- 1International Institute for Carbon-Neutral Energy Research (WPI-I2CNER), Kyushu University, Fukuoka, Japan
- 2Institute of Mathematics for Industry (IMI), Kyushu University, Fukuoka, Japan
- 3Japan Science and Technology Agency (JST), ERATO, Adachi Molecular Exciton Engineering Project, Fukuoka, Japan
- 4State Key Laboratory of Polymer Physics and Chemistry, Changchun Institute of Applied Chemistry (CIAC), Chinese Academy of Sciences, Changchun, China
- 5Center for Organic Photonics and Electronics Research (OPERA), Kyushu University, Fukuoka, Japan
This paper proposes a novel conceptual system of optical wireless power transfer (OWPT) between objects, which is different from the existing OWPT systems such that a single device—an optical transceiver—is employed. This optical transceiver, which is capable of both absorbing and emitting light, is fabricated from a metal halide perovskite known for its superior features that can help significantly reduce the whole system size and cost. The proposed system contributes to realizing a thing-to-thing OWPT network, in which surfaces of objects/things are covered by perovskite transceivers (fully or partially), enabling them to wirelessly charge or discharge from the others.
1 Introduction
Recently, WPT has emerged as a promising and interesting approach for power supply without any cables or wires, due to many advantages it provides. First, WPT brings the convenience and comfort since we do not need to plug in wires/cables for charging our EVs or electronic devices, instead just park our EVs and put our devices near wireless charging equipment. Second, fire and explosion problems with wired charging can be avoided. Last but not least, WPT is a requisite for many scenarios where wired power transfer is expensive, difficult, dangerous, or impossible, e.g., in high-voltage power grids (Lu et al, 2018), space (NASA-USA, 2020a; JAXA-Japan, 2020), or deep water exploration missions. For example, very recently, a helicopter named Ingenuity, created by NASA-USA (2020b), was landed on Mars and will be tested to fly in Mars environment, which has a solar panel to get solar energy; hence, OWPT would be a promising solution to extend its exploration missions when solar energy is not directly available (see Figure 1 in Section 2 for an illustration).
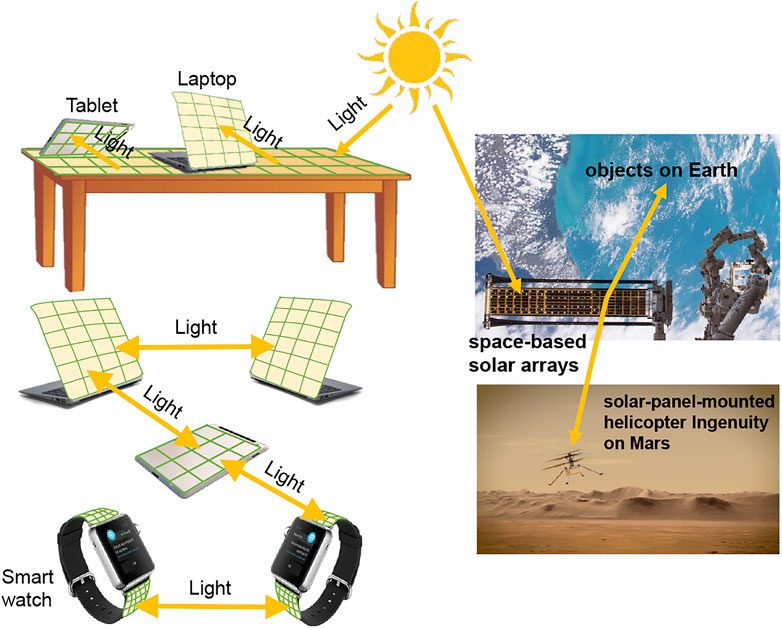
FIGURE 1. Envisioned illustration of the proposed conceptual OWPT system toward realization of a T2T-OWPT network (e.g., table–laptop, table–tablet, laptop–laptop, tablet–watch, and watch–watch bidirectional OWPT).
In the literature, different WPT technologies have been investigated, for example, IWPT and resonant IWPT (Li and Mi, 2015; Hata et al., 2018; Patil et al., 2018; Machura and Li, 2019), CWPT (Mostafa et al., 2017), and OWPT (Fakidis et al., 2016; Yoshida et al., 2016; Jin et al., 2018; Katsuta and Miyamoto, 2018; Jin and Zhou, 2019), and have been employed in various realistic systems. Among those WPT technologies, resonant IWPT has the highest PCE, but its transfer distance is limited. The PCE of CWPT is quite high; however, it causes strong side effects to humans nearby. The transfer distance of CWPT is also short. For longer energy transfer distances, OWPT is one of the most suitable approaches; therefore, it has been studied extensively in both academia and industry, e.g., Caltech (2020); FISE-Germany (2020); JAXA-Japan (2020); Wi-Charge (2020). In OWPT for long-distance applications (see, e.g., Fakidis et al., 2016; Katsuta and Miyamoto, 2018; Jin and Zhou, 2019), the laser beam is mostly used, but it is unsafe for humans, especially when exposed to eyes. On the contrary, LEDs are safer to be utilized in OWPT systems, but the generated light from them is more diverse than that from laser beams, leading to decreased efficiency and transfer distance. Hence, there is always a trade-off between efficiency and safety in OWPT systems when using lasers and LEDs.
