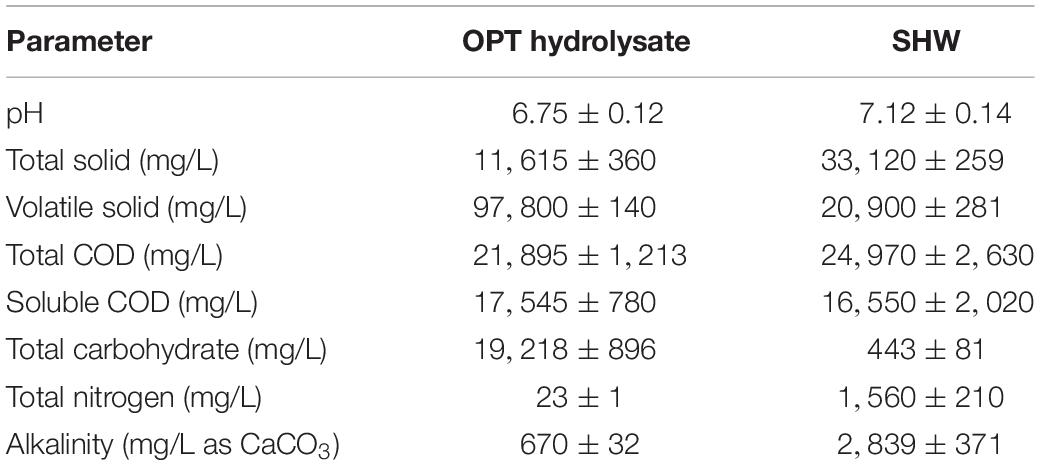
Table 1. Characteristics of oil palm trunk hydrolysate (OPT) hydrolysate and slaughterhouse wastewater (SHW).
Results obtained from assessments of the immobilization of T. thermosaccharolyticum KKU19 on expanded clay revealed that 1 g of expanded clay can immobilize 1.2 mg total volatile solids of this bacterium. A steady state was reached on day 7 after start-up (data not shown), at which time biogas production rate (BPR), hydrogen concentration, HPR, and HY values of 12.28 ± 0.68 L/L day, 42.17 ± 3.73%, 5.18 ± 0.47 L H2/L day, and 292.84 ± 17.95 mL H2/g-COD, respectively, were obtained. The main soluble metabolite products (SMPs) were acetic acid (2.85 ± 0.47 g/L) and butyric acid (2.73 ± 0.52 g/L). Subsequently, the FBR was operated at four different HRTs (8, 6, 4, and 2 h) corresponding to organic loading rate (OLRs) of 66.89 ± 1.18, 89.63 ± 1.08, 134.03 ± 2.11, and 267.12 ± 5.18 g COD/L day, respectively (Figure 2A and Table 2). In response to a reduction in the HRT from 8 to 6 h, we observed increases in the concentration of hydrogen in the biogas and COD removal (Figures 2B,C and Table 2). This can be attributed to an increase in the amount of substrate fed into the reactor, which in turn increases the activity of the hydrogen-producing bacteria, thereby enhancing fermentative hydrogen production (Chang et al., 2002). Contrastingly, we observed a slight reduction in biogas hydrogen concentration and COD removal when the HRT was reduced from 6 to 4 h (Figures 2B,C and Table 2). It is assumed that this reduction is associated with a higher substrate to microorganism ratio when the FBR is operated at a lower HRT, thereby exceeding the maximal efficiency of the hydrogen producers (Singh et al., 2013). Furthermore, significant reductions in biogas hydrogen concentration and COD removal were recorded when the HRT was reduced from 4 to 2 h (Figures 2B,C and Table 2). In this regard, Zulkhairi et al. (2010) have stated that a substantial shortening of the HRT is correlated with a very high OLR in the influent, resulting in the shock effect of OLR.
