- 1Laboratory for Thin Films and Photovoltaics, Empa - Swiss Federal Laboratories for Materials Science and Technology, Dübendorf, Switzerland
- 2Institut Adolphe Merkle, Faculté des Sciences et de Médecine, Université de Fribourg, Fribourg, Switzerland
Hybrid halide perovskites feature mixed ionic-electronic conductivities that are enhanced under device operating conditions. This has been extensively investigated over the past years by a wide range of techniques. In particular, the suppression of ionic motion by means of material and device engineering has been of increasing interest, such as through compositional engineering, using molecular modulators as passivation agents, and low-dimensional perovskite materials in conjunction with alternative device architectures to increase the stabilities under ambient and operating conditions of voltage bias and light. While this remains an ongoing challenge for photovoltaics and light-emitting diodes, mixed conductivities offer opportunities for hybrid perovskites to be used in other technologies, such as rechargeable batteries and resistive switches for neuromorphic memory elements. This article provides an overview of the recent developments with a perspective on the emerging utility in the future.
Introduction
The development of a new generation of thin-film semiconductors has been propelled by the emergence of hybrid halide perovskites (Kim et al., 2012; Lee et al., 2012; Jena et al., 2019). These materials are commonly defined by the ABX3 formula (Figure 1A) that represents the ionic crystal structure comprised of a central (A) cation, which can be either inorganic (such as Cs+) or organic (methylammonium (MA, CH3NH3+), formamidinium (FA, CH(NH2)2+), etc.), encased by divalent-metal-halide-based (B = mostly Pb2+ or Sn2+; X = I−, Br− or Cl−) octahedral framework (Grätzel, 2017). Such versatile ionic systems feature remarkable light-absorption coefficients as well as a high defect tolerance due to an interplay of electronic and structural features (Meggiolaro et al., 2020) that can account for exceptional charge-carrier lifetimes (Kim and Petrozza, 2020) of interest to a number of optoelectronic devices, from solar cells and photodetectors to light-emitting diodes (Rong et al., 2018; Snaith, 2018). As a result, they have been proven effective light-absorbers in photovoltaic devices leading to extraordinary performances that have in just a decade surpassed 25% (Ehrler et al., 2020), along with exceptional light-emission (Abdi-Jalebi et al., 2018; Lin et al., 2018; Smith et al., 2019) and photodetection capacities (Lei et al., 2020). Their application has, however, been hampered by the limited stability under the environmental conditions, such as oxygen and moisture, as well as due to intrinsic instabilities under the operating conditions of voltage bias, light, and elevated temperature (Wang R. et al., 2019). This has been primarily the result of mixed conductivity and subsequent reactivity of mobile ions (Figure 1B) that is often associated with the degradation mechanisms during device operation (Wang R. et al., 2019), as well as the appearance of the hysteresis in current-voltage characteristics (Figure 1C), the absence of which does not imply absence of mobile ions (Tress, 2017; Zhao et al., 2019). A number of strategies have thus been established to mitigate the phenomena caused by ion migration (Figure 1D), such as the use of passivating agents (Milić et al., 2019b; Ferdani et al., 2019) and low-dimensional materials (Grancini and Nazeeruddin, 2019; Mao et al., 2019). There has also been a surge to use mixed conductivity in other emerging applications, such as rechargeable batteries (Tathavadekar et al., 2017; Dawson et al., 2018; Li et al., 2020) and resistive switches (Xiao and Huang, 2016; Choi et al., 2018; Lv et al., 2020). This article provides a perspective on the present challenges and opportunities associated with mixed conductivity of hybrid perovskites, from its phenomenology and mitigation strategies to utilization.