In most of the existing OWPT studies, both the wireless energy transmitter and receiver or one of them is stationary. Thus, the energy mobility and availability as well as the convenience and comfort of users have not been fully exploited. For instance, the proposed system in Wi-Charge (2020) can charge one or multiple devices as they are moving, but the transmitter is fixed on the ceiling; hence, those devices need to be kept around the transmitter. Our recent work (Nguyen, 2020) showcased a simple proof-of-concept for OWPT between objects that are all moving. Nevertheless, in all existing OWPT systems, whetherthe energy transfer is only unidirectional, or two separated devices (e.g., a solar panel and an LED in Nguyen (2020)) need to be utilized for each object for bidirectional energy transfer between them.
To overcome the above limitations of the existing OWPT studies, the current research proposes a novel, conceptual OWPT system, in which a metal halide perovskite transceiver is employed for both absorbing light from and emitting light to other OWPT systems equipped with similar perovskite transceivers. As a result, each OWPT system has a single optical transceiver for both transmitting and receiving energy, i.e., for performing bidirectional WPT. Furthermore, this concept allows the bidirectional OWPT to be conducted between different objects, whether they are stationary or in motion, because such system can be switched from the energy reception mode to the energy transmission mode anywhere, at any time. On the contrary, bidirectional WPT systems using other WPT technologies have recently been introduced, e.g., those using IWPT implemented in smartphones, but again, both objects need to be stationary. Note also that our proposed OWPT system is certainly able to harvest solar energy or energy from other lighting resources. Therefore, the issues of energy ultra-mobility and availability, user convenience, and user comfort can be addressed by our proposed perovskite-transceiver–based OWPT system. This system, if realized, will contribute a sustainable and green energy technology to the society.
It is worth noting that although our proposed OWPT system is conceptual, initial experimental results will be introduced to demonstrate such concept. To fully realize the proposed system, much more work is needed; however, it is promising since research progresses in perovskite solar cells and perovskite LEDs are very fast.
The rest of this paper is organized as follows. Section 2 introduces our proposed bidirectional OWPT using perovskite transceivers for realization of a T2T-OWPT network, remarkable properties of perovskite-based devices, and how they are used as optical transceivers in the proposed OWPT system, supported by experimental results and discussions on comparison with other WPT systems. Then, the overall system QE is characterized and its experimental results are presented in Section 3, together with its affecting factors. Lastly, Section 4 concludes our study and provides discussions on the usefulness of the proposed system, as well as directions for future research.
2 Proposed OWPT System and Results
Figure 1 depicts the proposed OWPT system composed of basic elements such as devices whose surfaces are covered with optical transceivers and a transmission environment. The optical transceivers are made from a metal halide perovskite whose properties will be introduced in the next section. The transmission environment could be air, water, sea water, or outer space, depending on specific situations on which WPT is needed. Having the nice features of bidirectional transfer and ultra-mobility, the proposed OWPT system serves as an important step toward realization of the so-called T2T-OWPT network, in which energy can be ubiquitously exchanged anywhere and anytime between multiple objects/things equipped with perovskite transceivers, whether stationary or moving.
Note that the proposed conceptual OWPT system using a single optical transceiver for both emitting and absorbing light might be realized using other materials for the optical transceiver. For example, organic materials could be used, but their spectral overlap of light emission and absorption is small, i.e., the Stokes shift is large in organic materials. Meanwhile, it is currently difficult to find inorganic materials that can both emit and absorb light at high efficiency and low cost, like perovskites. Thus, a metal halide perovskite is proposed in the current research for fabricating optical transceivers.
On the contrary, it is certainly possible to create OWPT systems using separated devices for light emission and absorption (see, e.g., Hirukawa et al., 2019). However, in order to realize bidirectional OWPT between objects, both of those devices must be installed in every object, potentially leading to increased size, weight, and production cost and more complex electronic circuits. Additionally, those devices are not easy to be attached with curved surfaces. Hence, the current research proposes to use a single device—an optical transceiver made from a metal halide perovskite to overcome the above drawbacks. As a trade-off, the overall system efficiency of our proposed system is lower than those using separated devices for emitting and absorbing light, as will be discussed in more detail in Section 3.4.
To this end, it should be noted that the proposed OWPT system in this research is conceptual at this moment due to the limitation on the durability in the light emission mode of currently available perovskite solar cells, i.e., perovskite LEDs. However, the proposed system serves as the first step for a realistic realization of such OWPT systems in the future.