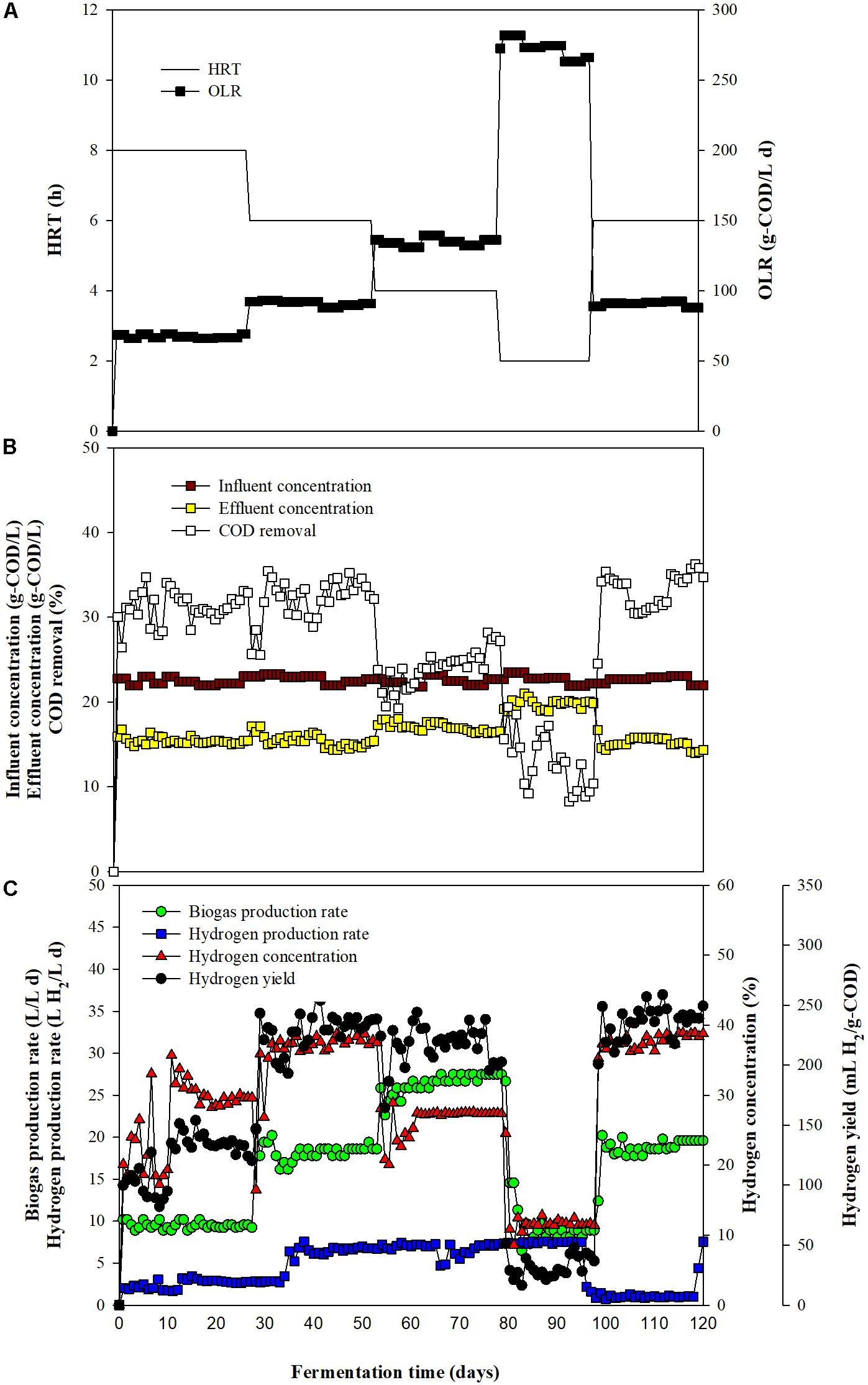
Figure 2. Effect of hydraulic retention time (HRT) on the performance of a fixed-bed reactor fed with oil palm trunk hydrolysate and slaughterhouse wastewater. (A) HRT and organic loading rate (OLR); (B) chemical oxygen demand (COD) removal, influent, and effluent concentrations, and (C) biogas production rate, hydrogen production rate, hydrogen concentration, and hydrogen yield.

Table 2. Performance of fixed-bed reactor (FBR) at various hydraulic retention time (HRT) (data are as mean ± SD, n = 10).
