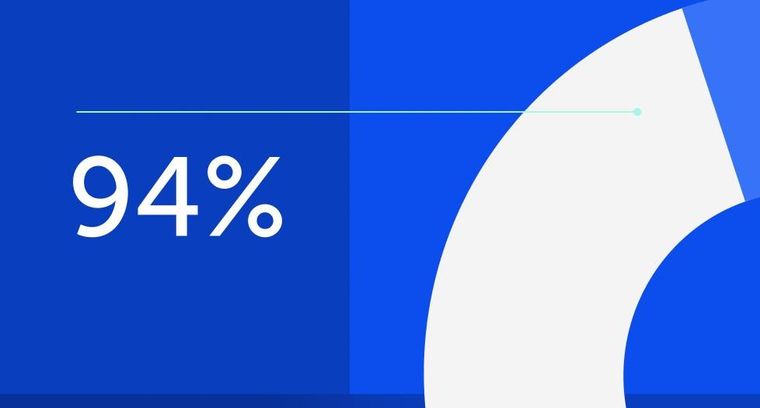
94% of researchers rate our articles as excellent or good
Learn more about the work of our research integrity team to safeguard the quality of each article we publish.
Find out more
REVIEW article
Front. Energy Res., 10 March 2021
Sec. Sustainable Energy Systems
Volume 9 - 2021 | https://doi.org/10.3389/fenrg.2021.638105
This article is part of the Research TopicUpscaling Low-Carbon Energy Resources: Exploring the Material Supply Risk, Environmental Impacts and Response PoliciesView all 10 articles
In response to climate change and energy transition, natural gas has been rapidly developed as a relatively low-carbon energy source by many countries. However, there remain environmental risks at different stages in the entire process from exploitation to utilization. Firstly, this article identifies various environmental risks and benefits of natural gas along the entire industry chain from upstream exploitation and midstream transportation to downstream utilization. It is found that, during upstream exploitation, hydraulic fracturing has the worst environmental impact. During the midstream storage and transportation stage, methane leakage is the biggest environmental risk. In the downstream combustion and utilization stage, the risk to environment is less than other energy sources, although there are some greenhouse gas effects and water pollution issues. Thus, this article puts forward some policy recommendations for different stages from exploitation to utilization. In the upstream stage, especially hydraulic fracturing activity, we suggest strengthening environmental assessment management, improving policy standards, creating a water quality monitoring plan, and promoting the innovation of key technologies. In terms of the midstream, besides pipeline laying and site selection, we focus on monitoring the system, including leak detection, quality management of engineering materials, and risk identification and management. When it comes to the downstream, we encourage the application of advanced technologies to improve thermal efficiency and reduce emissions, such as gas-fired related technologies, natural gas recycling technologies, distributed energy technologies, and green and low-carbon service technologies.
As a result of actual or predicted effects of climate change and the ongoing energy transition, natural gas has become a favored energy source, due to its relatively clean combustion, during the transition from fossil fuels to fully renewable energy by the governments of various countries. As a result, natural gas, rich in reserves, is the cleanest and fastest growing fossil fuel and accounts for nearly one-third of global energy demand growth and nearly one-quarter of power generation (World Energy Agency, 2020). Data from the Organization of Petroleum Exporting Countries (OPEC) show that the world's proven natural gas reserves were 188 trillion cubic meters (OPEC, 2019). According to BP’s World Energy Statistics Review 2020 (BP, 2020), the global average annual growth rate in natural gas consumption was 2.6% over the past ten years. In 2019, global natural gas consumption increased by 78 billion cubic meters, a year-on-year increase of 2%. Its growth rate is faster than that of oil (0.3% per year) and coal (average −0.1% per year). It is estimated that, by 2040, natural gas usage will surpass coal and approach oil (when converted to tons of oil equivalent) and will be the only fossil fuel energy source competing against renewable energy. Natural gas is significantly cleaner and has less greenhouse effect than coal and oil energy sources, based on carbon dioxide emission in the combustion and utilization stages. According to the United States Energy Information Administration (EIA) estimates, the carbon dioxide emissions of one million Btu of calorific value produced by natural gas combustion are 53.97 kg, whereas the combustion of various types of coal emits 95.35 kg of carbon dioxide on average, gasoline emits 71.30 kg, and petroleum coke emits 102.1 kg (EIA United States Energy Information Administration, 2018). Although the carbon dioxide emissions of natural gas in the combustion and utilization stage are much lower than other fossil energy sources, natural gas still has certain environmental risks in other stages of the industrial chain from exploration and production to utilization (Kondash et al., 2017). Therefore, it is necessary to conduct a comprehensive assessment of these environmental risks, given the expanding consumption of natural gas.
Natural gas is a fossil fuel mainly composed of methane, which is usually divided into conventional and unconventional natural gas. Conventional natural gas is found either as just a gas phase or as a gas cap over an oil reservoir. Unconventional gas reservoirs are mainly shale, tight sandstone, coal, and hydrate. Different reservoir types need different technologies for recovery of gas, and different technologies bring different environment risks. Depending on the transportation mechanism, natural gas can be divided into pipeline, compressed, and liquefied natural gas.
Many studies have focused on the environmental risks along the natural gas industrial chain. Published research mainly focuses on water resources and greenhouse gases. For example, a number of studies have conducted extensive research on water pollution caused by hydraulic fracturing activities during natural gas development. Gagnon et al. (2016) reviewed the literature on the impacts of hydraulic fracturing on water quality and focused on regulatory frameworks. Rodriguez et al. (2020) evaluated the impact of hydraulic fracturing on groundwater quality in the Permian Basin, West Texas. Yazdan et al. (2020) conducted a review of the impacts of wastewater disposal in the hydraulic fracturing industry in the United States. Some studies conducted research on the amount of greenhouse gas emissions in natural gas development. For example, Crow et al. (2019) assessed the potential impact of greenhouse gas emissions from natural gas production by combining the estimates of
Secondly, the published research has been mostly aimed at a given stage of the chain. Studies have focused on the environmental risks from upstream exploitation and production of natural gas. For example, Kondash et al. (2017), Gallegos et al. (2015), and Thacker et al. (2015) studied water treatment issues related to unconventional oil, gas exploration, and hydraulic fracturing activities in the upstream stage. Wang et al. (2020) focused on short-term mechanism coupling shear stress and hydraulic fracturing in an experimental simulation triggered by hydraulic fracturing and found that short-term hydraulic fracturing was less likely to cause rock mass instability or earthquake, but the long-term fluid injection could increase the pore pressure and change the in situ stress field in a large area to induce an earthquake. Mohan et al. (2013) and Brittingham et al. (2014) studied the ecological risks of natural gas development to wildlife, aquatic resources, and their habitats. Some studies have also conducted studies on other aspects of the natural gas industry. For example, Ou and Yuan (2019) conducted a life cycle analysis of greenhouse gas emissions on liquefied natural gas (LNG) and compressed natural gas (CNG) in heavy-duty trucks. Yuan et al. (2019) estimated the venting and fugitive leaks from natural gas supply chains and found that promoting natural gas vehicles is effective at helping to reduce greenhouse gas emissions from road transportation. However, there is still a lack of integrated analysis of the potential risks of natural gas utilization over the entire industry chain.
The purpose of this article is therefore to systematically analyze various environmental risks at different stages of the entire natural gas industry chain and recommend regulatory policies to deal with these environmental risks. The novel aspects of this article are mainly twofold: one is to cover the most environmental risks related to natural gas all around the world, not just the water pollution and greenhouse gas emissions that current scholars are mostly concerned about in one country; the other is to cover the entire natural gas industry chain, rather than just focusing on the environmental risks at a specific stage.