2.1 Light Receiver Mode
Metal halide perovskites have many advantages such as high carrier mobility, large carrier diffusion lengths, high absorption coefficients, bandgap tunability, thin and light weight, and compatibility with low-cost solution processing. These advantages make perovskites suitable for use in solar cells and can be flexibly attached on curved surfaces. Since the first discovery of perovskite solar cells in 2009 (Kojima et al., 2009), their performance has rapidly been increased. The highest certified PCE of perovskite solar cells has reached 25.5% (National Renewable Energy Laboratory-USA, 2020) which is comparable to that of silicon solar cell technology. Our group in Kyushu University has investigated perovskite solar cells to improve their PCEs and operational durability (see, e.g., Qin et al., 2016; Qin et al., 2017; Qin et al., 2019; G. Tumen-Ulzii et al., 2020; Tumen-Ulzii et al., 2020). Our perovskite solar cells reported in Qin et al. (2019) had an architecture of glass substrate/indium tin oxide (ITO) electrode/poly(3,4-ethylenedioxythiophene):poly-(styrenesulfonate) (PEDOT:PSS) hole transport layer/MA0.6 FA0.4 PbI2.8 Br0.2 perovskite light absorber (MA is methylammonium and FA is formamidinium)/C60 electron transport layer/2,9-dimethyl-4,7-diphenyl-1,10-phenanthroline (BCP) electron transport layer/Au electrode.
In this light absorption working mode, the system operation is illustrated in Figure 2A. Note that what are shown in Figure 2 are just for illustration purposes, not exactly the same as in real systems. IPCEs of our perovskite solar cells are measured and will be discussed in the next section.
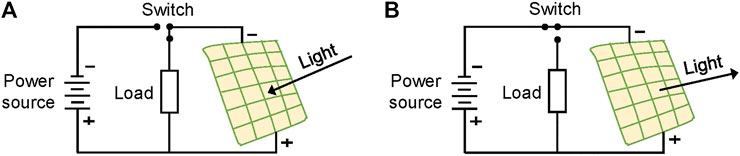
FIGURE 2. Demonstrated circuit diagram of the proposed OWPT system with a perovskite transceiver: (A) receiver mode; (B) transmitter mode.
Detailed fabrication conditions of our MA0.6 FA0.4 PbI2.8 Br0.2 perovskite films and solar cells are described in the Appendix. Although we already reported perovskite films and solar cells of the same composition in Qin et al. (2019), we briefly introduce their morphological, structural, and optical properties and solar cell performance as follows: MA0.6 FA0.4 PbI2.8 Br0.2 perovskite films were polycrystalline, with grains of hundreds of nanometers in diameter, and had the pure tetragonal phase (Qin et al., 2019). The absorption onset wavelength is
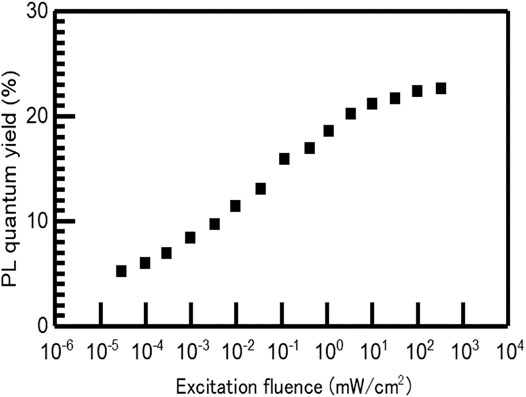
FIGURE 4. Plot of PL quantum yields as a function of excitation fluence for MA0.6 FA0.4 PbI2.8 Br0.2 perovskite films.
2.2 Light Transmitter Mode
Perovskites are known to have the large spectral overlap between emission and absorption, as shown in Figure 3. When a high voltage is applied to a perovskite solar cell, electrons and holes were injected from electrodes and recombined in the perovskite layer, which is a completely opposite process compared with that in solar cells. As a result, the perovskite solar cells began to emit light, i.e., they started working as light transmitters. Therefore, by taking advantage of efficient photoluminescence, perovskites can be employed as the emitter of efficient LEDs, i.e., a light transmitter in an OWPT system. Recently, very high EQEs of over 20% have been demonstrated from perovskite LEDs, see, e.g., Cao et al. (2018); Li et al. (2018); Xu et al. (2019). The operation principle of our proposed OWPT in the light-emitting mode is demonstrated in Figure 2B.
To make our perovskite solar cell emit light, a voltage is applied to it as mentioned above, which can be adjusted stepwise until obtaining the light emission (see Figure 6). The driving voltages to induce EL are below 10 V, as depicted in Figures 6, 7. Then, Figure 8A displays a photograph of our 2 mm × 2 mm perovskite solar cell emitting NIR light under electrical excitation, which was used in our experiments. This emission of NIR light is useful for OWPT systems because NIR light is invisible and not so harmful to humans compared with other higher energy lights such as ultraviolet or visible lights.
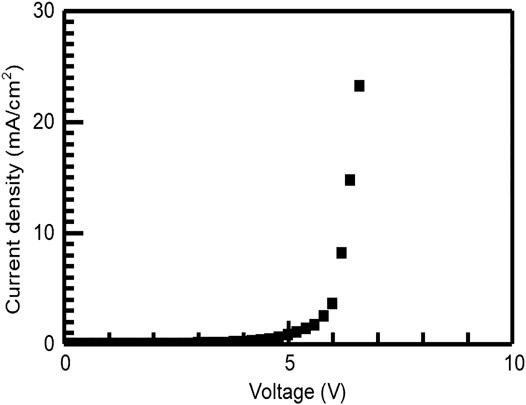
FIGURE 6. Current density–voltage curve of MA0.6 FA0.4 PbI2.8 Br0.2 perovskite solar cells operating in the light-emitting mode.