In response to a reduction in HRT from 8 to 6 h, we observed a corresponding increase in HPR from 2.75 ± 0.08 to 7.15 ± 0.22 L H2/L day, with production remaining relatively constant with a further reduction in HRT from 6 to 4 h. However, a subsequent reduction in HPR was observed when the HRT was reduced from 4 to 2 h (Figure 2C and Table 2). With respect to HY, we detected an increase from 130.90 ± 5.60 to 234.45 ± 5.14 mL H2/g-COD when the HRT was reduced from 8 to 6 h. However, there were reductions in HY when the HRT was less than 6 h (Figure 2C and Table 2). Accordingly, on the basis of these observations, we established that maximal values for HPR and HY of 7.15 ± 0.22 L/L day and 234.45 ± 5.14 L H2/L day, respectively, were obtained when operating the FBR at an HRT of 6 h, thereby indicating that this would be a suitable time for hydrogen production from OPT hydrolysate co-digested with SHW under non-sterile conditions in an FBR using expanded clay-immobilized T. thermosaccharolyticum KKU19.
With respect to hydrogen production, the observed differences in FBR performance at different HRTs can be explained in terms of SMP production. The SMPs obtained under steady-state conditions during continuous hydrogen fermentation at different HRTs are summarized in Table 2. Whereas we detected a slight increase in total SMPs in response to a reduction in HRT from 8 to 4 h (OLR from 66.89 ± 1.18 to 134.03 ± 2.11 g-COD/L day) (Table 2), when the HRT was further shortened from 4 to 2 h, there was a decline in production of approximately 43%. For each of HRTs assessed, we found that butyric and acetic acids, the detection of which is considered a reliable indicator of effective hydrogen fermentation (Lay, 2000; Pattra et al., 2008), were the primary metabolites, accounting for 67.35–75.65% of the total SMPs. Theoretically, 1 mol of glucose yields of 2 moles (Eq. 1) and 4 moles of hydrogen (Eq. 2) when butyrate and acetate are the main metabolic products, respectively (Lay, 2000).
The ratio of acetic acid to butyric acid can be used as an indicator of hydrogen production in acidogenesis systems (Peixoto et al., 2011), and in general, the higher the acetic acid/butyric acid ratio, the higher is the theoretical HY, according to stoichiometric Eqs (1) and (2) (Lay, 2000). In the present study, we found that at HRTs of 8 and 6 h, acetic acid was produced at higher rates (34.75 and 33.03% of the SMPs, respectively) (Table 2). Our observations indicated that the yields of acetic acid decreased concomitantly with a reduction in HRT, resulting in a reduction in the acetic acid/butyric acid ratio, despite a slight deviation in the percentage of butyric acid produced at HRTs of 4 and 2 h (Table 2).
The Effects of Long-Term FBR Operation on Hydrogen Production
Having optimized the operational HRT, the FBR was fed with OPT hydrolysate and SHW at an optimum HRT of 6 h. The performance of the FBR during long-term operation over a 70-days period is shown in Figure 3. In the early phase of operation (the initial 13 days), the BPR, hydrogen concentration, and COD removal fluctuated, as the reactor was switched from an HRT of 2–6 days. After 13 days, a steady state was reached, at which BPR, hydrogen concentration, HPR and HY values of 18.35 ± 1.07 L/L day, 37.12 ± 1.73%, 6.82 ± 0.56 L H2/L day, and 231.99 ± 19.59 mL H2/g-COD, respectively, were obtained (Figure 3A). The main SMPs were butyric acid (2.95 ± 0.46 g/L) and acetic acid (2.52 ± 0.71 g/L) (Figure 3B), thereby indicating the fermentative hydrogen reaction was a butyrate–acetate type fermentation.