This article presents a systematic review of the environmental risks from exploitation of natural gas resources along the industry chain. Generally, the entire industry chain of natural gas can be divided into three major stages: upstream, midstream, and downstream. The upstream industry is mainly engaged in the exploration for and development of different types of gas resources, such as conventional natural gas, coalbed methane, shale gas, and other tight gas. The midstream industry mainly provides natural gas transportation, storage, vaporization, and liquefaction services, including pipeline network transportation, gas storage facilities, gasification, and tank truck transportation. Downstream industries mainly involve the delivery of natural gas to the end user for different purposes, including domestic gas, industrial fuel, natural gas power generation, natural gas chemical industry, and different types of transportation. Since different technologies, equipment, and processes are used in different stages, the potential environmental risks are also different; then, we recognize the main points of environmental risks in the following (see Figure 1).
In the upstream extraction stage of natural gas, the main types of activities carried out include seismic exploration, drilling, workover, gas field gathering and on-site transportation, and natural gas purification (Dong et al., 2003). Conventional natural gas mostly exists in highly porous and permeable reservoirs, and standard vertical wells are easy to produce (see Figure 2). However, unconventional natural gas is mostly found in low-permeability rocks, and it is relatively difficult to produce. It is often necessary to develop and adopt novel technologies, such as horizontal drilling and formation stimulation. Hydraulic fracturing is currently the commonly used formation stimulation technology for gas in rocks with low porosity and permeability. In hydraulic fracturing operations, the fracturing fluid is pumped carefully under controlled high pressure, and the sand mixed with the fracturing fluid flows in to support the fractures generated. Afterward, sealing wells is necessary to prevent natural gas, fracturing fluids, chemicals, and produced water from leaking into the groundwater supply (Union of Concerned Scientists, 2020). Therefore, the entire upstream process may cause air, water, light, and noise pollution; affect land use, wildlife activities, and water use; and even induce earthquakes (source: adopted from United States Geological Survey Factsheet 0113–01 (public domain)).
The air pollutants produced during natural gas extraction mainly include construction dust, fuel exhaust gas, blast burning exhaust gas, leakage of methane, and pipeline cleaning exhaust gas (Yuan and Yang, 2019). Many studies show that the concentration of these harmful air pollutants increase in some areas during the drilling and production process (CEPAARB, 2012). Adverse health conditions can arise in some people when exposed to high levels of air pollutants, including respiratory symptoms, cardiovascular disease, and cancer (EPA Environmental Protection Agency, 2013b). Furthermore, the extent of the pollution and its impact on the environment are related to the distance from the development zone. It has been found that residents who lived within half a mile radius from gas wells were affected more severely than those who lived further away (McKenzie et al., 2012).
The main component of natural gas is methane (CH4), whose content is generally greater than 60% (in most cases it is more than 99%), and highly variable amounts of C2H6, C3H8, C4H10, CO2, N2, H2S, and trace inert gases (Milkov et al., 2005). Therefore, methane emission caused by natural gas leakage is usually the most abundant pollutant. According to estimates by the United States Environmental Protection Agency in 2017, natural gas, other petroleum systems, and abandoned gas wells accounted for approximately 32% of total United States methane emissions and approximately 4% of total United States greenhouse gas emissions (EPA, 2017). Greenhouse gas refers to any gas that absorbs and releases infrared radiation and exists in the atmosphere and mainly includes CO2, CH4, N2O, HFCS, PFCS, and SF6 (Breidenich et al., 1998). Untreated emitted methane will have some impact on global warming. It is known that the greenhouse effect of every cubic meter of methane leakage is nine times greater than the products of methane combustion (IPCC Intergovernmental Panel on Climate Change, 2007). However, there is still some controversy about the rate of methane leakage. Allen et al. (2013) systematically collected information on methane leakage from shale gas production in the United States, and they found that, under good conditions, methane emissions accounted for about 0.5% of the natural gas extracted. McCabe et al. (2015) believed that the leakage reported from the entire natural gas system ignored the emissions generated during well completion and production. The quantification of methane leakage is still an ongoing issue (Brandt et al., 2014). Although the impact of methane leakage is large, some technological improvements can greatly help reduce methane leakage. For example, green completion technology can reduce methane emissions by 90% during drilling activities and the total wellhead emissions by 81% (Mackay and Stone, 2013). Taking this a step further, the installation of leak-proof components in a well can help the natural gas sector reduce methane emissions by 31–44 MtCO2e every year in China (Brink et al., 2013).
Besides methane, there is still a small volume of other emissions from natural gas production. VOC, PM, and SO2 emissions in production are less than 1% of that in industrial emissions, and NOx emissions account for 2.9–4.8% of that in industrial emissions (Litovitz et al., 2013), but even this small-scale pollution discharge will have an impact on local or regional air quality. The concentration of harmful air pollutants increases in some areas during the drilling process, and although this impact may be short-term, it can still cause harmful effects on health and environment (Witherspoon, 2012).
In summary, in this stage, natural gas emissions are relatively small, but, limited by the current technology, there is a certain risk of leakage. The main outcome from natural gas leakage (methane) is to increase greenhouse gas emission, which will contribute to global warming, while a small volume of other gases is harmful to human health. Therefore, the key to the problem is to improve technology to reduce the risk of gas leakage.
The environmental impacts caused by the large-scale production of natural gas mainly include occupation of land resources, destruction of surface landforms and vegetation, change in surface runoff, and increase in soil erosion. It may also affect wildlife habitat, biodiversity, and local ecosystem balance in general (Yuan and Yang, 2019). During the drilling process, construction and land disturbance can change land use, damage or destroy local ecosystems, cause erosion, and destroy wildlife habitats and migration patterns. The hydraulic fracturing process will directly occupy the land, destroy animal habitats, and cause the fragmentation of the ecosystem. Well sites and dirt roads will also increase soil erosion and affect the flow and properties of rivers (Granoff et al., 2015). Taking coal-bed methane (CBM) as an example, a single CBM well covers an area of about 1,000–3,500 m2 during the construction period and a permanent area of about 600–25,002 during the mining period (Huang et al., 2018). Chen et al. (2017) studied the potential impact of flowback water from hydraulic fracturing on agricultural soil quality and found that soil enzyme activities were sensitive to the composition of flowback water. Mohan et al. (2013) compared the microbial ecology of prefracture water (fracturing raw water and fracturing fluid) and produced water at multiple time points in a natural gas well in southwestern Pennsylvania and found that the biodiversity is gradually declining.
In summary, the construction process will cause serious ecological damage to land, vegetation, and streams, which will affect the ecological environment of animals and plants, and affect the development of biodiversity. Especially for hydraulic fracturing activities, the fracturing fluid will directly affect the structure of the microbial community and break the original ecological balance. Thus, it is very necessary to reduce the frequency of human activities in the production process.
Water consumption for unconventional natural gas development is relatively larger than that of conventional natural gas (EPA Environmental Protection Agency, 2013a). The growth of hydraulic fracturing and its use of large amounts of water per well may strain local ground and surface water supplies, especially in water-scarce areas. Water consumption for hydraulic fracturing may vary depending on the local geology, as well as construction and hydraulic fracturing processes (Gagnon et al., 2016). Unlike other energy-related water extraction methods, where water usually returns to rivers and lakes, most of the water used for unconventional natural gas development is not recyclable. When a well continues to operate or is additionally fractured later in its life cycle to maintain well pressure and natural gas production, an additional large volume of water is required (Breitling Oil and Gas, 2012). At the same time, gas gathering stations, living areas, and site greening also require water resources. The more complex the reservoir conditions are and the greater the burial depth is, the more difficult it is to increase production and the greater the volume of water resources used becomes (Yuan and Yang, 2019).