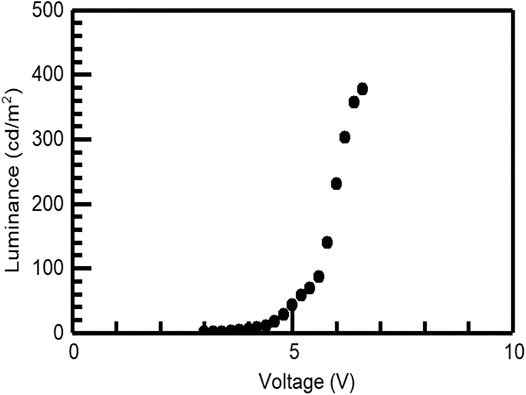
FIGURE 7. Luminance–voltage curve of MA0.6 FA0.4 PbI2.8 Br0.2 perovskite solar cells operating in the light-emitting mode.
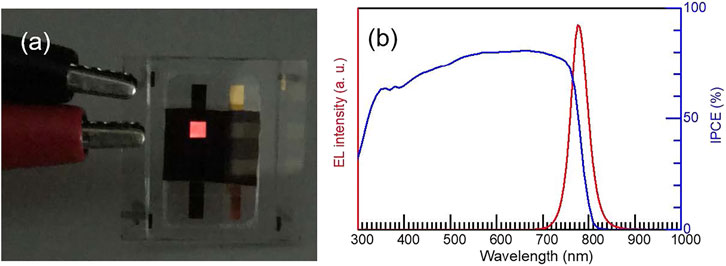
FIGURE 8. (A) Photograph of an NIR light–emitting MA0.6 FA0.4 PbI2.8 Br0.2 perovskite solar cell. (B) EL spectrum and IPCE curve of the same device.
As displayed in Figure 8B, our perovskite solar cell exhibits an EL peak at 790 nm, which overlaps with the IPCE curve. Moreover, this perovskite cell shows light emission near the band edge. Emission at longer wavelengths is possible by utilizing smaller bandgap perovskites such as tin iodide–based perovskites (P. Šcajev et al., 2019). These features clearly show that perovskites can be utilized as both the light absorber of solar cells (optical receivers for OWPT systems) and the emitter of LEDs (optical transmitters for OWPT systems) simultaneously in a single-device architecture, i.e., an optical transceiver.
Note, however, that one limitation of all perovskite LEDs so far is their limited durability. This certainly affects the operation of perovskite-based optical transceivers, but we believe that the durability of perovskite LEDs will be significantly improved soon. In what follows, we demonstrate the optical wireless energy transmission between two identical perovskite transmitters. First, a perovskite transmitter is operated in the light-emitting mode, where pulsed voltages of 7 V with a width of 10 ms and a duty cycle of 50% are used to suppress the device degradation. Consequently, the emitted EL from that perovskite transmitter is detected by a silicon photodiode combined with an oscilloscope, which is exhibited in Figure 9. Second, another perovskite transmitter is placed in front of the first perovskite transmitter above instead of the silicon photodiode to detect EL. The distance between two perovskite transmitters is
3 Efficiency of Proposed OWPT System
3.1 Computation of System QE
The OWPT system QE, denoted
where
In the current context of computing system QE,
Remark 1: If PCE is considered for the proposed perovskite-transceiver–based OWPT system, then
3.2 Experimental QEs of Perovskite Transceiver
The experimental
Consequently,
Detailed reciprocal relations between EQE and EL emission were shown in Rau (2007) with rigorous mathematical derivations, which are useful for further studying and improving the optical transceivers in OWPT systems. In particular, the direct relation between
At higher wireless energy transmission distances, the value of
3.3 Calculation of System PCE
When perovskite LEDs are stable enough (in the future), similar experiments like those reported in Nguyen (2020) using usual LEDs can be conducted to verify how the overall perovskite-transceiver–based OWPT system PCE depends on the transmitting distance. In what follows, we briefly revisit the theoretical and practical ways to derive the PCE of OWPT.
Theoretically, the PCE of an OWPT system, denoted
where
in which A is the physical area of the optical receiver, d is the optical transmission distance, φ is the angle of incidence (i.e., the angle at which the receiver sees the transmitter), ϕ is the angle of irradiance (i.e., the angle at which the transmitter sees the receiver),
We can clearly see from Eq. 3 that once OWPT happens,
In practice,
3.4 Affecting Factors to System Efficiency
First, system efficiency (whether QE or PCE) is affected by the alignment between the light transmitter and the light receiver, where any misalignment can make system efficiency decrease. Note that this happens to not only OWPT systems but also other WPT systems such as IWPT or CWPT. However, in such scenarios for OWPT systems, wireless energy transmitters based on LEDs are better than those based on lasers because OWPT might still occur using LEDs, thanks to their spread light beams, while it does not happen with lasers having focused and narrow light beams. To overcome the drawback of misalignment in WPT systems, additional devices are needed to help the energy transmitter and receiver track and align with each other. Nonetheless, this will certainly increase the system complexity, size, weight, and cost.
To quantitatively verify how the alignment between perovskite solar cells affects the overall system efficiency, additional experiments need to be conducted. Again, as discussed before, currently available perovskite LEDs are not durable enough for doing many experiments; hence, we hope to be able to report such results in the near future.