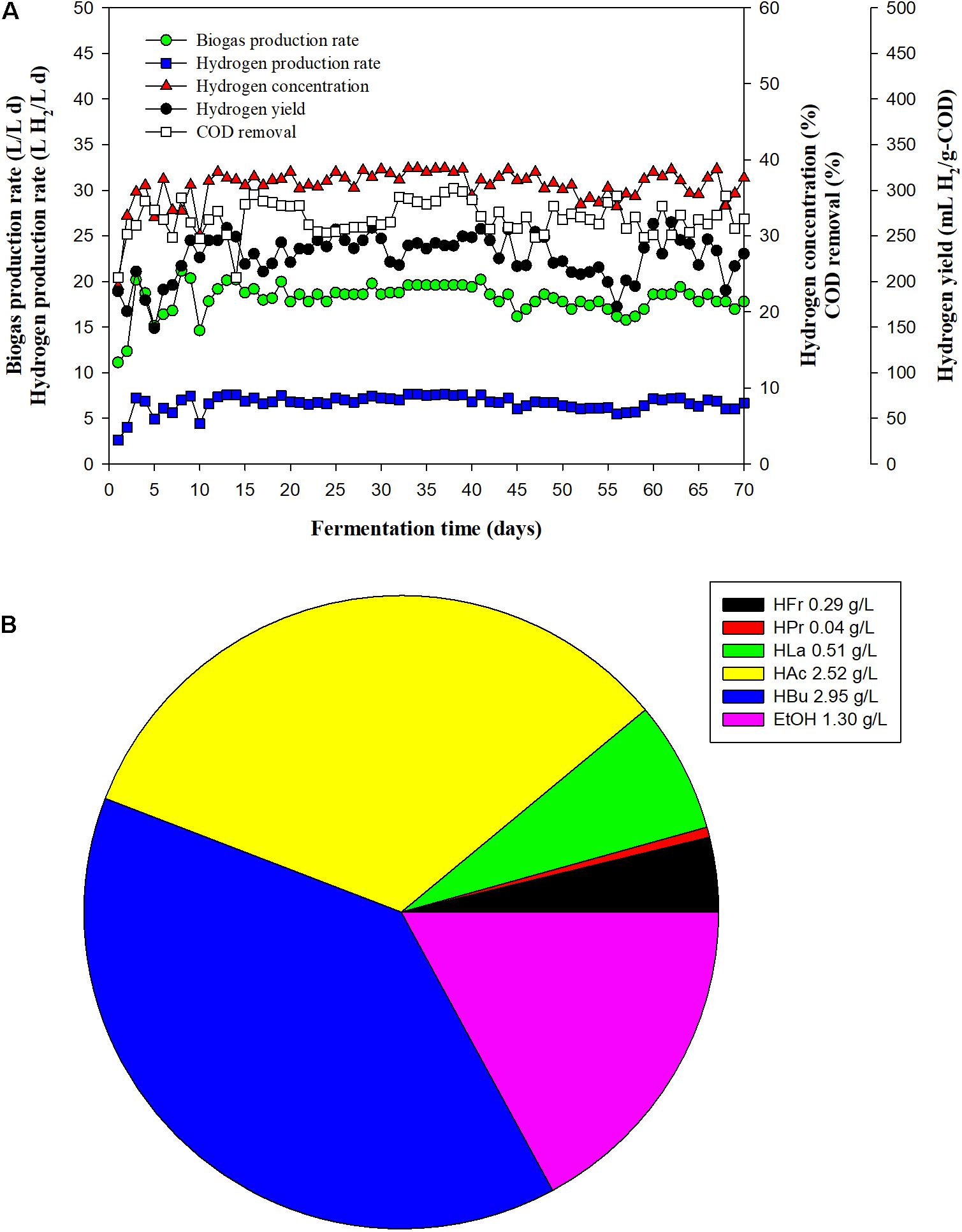
Figure 3. Fixed-bed reactor (FBR) performance with respect to (A) biogas production rate, hydrogen production rate, hydrogen concentration, hydrogen yield, and COD removal, and (B) soluble metabolite product production from the co-digestion of oil palm trunk hydrolysate and slaughterhouse wastewater in long-term operation of the FBR under an optimal hydraulic retention time (6 h).
From day 13–70 of operation, the COD removal efficiency was found to be relatively stable, with an estimated 30% COD removal being obtained over an extended period, which was comparable to that obtained during short-term operation at HRTs of 8 and 6 days. As a consequence, an HY of 231.99 ± 19.59 mL H2/g-COD was obtained. The long-term operation results revealed that the T. thermosaccharolyticum KKU19 immobilized on expanded clay successfully converted OPT hydrolysate and SHW to hydrogen. Our findings thus imply that expanded clay can serve as an efficient support material that affords protection to microbial cells and can be used for long-term operation.
Biofilm morphology was examined using scanning electron microscopy (JSM-5410LV; JEOL, Japan) (Figure 4), which revealed that the surface of the expanded clay had a rough uneven topography (Figure 4A), and given its high internal porosity (Figure 4C), this clay appears to be a good material for bacterial immobilization. Biofilm observations revealed that cells, mainly rod-shaped, covered the surface and cavities of the expanded clay, which is considered to be indicative of a high biomass retention capacity in the FBR (Figures 4B,D), resulting in stable hydrogen production. This is due to the expanded clay is resistant to abrasion and has a high rugosity for biomass attachment. However, unlike other supporting carriers, one of the most significant advantages of self-healing concrete as a bacteria carrier is its ability to withstand the internal environment (i.e., heat and pressure) (Stuckrath et al., 2014). These observations accordingly confirmed that expanded clay is a suitable carrier for the immobilization of T. thermosaccharolyticum KKU19 used to produce hydrogen from the co-digestion of OPT hydrolysate and SHW in an FBR.