Water pollution is mainly caused by gas pollutants including methane and volatile organic compounds, drilling fluid, fracturing fluid pollutants, equipment leakage, and improper handling during development (Shonkoff et al., 2014). The drilling fluid and hydraulic fracturing fluid contains a lot of chemical components, including acids (especially hydrochloric acid), fungicides, scale removers, and friction reducers (Wang et al., 2014). If the drilling and other activities are not handled properly and under inadequate supervision, it may cause fracturing fluid leakage in the vertical well section (Mordick, 2014). In other laterals, fracturing fluid can also enter groundwater along abandoned wells, improperly sealed and improperly constructed wells, and induced cracks or cesspits, and this can affect local water quality (Vidic et al., 2013). Many companies in the United States, China, and other places use wastewater recharge in the shale gas industry (wastewater recharge rate is close to 100%), and this wastewater recharge method will disrupt the water recycling and cause aquifer pollution (Freyman, 2014).
Pollution of drinking water sources will affect biological health and bring about a series of ecological and environmental impacts (Rahm and Riha, 2014). Depending on the geological structure of the rock formation, the fracturing fluid contains 20%–80% of the flowback wastewater within a few weeks after fracturing (Zammerilli et al., 2014). The harmful substances in wastewater can be in high enough concentrations to not only kill microorganisms but also induce negative effects on human health if consumed (Colborn et al., 2011).
In summary, in the process of natural gas extraction, the activities need consume large water and cause water pollution to some extent. Due to the fracturing activities’ need of adding some extra chemical substance, the improper dealing of technology and management would bring great water pollution. If drinking water is corroded, it will also affect microorganisms and human health. Thus, improving the technology of gas extraction, especially that of fracturing activities, is the key point to protect water resources.
The recharge of wastewater during hydraulic fracturing will put pressure on geological faults and may induce earthquakes. High-pressure injection to dispose hydraulic fracturing wastewater can cause major earthquakes in the United States (National Research Council, 2013). Van der Elst et al. (2013) found that at least half of the 4.5 or larger earthquakes on the Richter scale that hit the United States mainland in the past decade occurred in areas with potential injection-induced seismic activity. Amini and Eberhardt (2019) investigated the influence of the tectonic stress regime on the magnitude of induced seismicity related to hydraulic fracturing practices through a series of numerical simulations and found that thrust faulting stress regimes are more susceptible to larger induced seismicity than strike-slip stress regimes. The latest research showed that short-term hydraulic fracturing was less likely to cause rock mass instability or earthquakes, but the long-term fluid injection could increase the pore pressure and change the in situ stress field in a large area, thereby inducing an earthquake (Wang et al., 2020). Furthermore, Villa and Singh (2020) reached the same conclusion through a detailed analysis of 17 major hydraulic fracturing sites in the United States. They found that the incidence of earthquakes depends on the amount of water injected from horizontal injection wells; disposal injection wells; and the geological, hydrological, and geophysical environment near the drilling site. Burton and Nadelhoffer (2013) also showed that the effects of hydraulic fracturing could increase erosion and subsidence. If free methane and other gases reach a sufficient volume, they may cause an explosion and bring about a minor earth tremor (Airgas, 2013). According to Stark et al. (2014), frequent construction activities and bright lights at night bring intensive noise and light pollution, which directly affect the local environment and its ecosystem and affect the daily lives of local residents (Brittingham et al., 2014). Li et al. (2019) analyzed the effects of different gas wells exploitation on the concentrations of heavy metals in the soil and found that although the exploitation of shale gas mining had no obvious influence on the concentrations of heavy metals in the soil around the well field, the wells pose potential heavy metal pollution because of the high content of Cd in the soil before exploitation.
In summary, these frequent human activities directly affect the local environment, and the hydraulic fracturing activity even has deep, albeit mostly minor, geological impact.
Through this literature review, we found that in the upstream phase, the impact on land use, wildlife activities, and water use can be great due to the frequent human construction activity. Natural gas emissions are relatively small in the production process, but there is a certain risk of leakage. Gas leakage will increase the greenhouse effect. Work on quantification of methane leakage is still ongoing, and there is no consensus at present. However, there is no doubt that improvements in technology to reduce the risk of gas leakage will be of benefit. In terms of specific activities, many environmental risks are caused by hydraulic fracturing activities, such as minor earthquakes and water pollution. As for water consumption and pollution, the hydraulic fracturing process consumes the most water (see Figure 3) and the combination of hydraulic fracturing and horizontal drilling produces a large amount of wastewater (called “produced water”) (Clark et al., 2013). Fortunately, improvements in technology, such as membrane technology (membrane distillation, forward osmosis, membrane bioreactor, and pervaporation) and advanced oxidation processes (ozone oxidation, Fenton, and photocatalysis) can help to a degree with treatment of such water (Silva, 2017).
FIGURE 3. Water consumption for conventional and shale gas production by life cycle stage, accounting for use of recycled flowback water in hydraulic fracturing. Min and max scenarios show the extent of variability in water consumption by key processes (Silva et al., 2017).
Natural gas is normally presented in one of three states: gaseous natural gas (PNG), compressed natural gas (CNG), and liquid natural gas (LNG). Transportation is generally carried out by pipelines. Trains and ships are used as an effective supplementary method to transport CNG and LNG by land or sea (Zhao et al., 2015).
The natural gas pipeline system is mainly composed of gas gathering pipelines, dry gas transmission pipelines, domestic gas distribution pipelines, and related gas transmission (compression) fields and stations. The equipment and devices used start at the wellhead of the gas field, collect the gas, purify and transport it through the main pipeline, and then distribute it to the users through the gas distribution network (Figure 4) (Li, 2014).
FIGURE 4. The overall process of the natural gas gathering and transportation pipeline system (Li, 2014).
Most pipelines are buried underground, traverse a wide area and complex terrain. Xu et al. (2014) and others believe that the soil and vegetation damage caused by the excavation of pipe trenches during the construction process is more serious than the pollution impact produced after the pipeline is put into operation. During the pipeline construction process, the rolling of machinery and vehicles will disturb the soil; destroy the natural vegetation near the pipeline; discharge more waste water, waste gas, and waste residue; and produce more waste soil and rock. The layout of infrastructure such as pipelines and roads under construction destroys 2.9–3.6 ha of habitat and may even block rivers and cause ecosystem fragmentation (Brittingham et al., 2014). Tens of thousands of gallons of chemical additives per well are transported by trucks to well pads for storage. If not managed properly, these chemicals may leak or overflow from inappropriate storage containers or during transportation. Drilling mud, diesel, and other liquids can also spill on the surface (Wiseman, 2013). Yazdan et al. (2020) pointed out that in the process of wastewater transportation, land resources may be polluted due to pipeline breaks.
Luo et al. (2008) found that process units such as stations, pipe sections, and shut-off valve chambers in natural gas pipeline facilities can lead to environmental risks. When the critical mass of natural gas production sites is greater than 1t, it is a major hazard to install actions (Zhong et al., 2017). Due to the nature of some of the components in the gas transported, such as hydrogen sulfide, condensate oil and gas field water, the potential danger to the environment is substantial. For sulfur-containing natural gas pipelines, hazardous substances include hydrogen sulfide and sulfur dioxide. For the purification of natural gas pipelines, the main hazard is methane. For natural gas pipelines, the risks for accidents are leakage, explosion, and fire (Zhong et al., 2017). It is generally believed that leakage has little effect on water and soil because natural gas has low toxicity and is a gas at room temperature (He, 2009). However, Zhong et al. (2017) noted that it is difficult to conduct daily inspections of pipelines, and the pipelines are vulnerable to corrosion and breaks leading to leaks. Once the integrity of the pipeline is compromised, gas will leak and spread quickly in large quantities in a short period of time, which is likely to cause major accidents such as explosions and poisoning, causing heavy losses of lives and damage to property and even resulting in serious environmental pollution. Yuan et al. (2019) concluded that methane leakage during transportation accounts for 42–86% of the total methane leakage in the total supply chain and is the single largest cause of leakage.