Next, it is well known that the light beams of LEDs are spread, unlike those of lasers, which reduces the overall system efficiency since less energy can reach the light receiver when traveling through the transmission range. Recent progresses in perovskite laser research [see, e.g., reviews in Quan et al. (2019); Dong et al. (2020); Gunnarsson and Rand (2020), and our recent work by Qin et al. (2020)] suggest that electrically driven lasers based on a metal halide perovskite emitter are promising and hence can be employed for our proposed perovskite-transceiver–based bidirectional OWPT system to increase the overall system efficiency. Nevertheless, there are also disadvantages of lasers compared to LEDs; one is the unsuccessful WPT if misalignment between the optical transmitter and receiver exists, as noted above, and the other is the safety concern of lasers. Another solution to increase the system efficiency without using lasers is to utilize supplemented devices to spread the LED light beams less (see, e.g., Song et al., 2018), but such devices will certainly make the OWPT system more complicated and costly.
Lastly, energy losses, caused by load resistance in the wireless energy-receiving devices, can reduce system efficiency if the load resistance is considerably high. However, this not only happens to our proposed OWPT systems but also occurs in other WPT systems. Details on this issue should be investigated in a separate study; hence, we leave it as future work.
4 Conclusion and Discussion
4.1 Summary
This article has proposed a novel conceptual OWPT system between objects equipped with optical transceivers made from metal halide perovskite devices. Each of such objects can work as both a light absorber and a light emitter, leading to bidirectional OWPT between those objects, whether they are stationary or moving. Theoretical characterization of the overall system energy conversion efficiency has been provided, and experimental results on the light absorption and emission modes of perovskite devices have been reported.
Based on the experimental results and those reported in the literature, the QE of the proposed perovskite-transceiver–based OWPT system is now maximally 9.94% at the 790 nm light wavelength (peak EL intensity). The PCE of the proposed system is anticipated to be much lower than that of the resonant IWPT system. However, the payoffs of rather simple electronic requirement, small size and weight, potentially low cost, usability for curved surfaces, printability, and full support for moving objects render the proposed OWPT system appropriate for ubiquitous applications, especially in the current Internet-of-things (IoT) era. Those applications include not only the wireless energy transfer between devices equipped with perovskite transceivers but also the harvest of solar energy and energy from other lighting resources. Hence, this perovskite-based OWPT system provides a sustainable and green energy technology approach to the society.
In addition, the proposed conceptual system contributes the first step toward realization of a T2T-OWPT network, in which OWPT can be made anywhere and anytime between diverse entities (things), e.g., consumer electronic devices, implant medical devices, robots, and IoT devices. Such T2T-OWPT network will bring not only the ultra-mobility of energy but also the convenience and comfort for humans.
4.2 Further Discussions
The durability of perovskite LEDs is an important issue needed to be improved in the near future in order to make them available for commercialization as well as for reliable serving in the proposed OWPT system. Furthermore, additional devices such as the optical reflectors and concentrators can be employed to increase the overall system energy conversion efficiency.
In the area of vehicle electrification, our proposed OWPT system could impinge upon novel wireless charging and discharging methods for electrified vehicles covered with perovskite solar cells. It is worth mentioning that solar-cell-covered vehicles have recently been initiated by several automobile companies, e.g., Hyundai (2020); NEDO-Sharp-Toyota (2020); Sion (2020).
It is also worth noting that the laser can be used in the proposed perovskite-transceiver–based bidirectional OWPT system, in which the laser beam is generated in the light-emitting mode instead of LED light. Due to the coherence of laser beams, higher overall system energy conversion efficiency and longer WPT range could be achieved. Although the laser beam is harmful to humans if the energy level is high, as discussed before, this kind of system could be useful in specific applications where humans and other species are not present and energy grids do not exist or are not easily implemented, e.g., in space exploration missions (for instance, the Moon or Mars exploration) or in maritime applications. In such situations, other WPT technologies such as IWPT or CWPT are hard to be deployed, while with the proposed bidirectional perovskite-transceiver–based OWPT systems, solar energy can be harvested by spacecraft, satellites, landers, rovers, maritime vehicles and equipment, etc., and then is wirelessly transmitted to the others when they lack energy but cannot receive it from solar. As a result, the mission period can be extended smoothly, which is very important for such applications. Therefore, the proposed OWPT systems provide a viable solution despite some limitations (e.g., low efficiency, direct line-of-sight) as commonly found in other applications using solar cells.
Finally, light exposure safety would be of concern under high light intensity (e.g., when using the laser beam), or under high energy levels. For extensive information related to such issues, existing standards and guidelines are useful, see, e.g., ICNIRP (2020); Renesas (2020).
Data Availability Statement
The original contributions presented in the study are included in the article/Supplementary Material, and further inquiries can be directed to the corresponding author.
Author Contributions
DN conceptualized the idea, performed the methodology, and wrote the original draft. CQ and TM performed the experiments and reviewed and edited the paper. CA supervised the work and also took part in reviewing the paper.
Funding
The first author’s research was partially funded by JSPS Kakenhi Grant Number JP19K15013. Additionally, this research was supported by the Japan Science and Technology Agency (JST), ERATO, Adachi Molecular Exciton Engineering Project (JST ERATO Grant Number JPMJER1305); JSPS KAKENHI (grant numbers JP16H04192 and JP20H02817); The Canon Foundation; and The Samco Foundation.