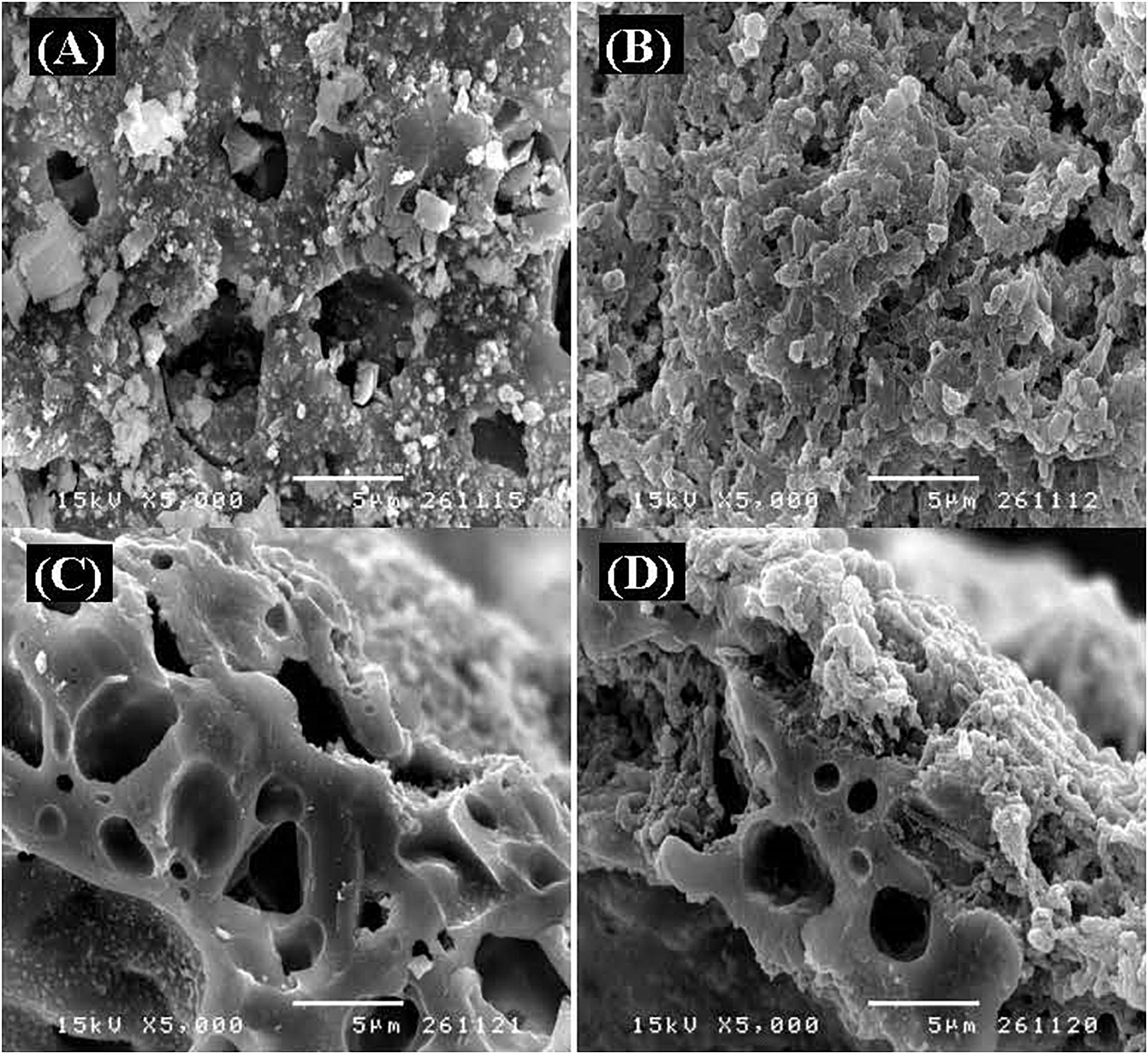
Figure 4. Scanning electron microscope studies of (A) the surface of expanded clay, (B) a biofilm on the expanded clay at the end of a long-term operation experiment, (C) a cross-section of the expanded clay, and (D) a cross-section of the biofilm on expanded clay at the end of a long-term operation experiment. Images at ×5,000 magnification were obtained using a JSM-5410LV electron microscope operating at 15 kV.