CH4 and CO2 are both greenhouse gases and are usually present in natural gas albeit in widely varying concentrations. Therefore, the leakage of natural gas during transportation can release a large amount of CH4 and CO2 into the atmosphere, further enhancing the greenhouse effect. Studies have shown that there is serious leakage in natural gas transportation. Bylin et al. (2009) found that methane emissions from natural gas distribution pipelines account for 32% of the industry’s total CH4 emissions in the United States. 5.3–10.8% of the natural gas that flows through the United Kingdom's natural gas pipelines leaks each year (McKenzie, 2010). The impact on the greenhouse effect depends on the amount of gas leakage. McKenzie (2010) used data from the United Nations Intergovernmental Panel on Climate Change to study the problem of natural gas leakage. It was found that when the natural gas leakage rate reaches 2.8%, the greenhouse effect it brings will offset the advantages from not using fossil fuels such as oil and coal. Other studies suggest substantial gas leakage. The United States Environmental Protection Agency (EPA) estimated that methane leakage from natural gas systems doubled during 2011. The EPA’s estimate of methane content shows that natural gas network leaks and emissions between production wells and the local distribution network comprised approximately 570 billion cubic feet in 2009, equivalent to 2.4% of the total United States natural gas production. Recent studies in the United States have shown that the leakage and combustion emissions of natural gas processing and distribution systems are serious (ICF International, 2014). Other studies have shown that solving the problem of natural gas leakage can effectively slow down the greenhouse effect. Alvarez et al. (2012) found that if the “well-to-wheel” leakage is reduced to an effective natural gas leakage rate of 1.6%, CNG fuel vehicles will immediately lead to climate benefits over time. Cooper and Balcombe (2019) adopted IPCC AR5 LCIA methodology to calculate the impacts on climate change using global warming potential (GWP) CO2 equivalences and found that LNG exhibits a lower GWP (17–21%) than diesel, and thus using natural gas as an alternative energy source can reduce the greenhouse effect.
On the whole, gas collection, laying of pipelines and final delivery of gas to user departments and gas storage can bring about environmental risks to the soil ecology and the atmosphere due to the greenhouse effect. The construction of pipelines and roads can cause soil, vegetation damage and habitat loss. In the transportation process of chemical additives, the leakage of the chemicals can cause soil pollution. In general, gas leakage is a risk and can be difficult to manage, leading to air pollution, potential explosion, and increase of the greenhouse effect. Although it is difficult to conduct daily inspections of pipelines, this study has concluded that the leakage in natural gas transportation is a serious issue. If we want to use natural gas as an alternative energy source to reduce the greenhouse effect, the leakage must be minimized. Thus, at this stage, the key point is to control gas leakage.
The use of natural gas downstream can be roughly divided into two categories: chemical raw material and fuel. As for chemical raw materials, it is mainly used to make synthetic ammonia and urea, and the pollutants from the production process are mainly CO2, waste liquid, etc., which is relatively an ideal and economical production method (Wang et al., 1995). In terms of global natural gas utilization, industrial fuel accounts for 40%, power generation accounts for 37%, domestic gas accounts for 21%, and transportation stations account for 2%. The development of natural gas usage in a typical country is typically driven initially by industrial and domestic gas consumption and subsequently by power generation. At this stage, the main environmental impact is increased air pollution, greenhouse effect, and water consumption. Considering the different technology in power plant, carbon dioxide emissions produced by burning natural gas in existing power plants in the United States are equivalent to 42–63% of those from coal (Lattanzio, 2015) for an equivalent amount of energy produced. Mackay and Stone (2013) found that, for natural gas to have lower life cycle emissions than new coal plants over short time frames of 20 years or less, methane losses must be kept below 3.2 percent, and low emissions depend on using new technology.
The use of natural gas will produce NOx, CO2, CH4, etc., but it causes little air pollution. The burning of natural gas does produce NOx, but the content is lower than that of combustion products from gasoline and diesel for automobiles. Using natural gas can improve air quality. Analysis by the United States Department of Energy suggests that every 10,000 United States households that use natural gas instead of coal can avoid emitting 1,900 tons of NOx, 3,900 tons of SO2, and 5,200 tons of particulate matter per year (National Renewable Energy Laboratory, 1999). The reduction of these air pollutants can alleviate health conditions such as asthma, bronchitis, lung cancer, and heart disease for thousands of Americans (Witherspoon, 2012). Che et al. (2017) found that CO2 emissions from gas-fired power generation and heating are close to 1/2 of that of coal-fired power generation and heating. CH4 emissions are less than 1/3 of coal-fired emissions, and N2O emissions are less than 1/10 of coal-fired emissions. In the transportation and residential areas, the use of natural gas has greatly reduced CO2, CH4, and N2O emissions. Sathaye et al. (2011) and others also found that, compared with coal power generation, natural gas power generation can significantly reduce the emission of air pollutants. Compared to other fossil fuels, the amount of sulfur, mercury, and particulates produced by natural gas combustion is also negligible (Table 1).
Research studies have not yet resulted in a clear and consistent view on the magnitude of the greenhouse effect brought about by the use of natural gas. Some studies believe that replacing traditional energy sources with natural gas can significantly reduce greenhouse gas emissions. Fulton et al. (2011) used the latest emission factor from the EPA's 2011 upward revisions, through top-down life-cycle analysis, and found that the greenhouse gas emissions from natural gas power generation are 47% lower than those of coal-fired power generation. Afsah and Salcito (2012), according to the environmental assessment method, used short-term price elasticity changes and the substitution relationship between natural gas and other energy sources and found that the replacement of coal by shale gas in the United States power sector has reduced carbon dioxide emissions by 35–50% in recent years. From 2008 to 2012, fossil fuel in the United States power sector fell by 13% based on the supply model of cost-optimal mix technologies (Logan et al., 2013). Expanding natural gas power generation capacity is the cheapest way to advance the use of low-carbon energy technologies in the next ten years (Lee et al., 2012). Yuan and Yang (2019) also found that the CH4 emissions from CBM are relatively small and will not significantly increase the total emissions of greenhouse gas and that replacing coal with CBM can also offset some greenhouse gas emissions. In the field of transportation, natural gas buses have low greenhouse gas emissions, which can make the greenhouse gas emission reduction potential reach 13.31% (Wang, 2015).
However, other studies have shown that the large supply of natural gas could cause an increase in overall energy consumption in the absence of strict policy implementation, which may increase greenhouse gas emissions that offset the reduction in net greenhouse gas emissions by switching from coal to gas (McJeon et al., 2014). Perhaps the actual situation is more complicated. Ravindra et al. (2006) evaluated various standard air pollutants (SPM, PM10, CO, SO2, and NOx), benzene, toluene, xylene (BTX) and the concentration of polycyclic aromatic hydrocarbons (PAHs) before and after the implementation of CNG in Delhi, India, and found that the concentration of PAHs, SO2, and CO showed a downward trend, while the level of NOx was higher than before CNG was implemented, and the concentrations of SPM, PM10, and BTX did not change significantly after CNG was implemented. That is to say, the greenhouse effect brought about by CNG applications is uncertain.