Conflict of Interest
The authors declare that the research was conducted in the absence of any commercial or financial relationships that could be construed as a potential conflict of interest.
Abbreviations
CWPT, capacitive wireless power transfer; EL, electroluminescence; EQE, external quantum efficiency; EV, electric vehicle; IPCE, incident photon-to-electron conversion efficiency; IWPT, inductive wireless power transfer; LED, light-emitting diode; NIR, near-infrared; OWPT, optical wireless power transfer; PCE, power conversion efficiency; PL, photoluminescence; QE, quantum efficiency; T2T, thing-to-thing; WPT, wireless power transfer.
References
Caltech, U. (2020). Space Solar Power Project. [retrieved 15 October 2020], Available at: https://www.spacesolar.caltech.edu.
Cao, Y., Wang, N., Tian, H., Guo, J., Wei, Y., Chen, H., et al. (2018). Perovskite Light-Emitting Diodes Based on Spontaneously Formed Submicrometre-Scale Structures. Nature 562, 249–253. doi:10.1038/s41586-018-0576-2
Charge, W. (2020). [retrieved 15 October 2020], Available at: https://wi-charge.com.
Dong, H., Zhang, C., Liu, X., Yao, J., and Zhao, Y. S. (2020). Materials Chemistry and Engineering in Metal Halide Perovskite Lasers. Chem. Soc. Rev. 49 (3), 951–982. doi:10.1039/c9cs00598f
Fakidis, J., Videv, S., Kucera, S., Claussen, H., and Haas, H. (2016). Indoor Optical Wireless Power Transfer to Small Cells at Nighttime. J. Lightwave Technol. 34 (13), 3236–3258. doi:10.1109/jlt.2016.2555883
FISE-Germany (2020). Science blog [retrieved 15 October 2020], Available at: https://blog.innovation4e.de/en/2016/09/12/power-by-light.
Gets, D., Alahbakhshi, M., Mishra, A., Haroldson, R., Papadimitratos, A., Ishteev, A., et al. (2021). Reconfigurable Perovskite Lec: Effects of Ionic Additives and Dual Function Devices. Adv. Opt. Mater. 9, 2001715. doi:10.1002/adom.202001715
Gets, D., Saranin, D., Ishteev, A., Haroldson, R., Danilovskiy, E., Makarov, S., et al. (2019). Light-emitting Perovskite Solar Cell with Segregation Enhanced Self Doping. Appl. Surf. Sci. 476, 486–492. doi:10.1016/j.apsusc.2019.01.031
Gunnarsson, W. B., and Rand, B. P. (2020). Electrically Driven Lasing in Metal Halide Perovskites: Challenges and Outlook. APL Mater. 8, 030902. doi:10.1063/1.5143265
Hata, K., Hanajiri, K., Imura, T., Fujimoto, H., Hori, Y., Sato, M., et al. (2018). Driving Test Evaluation of Sensorless Vehicle Detection Method for In-Motion Wireless Power Transfer, In The 2018 International Power Electronics Conference, 1–4.
Hirukawa, H., Yamaguchi, T., Ushida, Y., Onuma, T., and Honda, T. (2019). Prototype Optical Wireless Power Transmission System Using Blue Ld as Light Source and Led as Photovoltaic Receiver, In The 1st Optical Wireless and Fiber Power Transmission Conference. Yokohama, Japan: OWPT.
Hyundai (2020). [retrieved 15 October 2020], Available at: https://www.hyundai.news/eu/brand/hyundai-launches-first-car-with-solar-roof-charging-system.
ICNIRP (2020). Icnirp Guidelines on Limits of Exposure to Incoherent Visible and Infrared Radiation. [retrieved 15 October 2020], Available at: https://www.icnirp.org/cms/upload/publications/ICNIRPVisible_Infrared2013.pdf.
JAXA-Japan (2020). Research on Laser Wireless Power Transmission Technology. [retrieved 15 October 2020], Available at: http://www.kenkai.jaxa.jp/eng/research/ssps/ssps-lssps.html.
Jin, K., and Zhou, W. (2019). Wireless Laser Power Transmission: A Review of Recent Progress. IEEE Trans. Power Elect. 34 (4), 3482–3499. doi:10.1109/tpel.2018.2853156
Jin, M. H. C., Pierce, J. M., Lambiotte, J. C., Fite, J. D., Marshall, J. S., and Huntley, M. A. (2018). Underwater Free-Space Optical Power Transfer: An Enabling Technology for Remote Underwater Intervention. Offshore Tech. Conf. 1–9. doi:10.4043/28892-MS
Katsuta, Y., and Miyamoto, T. (2018). Design and Experimental Characterization of Optical Wireless Power Transmission Using GaAs Solar Cell and Series-Connected High-Power Vertical Cavity Surface Emitting Laser Array. Jpn. J. Appl. Phys. 57 (08PD01). doi:10.7567/jjap.57.08pd01
Kojima, A., Teshima, K., Shirai, Y., and Miyasaka, T. (2009). Organometal Halide Perovskites as Visible-Light Sensitizers for Photovoltaic Cells. J. Am. Chem. Soc. 131 (17), 6050–6051. doi:10.1021/ja809598r
Komine, T., and Nakagawa, M. (2004). Fundamental Analysis for Visible-Light Communication System Using LED Lights. IEEE Trans. Consumer Electron. 50 (1), 100–107. doi:10.1109/tce.2004.1277847
Li, S., and Mi, C. C. (2015). Wireless Power Transfer for Electric Vehicle Applications. IEEE J. Emerging Selected Topic Power Elect. 3 (1), 4–17.