A comparison of the hydrogen concentrations obtained in the present study with those reported previously is presented in Table 3, which reveals that our findings are comparable to the concentrations reported by Chang et al. (2002), although lower than those obtained by Dos Reis and Silva (2014). However, given that the production of hydrogen is dependent on fermentation conditions, particularly temperature and nutrients, we should accordingly expect disparities among the findings of different studies that employ diverse types of substrate, inocula, and fermentation conditions.
Conclusion
In this study, expanded clay was successfully used as a support material to immobilize the bacterium T. thermosaccharolyticum KKU19 for the production of hydrogen from the co-digestion of oil palm trunk hydrolysate and slaughterhouse wastewater in a fixed-bed reactor. Hydraulic retention time plays an important role in hydrogen production, and we established a hydraulic retention time of 6 h to be optimal for hydrogen production from the co-digestion of oil palm trunk hydrolysate and slaughterhouse wastewater by expanded clay-immobilized T. thermosaccharolyticum KKU19 under non-sterile conditions in a fixed-bed reactor. Long-term operation results revealed that the immobilized T. thermosaccharolyticum KKU19 successfully converted oil palm trunk hydrolysate and slaughterhouse wastewater to hydrogen. Our findings thus indicate that expanded clay can serve as an effective support material affording protection to microbial cells within fixed-bed reactors and can be used for long-term operation.
Data Availability Statement
The original contributions presented in the study are included in the article/supplementary material, further inquiries can be directed to the corresponding author.
Author Contributions
SK: methodology, data analysis, investigation, and writing–original draft. SS: conceptualization, validation, data curation, writing–reviewing and editing. TI: visualization and resources. AR: conceptualization, project administration, supervision, writing–reviewing and editing, and funding acquisition. All authors contributed to the article and approved the submitted version.
Funding
This work was financially supported by Thailand Research Fund for a Ph.D. scholarship to SK through the Royal Golden Jubilee Ph.D. Program (Grant No. PHD/0107/2551), Senior Research Scholar, Thailand Science Research and Innovation (TSRI) (Grant No. RTA6280001).
Conflict of Interest
The authors declare that the research was conducted in the absence of any commercial or financial relationships that could be construed as a potential conflict of interest.
Acknowledgments
The authors acknowledge the National Research University Project through Biofuels Research Cluster-Khon Kaen University, Office of the Higher Education Commission.
References
Ameel, J. J., Axler, R., and Owen, C. J. (1993). Persulfate digestion for determination of total nitrogen and phosphorus in low-nutrient waters. Am. Environ. Lab. 10, 1–11.
American Public Health Association (1995). Standard Methods for Examination of Water and Wastewater. Washington DC: American Public Health Association.
Angelidaki, I., and Sanders, W. (2004). Assessment of the anaerobic biodegradability of macropollutants. Rev. Environ. Sci. Bio Technol. 3, 117–129. doi: 10.1007/s11157-004-2502-3
Cavalcante de Amorim, E. L., Barros, A. R., Rissato Zamariolli, Damianovic, M. H., and Silva, E. L. (2009). Anaerobic fluidized bed reactor with expanded clay as support for hydrogen production through dark fermentation of glucose. Int. J. Hydr. Energy 34, 783–790. doi: 10.1016/j.ijhydene.2008.11.007
Chang, J. S., Lee, K. S., and Lin, P. J. (2002). Biohydrogen production with fixed-bed bioreactors. Int. J. Hydr. Energy 27, 1167–1174. doi: 10.1016/s0360-3199(02)00130-1
Department of Commercial Economics (2010). Thailand Oil Palm Plantation [Online]. Available online at: http://www.oae.go.th/pdffile/indicator.pdf;2010. [accessed on Mar, 19 2010]
Dos Reis, C. M., and Silva, E. L. (2014). Simultaneous coproduction of hydrogen and ethanol in anaerobic packed-bed reactors. Bio Med. Res. Int. 2014:921291.