Research by Jenner and Lamadrid (2013) found that coal and natural gas demand roughly the same water consumption during the production and processing stages, but water consumption during the combustion and power generation stages is significantly lower than that of coal. Meldrum et al. (2013) found that in the process of power generation, natural gas life cycle water consumption is 220–1,000 L per thousand kilowatt-hours of electricity, while coal consumption is 1,500–2,500 L. Clark et al. (2013) found that when natural gas is used as a transportation fuel, its water consumption is significantly lower than other transportation fuels. Compared with the operation of a power plant, the water consumption of fuel has little impact. Natural gas needs to be compressed first when used as a car fuel tank, and electricity consumption during the compression process consumes an additional 0.6–0.8 L of water. Macknick et al. (2011) pointed out that among all power plants that use circulating cooling, natural gas power plants consume the least water, which is a little more than half of the most water-efficient coal-fired power plants. When the same cooling technology is used, it is less than 1/3 of the water consumption of nuclear power plants. In summary, during the use of natural gas, a small amount of water is consumed, and the amount of water consumed depends on the type of usage.
In summary, the burning of natural gas downstream generates less emission of pollutants and causes little pollution compared with coal and gasoline. Thus, it can improve the air quality if it replaces coal or gasoline consumption. However, the greenhouse effect accompanied by the usage of gas is hard to establish. The varying degrees of impact depends on different usages and the different technologies adopted. Generally speaking, natural gas can reduce greenhouse gas effects in the power generation sector. However, there is no doubt that reducing greenhouse gases requires speeding up adoption of new technology. For water consumption, natural gas requires less water in power generation stages and as a transportation fuel than coal. We expect that the more advanced the production technology, the less water will be consumed in the gas consumption process.
In terms of the entire life cycle of natural gas from extraction to using, some environmental risks exist at every stage. In the upstream phase, some toxic gas in the air may influence the health of both wildlife and human beings who live nearby; in the middle stage, the leakage of gas may cause explosion; in the downstream stage, the burning of gas will generate some pollution, but that will have less impact than that of coal and gasoline, and the pollution will vary depending on the type of usage. At every stage, regular human activities, such as drilling, hydraulic fracturing, and pipe laying, will cause some impact to land use, soil, wildlife, and the ecosystem as a whole. With regard to water consumption and pollution, there is a huge negative impact in the upstream phase and a more positive influence in other stages. The extent of the impact depends on the specific utilization, working activities, and the adoption of technology, especially for hydraulic fracturing. However, the biggest controversy is whether natural gas can reduce the greenhouse effect compared to other source of energy. The biggest uncertainty in this regard is the scale of gas leakage. Thus, making efforts to control and reduce the level of leakage of natural gas will enhance the environmental quality.
Firstly, strengthen environmental assessment management. Establish environmental assessment procedures for pre-, mid-, and posthydraulic fracturing; fracturing operations; and wastewater treatment. The environmental impact assessment of natural gas exploitation can prevent environmental pollution and ecological damage (Wu et al., 2018). The advancement of monitoring technologies and data-analysis technologies in the digital era make this task more practical and efficient. The comprehensive policies, regulations, and standards of the United States have played a guiding role in the environmental assessment work by other countries. During project preparation, design, and approval, monitor the integrity comprehensively, monitor environmental indicators in real time, and conduct environmental risk emergency management in advance.
Secondly, improve policy standards. Formulate strict environmental supervision policies, especially clarify the treatment standards for wastewater reinjection, wastewater treatment, and fracturing fluid composition for hydraulic fracturing. It is necessary to learn from the mature regulatory systems of the United States and Canada to improve the well spacing and casing integrity standards during the oil well construction process (Gagnon et al., 2016). Develop sensible well spacing, and limit the distance to houses, buildings, etc. Enact strict casing integrity regulations to prevent oil, gas, and water from migrating from one formation to another, thereby ensuring the safety of the surrounding ground and surface water.
Thirdly, make a water quality monitoring plan specifically for hydraulic fracturing. The United States Environmental Protection Agency (2015) pointed out that the lack of data and related chemical information collected before and during hydraulic fracturing operations will reduce the ability to identify hydraulic fracturing contaminated areas. It is vital to make a plan for water quality monitoring. Determine water quality monitoring standards, including monitoring time, location, and the number of detections. Identify potential pollutants, determine the priority of pollutant hazards test chemical composition, and make a list of water quality analytes. Based on the water quality monitoring data, formulate a regional water quality supervision plan.
Fourthly, promote the innovation of key technologies. Further improve green drilling and completion technologies, efficient drilling technologies, and recycling technologies to reduce environmental pollution from drilling activities. Promote technological breakthroughs in the direction of waterless fracturing technology, key oil production engineering technologies, and intelligent gas production technologies. Government subsidies and financial support will quickly promote the development and application of technology substitution.
Firstly, in the process of natural gas storage and transportation, pipeline laying and storage site selection should be as far away as possible from environmentally sensitive areas and residential areas, and an environmental assessment should be prepared with in-depth demonstrations of specific construction and environmental risks.
Secondly, strengthen the leak detection of methane and volatile organic compounds, and then take effective measures to prevent and manage risks. Continuously improve monitoring technology of methane emissions. There are already some technologies that can help us monitor the leakage of natural gas pipelines, such as infrared imaging technology, olfactory sensor leak detection technology, schlieren imaging technology, gas detection method, and distributed optical fiber acoustic sensor detection method.
Thirdly, strengthen the quality control of engineering materials. Pipeline gas engineering is usually constructed based on its expected service life. The quality of engineering pipes and equipment has a direct impact on the service life of the project. The main reason for leakage of natural gas is defects in the pipe equipment (Peng et al., 2013). Therefore, the entire process of manufacturing pipe equipment, from design, model selection, bidding, quality inspection, procurement, and transportation to construction, requires a sound quality assurance system.
In addition, urban gas has the closest connection to the residents. In order to reduce the hazardous consequences, frequency and impact of accidental gas fires and explosions caused by the rupture of urban gas pipelines, the risk identification and management of urban gas pipelines should be strengthened. This should include design, construction, daily maintenance, and strict control of the aging and connection of pipes. Strengthen safety training and guidance, and prepare accident emergency plans in advance.
It is shown that gas utilization is more efficient than coal-fired equipment. Gas-fired power plants have a thermal efficiency of more than 10%, and cogeneration and distributed energy have a thermal efficiency of more than 20% (He, 2016). Compared with ordinary coal-fired units and "ultra-low emission" units, gas-fired power plants have obvious advantages in terms of reduced emissions. Among them, smoke and dust can be reduced by 14–80%,
After systematically reviewing the studies on the whole life cycle of the upper, middle, and lower stages of the natural gas industry chain, we focused on identifying environmental risks such as air pollution, land use, water use, ecological impact, and greenhouse effect at various stages. There are some conclusions that can help us carry out more targeted work.
Firstly, although there are environmental risks in the natural gas production and supply process, they can be reduced through technologies and measures. Secondly, the usage of natural gas is accompanied by the release of greenhouse gases. However, compared with traditional energy sources such as coal, environmental pollution, the greenhouse effect, and the ecological impact of natural gas are much smaller. Thirdly, through this literature review and identification of various environment risks, we identify the key activities at different stages. During upstream exploitation, hydraulic fracturing has the worst environmental impact. In the midstream storage and transportation stage, methane leakage is the biggest environmental risk. In the downstream combustion and utilization stage, different application technologies will produce different resource consumption and emissions.