Lin, K., Xing, J., Quan, L. N., de Arquer, F. P. G., Gong, X., Lu, J., et al. (2018). Perovskite Light-Emitting Diodes with External Quantum Efficiency Exceeding 20 Per Cent. Nature 562, 245–248. doi:10.1038/s41586-018-0575-3
Lu, M., Bagheri, M., James, A. P., and Phung, T. (2018). Wireless Charging Techniques for Uavs: A Review, Reconceptualization, and Extension. IEEE Access 6, 29865–29884. doi:10.1109/access.2018.2841376
Machura, P., and Li, Q. (2019). A Critical Review on Wireless Charging for Electric Vehicles. Renew. Sust. Energ. Rev. 104, 209–234. doi:10.1016/j.rser.2019.01.027
Miao, Y., Ke, Y., Wang, N., Zou, W., Xu, M., Cao, Y., et al. (2019). Stable and Bright Formamidinium-Based Perovskite Light-Emitting Diodes with High Energy Conversion Efficiency. Nat. Commun. 10, 3624. doi:10.1038/s41467-019-11567-1
Mostafa, T. M., Muharam, A., and Hattori, R. (2017). Wireless Battery Charging System for Drones via Capacitive Power Transfer, In 2017 IEEE PELS Workshop on Emerging Technologies: Wireless Power Transfer, 1–6.
NASA-USA (2020a). 2020 nasa technology taxonomy [retrieved 15 October 2020], Available at: https://www.nasa.gov/sites/default/files/atoms/files/2020_nasa_technology_taxonomy_lowres.pdf.
NASA-USA (2020b). 6 Things to Know about Nasa’s Ingenuity mars Helicopter. [retrieved 6 February 2021], Available at: https://www.nasa.gov/feature/jpl/6-things-to-know-about-nasas-ingenuity-mars-helicopter.
National Renewable Energy Laboratory-USA (2020). Best Research-Cell Efficiencies. [retrieved 15 October 2020], Available at: https://www.nrel.gov/pv/assets/pdfs/best-research-cell-efficiencies.20200925.pdf.
NEDO-Sharp-Toyota (2020). [retrieved 15 October 2020], Available at: https://global.toyota/en/newsroom/corporate/28787347.html.
Nguyen, D. H. (2020). Optical Wireless Power Transfer for Moving Objects as a Life-Support Technology, In The 2020 IEEE 2nd Global Conference on Life Sciences and Technologies. Kyoto, Japan LifeTech, 405–408.
Patil, D., Mcdonough, M. K., Miller, J. M., Fahimi, B., and Balsara, P. T. (2018). Wireless Power Transfer for Vehicular Applications: Overview and Challenges. IEEE Trans. Transp. Electrific. 4 (1), 3–37. doi:10.1109/tte.2017.2780627
Qin, C., Matsushima, T., Fujihara, T., and Adachi, C. (2017). Multifunctional Benzoquinone Additive for Efficient and Stable Planar Perovskite Solar Cells. Adv. Mater. 29 (4), 1603808. doi:10.1002/adma.201603808
Qin, C., Matsushima, T., Fujihara, T. W. J. P., Potscavage, W. J., and Adachi, C. (2016). Degradation Mechanisms of Solution-Processed Planar Perovskite Solar Cells: Thermally Stimulated Current Measurement for Analysis of Carrier Traps. Adv. Mater. 28 (3), 466–471. doi:10.1002/adma.201502610
Qin, C., Matsushima, T., Klotz, D., Fujihara, T., and Adachi, C. (2019). Device Stability: The Relation of Phase-Transition Effects and Thermal Stability of Planar Perovskite Solar Cells (Adv. Sci. 1/2019). Adv. Sci. 6 (1), 1970004. doi:10.1002/advs.201970004
Qin, C., Sandanayaka, A. S. D., Zhao, C., Matsushima, T., Zhang, D., Fujihara, T., et al. (2020). Stable Room-Temperature Continuous-Wave Lasing in Quasi-2d Perovskite Films. Nature 585, 53–57. doi:10.1038/s41586-020-2621-1
Quan, L. N., Rand, B. P., Friend, R. H., Mhaisalkar, S. G., Lee, T.-W., and Sargent, E. H. (2019). Perovskites for Next-Generation Optical Sources. Chem. Rev. 119 (12), 7444–7477. doi:10.1021/acs.chemrev.9b00107
Rau, U. (2007). Reciprocity Relation between Photovoltaic Quantum Efficiency and Electroluminescent Emission of Solar Cells. Phys. Rev. B. 76, 085303. doi:10.1103/physrevb.76.085303
Renesas, (2020). Eye Safety for Proximity Sensing Using Infrared Light-Emitting Diodes. (version 3.00, apr. 28, 2016) [retrieved 15 October 2020]. https://www.renesas.com/jp/ja/doc/application-note/an1737.pdf.