Dubois, M., Gilles, K. A., Hamilton, J. K., Rebers, P. A., and Smith, F. (1956). Colorimetric method for determination of sugars and related substances. Analyt. Chem. 28, 350–356. doi: 10.1021/ac60111a017
Fangkum, A., and Reungsang, A. (2011). Biohydrogen production from sugarcane bagasse hydrolysate by elephant dung: effects of initial pH and substrate concentration. Int. J. Hydrog. Energy 36, 8687–8696. doi: 10.1016/j.ijhydene.2010.05.119
Hniman, A., O-Thong, S., and Prasertsan, P. (2011). Developing a thermophilic hydrogen-producing microbial consortia from geothermal spring for efficient utilization of xylose and glucose mixed substrates and oil palm trunk hydrolysate. Int. J. Hydrog. Energy 36, 8785–8793. doi: 10.1016/j.ijhydene.2010.09.067
Jo, J. H., Lee, D. S., Park, D., and Park, J. M. (2008). Biological hydrogen production by immobilized cells of Clostridium tyrobutyricum JM1 isolated from a food waste treatment process. Bioresour. Technol. 99, 6666–6672. doi: 10.1016/j.biortech.2007.11.067
Kelly-Yong, T. L., Lee, K. T., Mohamed, A. R., and Bhatia, S. (2007). Potential of hydrogen from oil palm biomass as a source of renewable energy worldwide. Energy Policy 35, 5692–5701. doi: 10.1016/j.enpol.2007.06.017
Khamtib, S., Plangklang, P., and Reungsang, A. (2011). Optimization of fermentative hydrogen production from hydrolysate of microwave assisted sulfuric acid pretreated oil palm trunk by hot spring enriched culture. Int. J. Hydr. Energy 36, 14204–14216. doi: 10.1016/j.ijhydene.2011.05.117
Khamtib, S., and Reungsang, A. (2012). Biohydrogen production from xylose by Thermoanaerobacterium thermosaccharolyticum KKU19 isolated from hot spring sediment. Int. J. Hydr. Energy 37, 12219–12228. doi: 10.1016/j.ijhydene.2012.06.038
Khamtib, S., and Reungsang, A. (2014). Co-digestion of oil palm trunk hydrolysate with slaughterhouse wastewater for thermophilic bio-hydrogen production by Thermoanaerobacterium thermosaccharolyticm KKU19. Int. J. Hydr. Energy 39, 6872–6880. doi: 10.1016/j.ijhydene.2014.02.073
Kumar, D., Eswari, A. P., Park, J.-H., Adishkumar, S., and Banu, J. R. (2019). Biohydrogen generation from macroalgal biomass, Chaetomorpha antennina through surfactant aided microwave disintegration. Front. Energy Res. 7:78.
Lay, J. J. (2000). Modeling and optimization of anaerobic digested sludge converting starch to hydrogen. Biotechnol. Bioeng. 68, 269–278. doi: 10.1002/(sici)1097-0290(20000505)68:3<269::aid-bit5>3.0.co;2-t
Leite, J. A. C., Fernandes, B. S., Pozzi, E., Barboza, M., and Zaiat, M. (2008). Application of an anaerobic packed-bed bioreactor for the production of hydrogen and organic acids. Int. J. Hydr. Energy 33, 579–586. doi: 10.1016/j.ijhydene.2007.10.009
Li, S., Lai, C., Cai, Y., Yang, X., Yang, S., Zhu, M., et al. (2010). High efficiency hydrogen production from glucose/xylose by the ldh-deleted Thermoanaerobacterium strain. Bioresour. Technol. 101, 8718–8724. doi: 10.1016/j.biortech.2010.06.111
O-Thong, S., Prasertsan, P., Karakashev, D., and Angelidaki, I. (2008). Thermophilic fermentative hydrogen production by the newly isolated Thermoanaerobacterium thermosaccharolyticum PSU-2. Int. J. Hydr. Energy 33, 1204–1214. doi: 10.1016/j.ijhydene.2007.12.015
Owen, W. F., Stuckey, D. C., Healy, J. B., Young, L. Y., and Mccarty, P. L. (1979). Bioassay for monitoring biochemical methane potential and anaerobic toxicity. Water Res. 13, 485–492. doi: 10.1016/0043-1354(79)90043-5