Therefore, this article puts forward some policy recommendations for different stages. In the upstream stage, for hydraulic fracturing activities in particular, we suggest strengthening environmental assessment management, improving policy standards, making a water quality monitoring plan, and promoting the innovation of key technologies. In terms of the midstream, besides pipeline laying and site selection, we focus on monitoring the system, including leak detection, quality management of engineering materials, and risk identification and management. When it comes to downstream, we encourage the application of advanced technologies to improve thermal efficiency and reduce emissions, such as gas-fired related technologies, natural gas recycling technologies, distributed energy technologies, and green and low-carbon service technologies.
The authors confirm being the sole contributors of this work and have approved it for publication.
The authors declare that the research was conducted in the absence of any commercial or financial relationships that could be construed as a potential conflict of interest.
Afsah, S., and Salcito, K. (2012). Shale gas and the overhyping of its CO2 reductions. Available at: https://thinkprogress.org/shale-gas-and-the-overhyping-of-its-co2-reductions-f576130f5bbe/ (Accessed August 7, 2012).
Airgas (2013). Material safety data sheet, methane. Available at: http://louisville.edu/micronano/files/documents/safety-data-sheets-sds/Methane.pdf (Accessed January 16, 2013).
Allen, D. T., Torres, V. M., Thomas, J., Sullivan, D. W., Harrison, M., Hendler, A., et al. (2013). Measurements of methane emissions at natural gas production sites in the United States. Proc. Nat. Acad. Sci. 110 (44), 17768–17773. doi:10.1073/pnas.1304880110
Alvarez, R. A., Pacala, S. W., Winebrake, J. J., Chameides, W. L., and Hamburg, S. P. (2012). Greater focus needed on methane leakage from natural gas infrastructure. Proc. Nat. Acad. Sci. 109 (17), 6435–6440. doi:10.1073/pnas.1202407109
Amini, A., and Eberhardt, E. (2019). Influence of tectonic stress regime on the magnitude distribution of induced seismicity events related to hydraulic fracturing. J. Petrol. Sci. Eng. 182, 106284. doi:10.1016/j.petrol.2019.106284
BP (2020). Statistical review of world energy 2020: a pivotal moment. Available at: https://www.bp.com/en/global/corporate/news-and-insights/press-releases/bp-statistical-review-of-world-energy-2020-published (Accessed June 17, 2020).
Brandt, A. R., Heath, G. A., Kort, E. A., O'Sullivan, F., Pétron, G., Jordaan, S. M., et al. (2014). Methane leaks from North American natural gas systems. Science 343 (6172), 733–735. doi:10.1126/science.1247045
Breidenich, C., Magraw, D., Rowley, A., and Rubin, J. (1998). The kyoto protocol to the united Nations framework convention on climate change. Am. J. Int. Law. 92 (2), 315–331. doi:10.2307/2998044
Breitling, Oil, and Gas (2012). United States shale faces water.transparency complaints. Available at: http://www.breitlingoilandgas.com/us-shale-faces-water-transparency-complaints/ (Accessed October 4, 2012).
Brink, S., Singer, L. E., Sengupta, M., Godfrey, H., Lyser, S., and Mignotte, S. (2013). “Methane mitigation opportunities in China,” in Woodrow wilson School of public and international affairs graduate policy workshop. (Princeton, NJ: Princeton University), 1–55.
Brittingham, M. C., Maloney, K. O., Farag, A. M., Harper, D. D., and Bowen, Z. H. (2014). Ecological risks of shale oil and gas development to wildlife, aquatic resources and their habitats. Environ. Sci. Technol. 48 (19), 11034–11047. doi:10.1021/es5020482
Burton, A., and Nadelhoffer, K. (2013). Report N0.: 4. Hydraulic fracturing in the state of Michigan-Environment/Ecology technical report. Available at: http://hdl.handle.net/2027.42/102577 (Accessed September 03, 2013).
Bylin, C., Cassab, L., Cazarini, A., Ori, D., Robinson, D., and Sechler, D. (2009). New measurement data has implications for quantifying natural gas losses from cast iron distribution mains. Pipeline Gas J. 236 (9), 32–38. doi:10.2523/77925-MS
Che, M., Shan, W. P., Yu, X. D., Wu, Y. Y., and Liu, A. D. (2017). The development process, status quo and enlightenment of the British natural gas industry. Sino-Foreign Energy. 22 (011), 11–18. doi:10.3969/j.issn.1003-2355.2017.04.006
Chen, S. S., Sun, Y., Tsang, D. C., Graham, N. J., Ok, Y. S., Feng, Y., et al. (2017). Potential impact of flowback water from hydraulic fracturing on agricultural soil quality: metal/metalloid bioaccessibility, Microtox bioassay, and enzyme activities. Sci. Total Environ. 579, 1419–1426. doi:10.1016/j.scitotenv.2016.11.141
Clark, V. E., Erson-Omay, E. Z., Serin, A., Yin, J., Cotney, J., Ozduman, K., et al. (2013). Genomic analysis of non-NF2 meningiomas reveals mutations in TRAF7, KLF4, AKT1, and SMO. Science. 339 (6123), 1077–1077. doi:10.1126/science.1233009
Colborn, T., Kwiatkowski, C., Schultz, K., and Bachran, M. (2011). Natural gas operations from a public health perspective. Human and ecological risk assessment. Int. J. 17 (5), 1039–1056. doi:10.2172/1007397
Cooper, J., and Balcombe, P. (2019). Life cycle environmental impacts of natural gas drivetrains used in road freighting. Procedia CIRP. 80, 334–339. doi:10.1016/j.procir.2019.01.070
Crow, D. J., Balcombe, P., Brandon, N., and Hawkes, A. D. (2019). Assessing the impact of future greenhouse gas emissions from natural gas production. Sci. Total Environ. 668, 1242–1258. doi:10.1016/j.scitotenv.2019.03.048
Dong, Z. Q., Ma, X. Q., Zhang, L., and Zhao, Z. L. (2003). Life cycle analysis of the impact of natural gas utilization on the environment. Nat. Gas Ind. 6, 126–130. doi:10.3321/j.issn:1000-0976.2003.06.036
EIA United States Energy Information Administration (2018). How much carbon dioxide is produced when different fuels are burned 2018. Available at: https//www.eia.gov/tools/faqs/faq.php?id=73&t=11 (Accessed April 11, 2018).
EPA Environmental Protection Agency (2013b). Ground level ozone. Available at: https://www.epa.gov (Accessed August 14, 2013).
EPA Environmental Protection Agency (2013a). Natural gas extraction—hydraulic fracturing. Available at: https//www.epa.gov (Accessed July 12, 2013).
ICF International (2014). Economic analysis of methane emission reduction opportunities in the US onshore oil and natural gas industries. Available at: https://www.ccacoalition.org/en/resources/ (Accessed March 1, 2014).
Freyman, M. (2014). Hydraulic fracturing and water stress, Water demand by the numbers. Boston, MA: Ceres, 85.
Fulton, M., Mellquist, N., Kitasei, S., and Bluestein, J. (2011). Comparing life-cycle greenhouse gas emissions from natural gas and coal. Alemanha: Worldwatch Institute, 1–29.
Gagnon, G. A., Krkosek, W., Anderson, L., Mcbean, E., Mohseni, M., Bazri, M., et al. (2016). Impacts of hydraulic fracturing on water quality: a review of literature, regulatory frameworks and an analysis of information gaps. Environ. Rev. 24 (2), 122–131. doi:10.1139/er-2015-0043
Gallegos, T. J., Varela, B. A., Haines, S. S., and Engle, M. A. (2015). Hydraulic fracturing water use variability in the United States and potential environmental implications. Water Resour. Res. 51 (7), 5839–5845. doi:10.1002/2015WR017278
Granoff, I., Pickard, S., Doczi, J., Calow, R., Hou, Z. B., and D’Alancon, V. (2015). Can fracking green China's growth? Opportunities, risks and recommendations for unconventional gas in China's environmental transformation. Available at: https://www.odi.org/sites/odi.org.uk/files/odi-assets/publications-opinion-files/9609.pdf (Accessed April 23, 2015).