Šcajev, P., Aleksieju̅nas, R., Baronas, P., Litvinas, D., Kolenda, M., Qin, C., et al. (2019). Carrier Recombination and Diffusion in Wet-Cast Tin Iodide Perovskite Layers under High Intensity Photoexcitation. The J. Phys. Chem. C 123 (32), 19275–19281.
Sion, S. (2020). [retrieved 15 October 2020], Available at: https://sonomotors.com/en/sion. doi:10.1145/3372297.3416242
Song, J., Kim, K. H., Kim, E., Moon, C. K., Kim, Y. H., Kim, J. J., et al. (2018). Lensfree Oleds with over 50% External Quantum Efficiency via External Scattering and Horizontally Oriented Emitters. Nat. Commun. 9, 3207. doi:10.1038/s41467-018-05671-x
Tumen-Ulzii, G., Matsushima, T., Klotz, D., Leyden, M. R., Wang, P., Qin, C., et al. (2020). Hysteresis-less and Stable Perovskite Solar Cells with a Self-Assembled Monolayer. Commun. Mater. 1 (31). doi:10.1038/s43246-020-0028-z
Tumen-Ulzii, G., Qin, C., and Adachi, C. (2020). Detrimental Effect of Unreacted Pbi2 on the Long-Term Stability of Perovskite Solar Cells. Adv. Mater. 32 (16), 1905035.
Xu, W., Hu, Q., Bai, S., Bao, C., Miao, Y., Yuan, Z., et al. (2019). Rational Molecular Passivation for High-Performance Perovskite Light-Emitting Diodes. Nat. Photon. 13, 418–424. doi:10.1038/s41566-019-0390-x
Yoshida, S., Tanomura, M., Hama, Y., Hirose, T., Suzuki, A., Matsui, Y., et al. (2016). Underwater Wireless Power Transfer for Non-fixed Unmanned Underwater Vehicle in the Ocean. In 2016 IEEE/OES Autonomous Underwater Vehicles (AUV). 177–180.
Appendix
Detailed Fabrication Conditions of MA0.6 FA0.4 PBI2.8 BR0.2 Perovskite Solar Cells and Films
Glass substrates coated with a prepatterned ITO layer with a thickness of ≈150nm (ATSUGI MICRO) or fused silica substrates were cleaned by ultrasonication in detergent, pure water, acetone, and isopropanol and then subjected to UV-ozone treatment.
For the perovskite film fabrication, PbI2, PbBr2, MAI, and FAI were dissolved in a mixture of
For the perovskite solar cell fabrication, a thin layer (≈50nm) of PEDOT:PSS (Clevios, Al4083) was prepared by spin-coating at 3,000 rpm for 45 s on top of ITO in air, followed by baking the PEDOT:PSS layer at 160°C for 10 min. The perovskite layer was prepared in a nitrogen-filled glove box in the same way described earlier. Finally, 30 nm C60, 10 nm BCP, and 100 nm Au layers were thermally deposited on top of the perovskite layer under a high vacuum (10-4 Pa). The completed solar cells were encapsulated using a glass lid and UV-cured sealant, without exposure to air at all. Current density–voltage and IPCE measurements were performed on the PSCs using a computer-controlled Keithley 2400 source unit and an EQE measurement system (WXS-155S-10: Wacom Denso) under simulated AM1.5G solar illumination from an Xe lamp–based solar simulator (SRO-25 GD, Bunkokeiki). The active area of the solar cells was 16 mm2. The lamp power was carefully calibrated at 100 mW cm-2 (1 Sun) using a crystalline Si reference cell with an amorphous Si optical filter (Bunkokeiki), which was certified by the National Institute of Advanced Industrial Science and Technology of Japan. LED properties of solar cells were measured using an integrating sphere system (A10094, Hamamatsu Photonics) equipped with a photonic multichannel analyzer (C9920-12, Hamamatsu Photonics) and a computer-controlled source meter (2400, Keithley).
Keywords: optical wireless power transfer, optical transceiver, metal halide perovskite, bidirectional wireless power transfer, wireless charging
Citation: Nguyen DH, Matsushima T, Qin C and Adachi C (2021) Toward Thing-to-Thing Optical Wireless Power Transfer: Metal Halide Perovskite Transceiver as an Enabler. Front. Energy Res. 9:679125. doi: 10.3389/fenrg.2021.679125
Received: 11 March 2021; Accepted: 18 May 2021;
Published: 17 June 2021.
Edited by:
Farhad Taghizadeh-Hesary, Tokai University, JapanCopyright © 2021 Nguyen, Matsushima, Qin and Adachi. This is an open-access article distributed under the terms of the Creative Commons Attribution License (CC BY). The use, distribution or reproduction in other forums is permitted, provided the original author(s) and the copyright owner(s) are credited and that the original publication in this journal is cited, in accordance with accepted academic practice. No use, distribution or reproduction is permitted which does not comply with these terms.
*Correspondence: Dinh Hoa Nguyen, aG9hLm5kQGkyY25lci5reXVzaHUtdS5hYy5qcA==