Pattra, S., Sangyoka, S., Boonmee, M., and Reungsang, A. (2008). Biohydrogen production from the fermentation of sugarcane bagasse hydrolysate by Clostridium butyricum. Int. J. Hydr. Energy 33, 5256–5265. doi: 10.1016/j.ijhydene.2008.05.008
Peixoto, G., Saavedra, N. K., Varesche, M. B. A., and Zaiat, M. (2011). Hydrogen production from soft-drink wastewater in an upflow anaerobic packed-bed reactor. Int. J. Hydr. Energy 36, 8953–8966. doi: 10.1016/j.ijhydene.2011.05.014
Prasertsan, P., O-Thong, S., and Birkeland, N.-K. (2009). Optimization and microbial community analysis for production of biohydrogen from palm oil mill effluent by thermophilic fermentative process. Int. J. Hydr. Energy 34, 7448–7459. doi: 10.1016/j.ijhydene.2009.04.075
Ren, N., Cao, G., Wang, A., Lee, D.-J., Guo, W., and Zhu, Y. (2008). Dark fermentation of xylose and glucose mix using isolated Thermoanaerobacterium thermosaccharolyticum W16. Int. J. Hydrogen Energy 33, 6124–6132. doi: 10.1016/j.ijhydene.2008.07.107
Shetty, P., Boboescu, I. Z., Pap, B., Wirth, R., Kovács, K. L., Bíró, T., et al. (2019). Exploitation of algal-bacterial consortia in combined biohydrogen generation and wastewater treatment. Front. Energy Res. 7:52. doi: 10.3389/fenrg.2019.00052
Singh, L., Wahid, Z. A., Siddiqui, M. F., Ahmad, A., Ab Rahim, M. H., and Sakinah, M. (2013). Application of immobilized upflow anaerobic sludge blanket reactor using Clostridium LS2 for enhanced biohydrogen production and treatment efficiency of palm oil mill effluent. Int. J. Hydr. Energy 38, 2221–2229. doi: 10.1016/j.ijhydene.2012.12.004
Stuckrath, C., Serpell, R., Valenzuela, L. M., and Lopez, M. (2014). Quantification of chemical and biological calcium carbonate precipitation: performance of self-healing in reinforced mortar containing chemical admixtures. Cement Concrete Comp. 50, 10–15. doi: 10.1016/j.cemconcomp.2014.02.005
Vasiliadou, I. A., Berná, A., Manchon, C., Melero, J. A., Martinez, F., Esteve-Nuñez, A., et al. (2018). Biological and bioelectrochemical systems for hydrogen production and carbon fixation using purple phototrophic bacteria. Front. Energy Res. 6:107. doi: 10.3389/fenrg.2018.00107
Xie, G. J., Feng, L. B., Ren, N. Q., Ding, J., Liu, C., Xing, D. F., et al. (2010). Control strategies for hydrogen production through co-culture of Ethanoligenens harbinense B49 and immobilized Rhodopseudomonas faecalis RLD-53. Int. J. Hydr. Energy 35, 1929–1935. doi: 10.1016/j.ijhydene.2009.12.138
Keywords: bioenergy, dark fermentation, hydrogen, continuous process, hydraulic retention time
Citation: Khamtib S, Sittijunda S, Imai T and Reungsang A (2021) Co-digestion of Oil Palm Trunk Hydrolysate and Slaughterhouse Wastewater for Biohydrogen Production in a Fixed Bed Reactor by Immobilized Thermoanaerobacterium thermosaccharolyticum KKU19 on Expanded Clay. Front. Energy Res. 9:683989. doi: 10.3389/fenrg.2021.683989
Received: 22 March 2021; Accepted: 16 April 2021;
Published: 11 May 2021.
Edited by:
Hu Li, Guizhou University, ChinaCopyright © 2021 Khamtib, Sittijunda, Imai and Reungsang. This is an open-access article distributed under the terms of the Creative Commons Attribution License (CC BY). The use, distribution or reproduction in other forums is permitted, provided the original author(s) and the copyright owner(s) are credited and that the original publication in this journal is cited, in accordance with accepted academic practice. No use, distribution or reproduction is permitted which does not comply with these terms.
*Correspondence: Alissara Reungsang, YWxpc3NhcmFAa2t1LmFjLnRo