He, P. F. (2016). Discussion on natural gas power generation technology. Sci. Technol. Wind. 3, 23. doi:10.3969/j.issn.1671-7341.2016.06.022
He, X. Z. (2009). Discussion on several elements of environmental risk assessment during operation period of natural gas long-distance pipeline project. Energy Environ. 3, 76–77. doi:10.3969/j.issn.1672-9064.2009.03.033
Huang, J. L., Zhang, W. B., Qiu, X. Y., and Zhou, J. (2018). Research progress on the characteristics of coalbed methane produced water and its environmental impact. Environ Impact Assess. 40 (3), 36–38.
IPCC Intergovernmental Panel on Climate Change (2007). Report No.: AR4. Direct global warming potentials. Available at: https://www.ipcc.ch/assessment-report/ (Accessed September, 2007).
Jenner, S., and Lamadrid, A. J. (2013). Shale gas vs. Coal, policy implications from environmental impact comparisons of shale gas, conventional gas, and coal on air, water, and land in the United States. Energy Policy 53, 442–453. doi:10.1016/j.enpol.2012.11.010
Kondash, A. J., Albright, E. A., and Vengosh, A. (2017). Quantity of flowback and produced waters from unconventional oil and gas exploration. Sci. Total Environ. 574, 314–321. doi:10.1016/j.scitotenv.2016.09.069
Lattanzio, R. K. (2015). Life-cycle greenhouse gas assessment of coal and natural gas in the power sector. Available at: https://nationalaglawcenter.org/wp-content/uploads/assets/crs/R44090.pdf (Accessed June 26, 2015).
Lee, A., Zinamen, O., Logan, J., Bazillian, M., Arent, D., and Newmark, R. (2012). Interactions, complementarities and tensions at the nexus of natural gas and renewable energy. Elect. J. 25 (10), 38–48. doi:10.1016/j.tej.2012.10.021
Li, Y. R. (2014). Environmental riskS assessment of natural gas gathering and transportation pipelines based on fuzzy comprehensive evaluation. Strait Sci. 06, 47–51. doi:10.1016/0025-326X(93)90482-Y
Li, Y., T, D. M., L, X., M, X. X., Y, L., X, Y., et al. (2019). Soil heavy metal content characteristics and ecological risk assessment of shale gas well sites. J. Sichuan Agric. Univ. 5, 15.
Litovitz, A., Curtright, A., Abramzon, S., Burger, N., and Samaras, C. (2013). Estimation of regional air-quality damages from Marcellus Shale natural gas extraction in Pennsylvania. Environ. Res. Lett. 8 (1), 014017. doi:10.1088/1748-9326/8/1/014017
Logan, J., Lopez, A., Mai, T., Davidson, C., Bazillian, M., and Arent, D. (2013). Natural gas scenarios in the US power sector. Energy Econ. 40 (0), 183–195. doi:10.1016/j.eneco.2013.06.008
Luo, X. L., Xiang, Q. G., Yin, X. B., Zhou, D., and Yan, Z. P. (2008). Recognition on environmental risk assessment of natural gas pipelines. Chem. Eng. Oil Gas. 37 (06), 532–534. doi:10.3969/j.issn.1007-3426.2008.06.020
Mac Kinnon, M. A., Brouwer, J., and Samuelsen, S. (2018). The role of natural gas and its infrastructure in mitigating greenhouse gas emissions, improving regional air quality, and renewable resource integration. Prog. Energy Combust. Sci. 64, 62–92. doi:10.1016/j.pecs.2017.10.002
Mackay, D. J. C., and Stone, T. J. (2013). Potential greenhouse gas emissions associated with shale gas extraction and use. London, United Kingdom: Department of Energy and Climate Change, 50.
Macknick, J., Newmark, R., Heath, G., and Hallett, K. C. (2011). Report No.: NREL/TP-6A20-50900. Review of operational water consumption and withdrawal factors for electricity generating technologies. Available at: https://www.nrel.gov/docs/fy11osti/50900.pdf (Accessed March 1, 2011).
McCabe, D., Geertsma, M., Matthews, N., Fleischman, L., and Schroeder, D. (2015). Waste not common sense ways to reduce methane pollution from the oil and natural gas industry. Available at: https://www.nrdc.org/resources/waste-not-common-sense-ways-reduce-methane-pollution-oil-and-natural-gas-industry (Accessed November 19, 2014).
McJeon, H., Edmonds, J., Bauer, N., Clarke, L., Fisher, B., Flannery, B. P., et al. (2014). Limited impact on decadal-scale climate change from increased use of natural gas. Nature 514 (7523), 482–485. doi:10.1038/nature13837
McKenzie, D. (2010). Leaking gas mains help to warm the globe. Available at: https://www.newscientist.com/article/mg12717351-100-leaking-gas-mains-help-to-warm-the-globe/ (Accessed September 22, 1990).
McKenzie, L. M., Witter, R. Z., Newman, L. S., and Adgate, J. L. (2012). Human health risk assessment of air emissions from development of unconventional natural gas resources. Sci. Total Environ. 424, 79–87. doi:10.1016/j.scitotenv.2012.02.018
Milkov, A. V., Claypool, G. E., Lee, Y. J., and Sassen, R. (2005). Gas hydrate systems at Hydrate Ridge offshore Oregon inferred from molecular and isotopic properties of hydrate-bound and void gases. Geochim. Et Cosmochimica Acta. 69 (4), 1007–1026. doi:10.1016/J.GCA.2005.08.021
Meldrum, J., Nettles-Anderson, S., Heath, G., and Macknick, J. (2013). Life cycle water use for electricity generation, a review and harmonization of literature estimates. Environ. Res. Lett. 8 (1), 015031. doi:10.1088/1748-9326/8/1/015031
Mohan, A. M., Hartsock, A., Bibby, K., Hammack, R., Vidic, R. D., and Gregory, K. B. (2013). Microbial community changes in hydraulic fracturing fluids and produced water from shale gas extraction. Environ. Sci. Technol. 47 (22), 13141–13150. doi:10.1021/es402928b
Moortgat, J., Schwartz, F. W., and Darrah, T. H. (2018). Numerical modeling of methane leakage from a faulty natural gas well into fractured tight formations. Groundwater 56 (2), 163–175. doi:10.1111/gwat.12630
Mordick, B. (2014). Leaky oil and gas wells threaten the environment and public health and safety. New York, NY: NRDC, 1–29.
National Renewable Energy Laboratory (1999). Life cycle assessment of Coal-fired Power Production. Available at: https://www.osti.gov/biblio/12100-life-cycle-assessment-coal-fired-power-production (Accessed September 1, 1999).
National Research Council (2013). Induced seismicity potential in energy technologies. Washington, DC: The National Academies Press, 262.
Ou, X. M., and Yuan, Z. Y. (2019). Life cycle analysis on liquefied natural gas and compressed natural gas in heavy-duty trucks with methane leakage emphasized. Energy Procedia. 158, 3652–3657. doi:10.1016/j.egypro.2019.01.896
Peng, H. T., Guo, D., and Duan, M. S. (2013). Discussion on environmental risk assessment and management of urban gas pipelines. Environ. Prot. Oil Gas Fields 5, 68–71. doi:10.3969/j.issn.1005-3158.2013.05.023
Rahm, B. G., and Riha, S. J. (2014). Evolving shale gas management, water resource risks, impacts and lessons learned. Environ Sci. Process. Impacts 16, 1400–1412. doi:10.1039/c4em00018h
Ravindra, K., Wauters, E., Tyagi, S. K., Mor, S., and Van Grieken, R. (2006). Assessment of air quality after the implementation of compressed natural gas (CNG) as fuel in public transport in Delhi, India. Environ. Monitor. Assess. 115 (1–3), 405–417. doi:10.1007/s10661-006-7051-5
Rodriguez, J., Heo, J., and Kim, K. H. (2020). The impact of hydraulic fracturing on groundwater quality in the Permian Basin. Water. 12 (3), 796. doi:10.3390/w12030796
Sathaye, J., Lucon, O., Rahman, A., Christensen, J., Denton, F., Fujino, J., et al. (2011). Renewable energy in the context of sustainable development. Int. J. Energy Environ. Eng. 4, 42. doi:10.1186/2251-6832-4-42
Shen, X., Tu, Y. D., Wang, J., and Chen, J. D. (2016). Strategic direction selection and policy recommendations for China's natural gas utilization. Int. Petrol. Econ. 10, 69–78.
Shonkoff, S. B. C., Hays, J., and Finkel, M. L. (2014). Environmental public health dimensions of shale and tight gas development. Environ. Health Perspect. 122 (8), 787–795. doi:10.1289/ehp.1307866
Silva, T. L., Morales-Torres, S., Castro-Silva, S., Figueiredo, J. L., and Silva, A. M. (2017). An overview on exploration and environmental impact of unconventional gas sources and treatment options for produced water. J. Environ. Manag. 200, 511–529. doi:10.1016/j.jenvman.2017.06.002
Stark, M., Blackford, T., Vargas, M., Sorice, J., and Davis, M. (2014). Achieving high performance in unconventional operations Integrated planning, services, logistics and materials management. Available at: https://www.accenture.com/t20160513t024221__w__/in-en/_acnmedia/accenture/conversion-assets/dotcom/documents/global/pdf/dualpub_11/accenture-high-performance-unconventional-operations.pdf (Accessed October 20, 2014).
Thacker, J. B., Carlton, D. D., Hildenbrand, Z. L., Kadjo, A. F., and Schug, K. A. (2015). Chemical analysis of wastewater from unconventional drilling operations. Water 7 (4), 1568–1579. doi:10.3390/w7041568
Van der Elst, N. J., Savage, H. M., Keranen, K. M., and Abers, G. A. (2013). Enhanced remote earthquake triggering at fluid-injection sites in the midwestern United States. Science 341 (6142), 164–167. doi:10.1126/science.1238948
Vidic, R. D., Brantley, S. L., Vandenbossche, J. M., Yoxtheimer, D., and Abad, J. D. (2013). Impact of shale gas development on regional water quality. Science 340 (6134), 1235009. doi:10.1126/science.1235009
Villa, V., and Singh, R. P. (2020). Hydraulic fracturing operation for oil and gas production and associated earthquake activities across the United States. Environ. Earth Sci. 79, 1–11. doi:10.1007/s12665-020-09008-0
Wang, G., Liu, Y., and Xu, J. (2020). Short‐term failure mechanism triggered by hydraulic fracturing. Energy Sci. Eng. 8 (3), 592–601. doi:10.1002/ese3.535
Wang, H., Li, Q. J., and Tang, S. R. (1995). Discussion on the domestic production plan of using Tarim natural gas to produce chemical fertilizer. China Nitrogen Fert. 5, 3–9.
Wang, R. J. (2015). Fuel life cycle assessment of the energy environmental impact of electric vehicles and natural gas vehicles. Transp. Res. Part D. 75, 211–222. doi:10.1007/s11367-012-0440-9
Wang, Y. S., Wang, H. L., Liu, D. W., Yuan, M. L., and Jiang, H. T. (2014). The status quo and development trend of coalbed methane drilling technology in China. Nat. Gas Ind. 34 (8), 87–91. doi:10.3787/j.issn.1000-0976.2014.08.013
Wiseman, H. J. (2013). Risk and response in fracturing policy. SSRN, 84 (3), 729. doi:10.2139/ssrn.2017104
Witherspoon, C. (2012). Regulations to control greenhouse gas emissions from motor vehicles. Available at: https://ww2.arb.ca.gov/news/air-resources-board-and-usepa-join-forces-precision-reporting-greenhouse-gas-emissions (Accessed January 1, 2012).
Wu, L., Li, N., and Yang, Y. (2018). Prediction of air quality indicators for the Beijing-Tianjin-Hebei region. J. Clean. Produc. 196, 682–687. doi:10.1016/j.jclepro.2018.06.068
Xu, B., and Lin, B. (2019). Can expanding natural gas consumption reduce China's emissions? Energy Econ. 81, 393–407. doi:10.1016/j.eneco.2019.04.012
Xu, W. M., Lu, M. X., and Zhu, B. (2014). Study on ecological environmental risks and countermeasures faced by sichuan oil and gas exploration and development. Western Econ. Manag. Forum. 25 (04), 39–44. doi:10.3969/j.issn.2095-1124.2014.04.008
Yazdan, M. M., Ahad, M. T., Jahan, I., and Mazumder, M. (2020). Review on the evaluation of the impacts of wastewater disposal in hydraulic fracturing industry in the United States. Technologies 8 (4), 67. doi:10.3390/technologies8040067
Yuan, J. M., and Yang, D. M. (2019). Environmental impacts and countermeasures of coalbed methane development and utilization. Environ. Impact Assess. 41 (04), 32–35. doi:10.1093/molbev/msn221
Yuan, Z., Ou, X., Peng, T., and Yan, X. (2019). Life cycle greenhouse gas emissions of multi-pathways natural gas vehicles in China considering methane leakage. Appl. Energy 253, 113472. doi:10.1016/j.apenergy.2019.113472
Zammerilli, A., Murray, R. C., Davis, T., and Littlefield, J. (2014). Environment impacts of unconventional natural gas development and production. Pittsburgh, PA: DOE, National Energy Technology Laboratory, Office of Fossil Energy, 58.
Zhao, H., Jin, X., and Hu, X. L. (2015). Application analysis of compressed natural gas pipeline transportation. Henan Sci. Technol. 33 (12), 125–126. doi:10.3969/j.issn.1003-5168.2015.12.046
Keywords: life cycle assessment, environmental risks, different stages, control measures, natural gas
Citation: Fu J, Liu Y and Sun F (2021) Identifying and Regulating the Environmental Risks in the Development and Utilization of Natural Gas as a Low-Carbon Energy Source. Front. Energy Res. 9:638105. doi: 10.3389/fenrg.2021.638105
Received: 05 December 2020; Accepted: 19 January 2021;
Published: 10 March 2021.
Edited by:
Jianliang Wang, China University of Petroleum, ChinaReviewed by:
Wenhuan Wang, Zhejiang University of Technology, ChinaCopyright © 2021 Fu, Liu and Sun. This is an open-access article distributed under the terms of the Creative Commons Attribution License (CC BY). The use, distribution or reproduction in other forums is permitted, provided the original author(s) and the copyright owner(s) are credited and that the original publication in this journal is cited, in accordance with accepted academic practice. No use, distribution or reproduction is permitted which does not comply with these terms.
*Correspondence: Jiaxin Fu, NjkxMDQ3NjcyQHFxLmNvbQ==
Disclaimer: All claims expressed in this article are solely those of the authors and do not necessarily represent those of their affiliated organizations, or those of the publisher, the editors and the reviewers. Any product that may be evaluated in this article or claim that may be made by its manufacturer is not guaranteed or endorsed by the publisher.
Research integrity at Frontiers
Learn more about the work of our research integrity team to safeguard the quality of each article we publish.