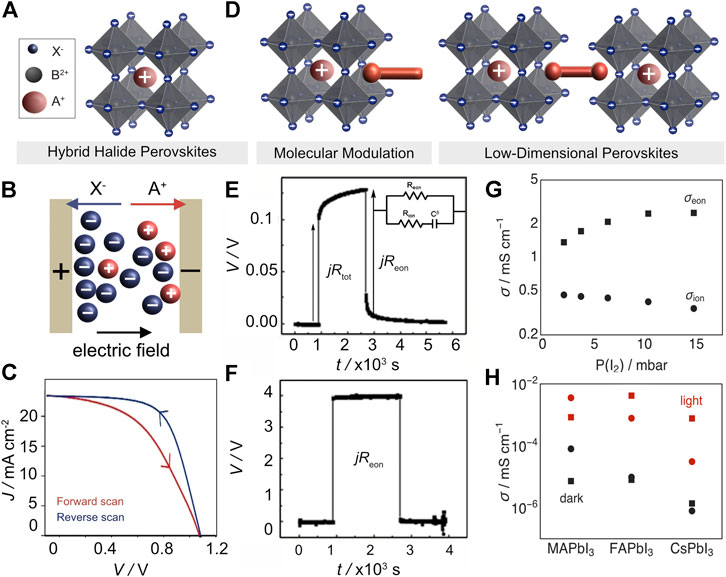
FIGURE 1. (A) Structural representation of 3D perovskites defined by the ABX3 formula illustrated by a cubic structure. (B) Illustration of mixed conductivity caused by ion migration in an electric field. (C) Hysteresis in current-voltage device characteristics that is commonly associated with ion migration. Based on the work published by the Royal Society of Chemistry (Weber et al., 2018). (D) Schematic of molecular modulation of hybrid perovskites (left) and layered low-dimensional perovskite architectures (right) based on organic moieties (red rods) (Graetzel and Milić, 2019). These structures can contribute to mitigating the detrimental effects of ionic motion, e.g., through interface, grain, and strain engineering. (E,F) Evolution of potential during a galvanostatic (1 nA) polarization measurement of MAPbI3 in the (E) dark and (F) under light illumination. Inset shows a simplified circuit model used to represent mixed conductivity, including electronic resistance (Reon), ionic resistance (Rion) and chemical capacitance (Cδ). Adapted with permission from Wiley VCH (Yang et al., 2015). (G,H) Electronic (square) and ionic (circle) conductivities under illumination (red) and in the dark (black) upon (G) iodine partial pressure of MAPbI3 [Adapted from Nature (Kim et al., 2018)] or (H) other perovskite compositions. Adapted from Wiley VCH (Kim et al., 2020).
Phenomenology
Halide perovskites are known to be ion conductors since the 1980s (Mizusaki et al., 1983). The recent developments in hybrid perovskite optoelectronics stimulated an increased interest in their mixed conductivity (Yang et al., 2015; Walsh and Stranks, 2018). The ionic conductivity is given by Eq. 1
where q is the charge,
where
where
To obtain a complete understanding of the ionic conductivity, the density of the mobile ions is of crucial importance. However, no consensus has yet been reached on the density of mobile ions, with reported values ranging from 1012 to 1019 cm−3(Birkhold et al., 2018; Moia et al., 2019; Reichert et al., 2020b; Duijnstee et al., 2021). Commonly used techniques for quantifying both the diffusion coefficient and the density of mobile ions include impedance spectroscopy and transient current and capacitance measurements (Almora et al., 2016; Bertoluzzi et al., 2018; Wang H. et al., 2019; Futscher et al., 2019). The advantage of these techniques is that the ion migration can be quantified in complete devices. However, these are indirect methods, which means that theoretical models must be used for quantification, which leads to a large spread in obtained mobile ion densities that can vary by several orders of magnitude depending on the model used (Bertoluzzi et al., 2020). Moreover, most models assume only a single mobile ion species, which is not always the case. As a result, there is an ongoing debate about the mobile ion density and its influence on the operation of perovskite-based devices (Moia et al., 2019; Caram et al., 2020; McGovern et al., 2020).
During illumination with photon energies above the band gap, both the electronic and the ionic conductivity increases (Figures 1G,H), with important implications on photo-induced phase separation (Brennan et al., 2020). There are a number of possible effects that can explain this behavior which have been studied experimentally (Xing et al., 2016; Kim et al., 2018; Motti et al., 2019) as well as theoretically (Katan et al., 2018; Li Y. T. et al., 2019; Meggiolaro et al., 2019). Here, we provide a brief overview of these effects to highlight their impact on ion migration. The density of mobile ions can be increased by an enhanced defect concentration, facilitated by interstitial formations due to hole capture or by non-radiative recombination (Stranks, 2017; Kim et al., 2020). In addition, the attempt frequency may be influenced by an enhanced electron-phonon coupling as indicated by first-principles calculation (Katan et al., 2018). Finally, the jump distance and the activation energy can be affected due to a change in the local crystal structure, such as by photostriction or by polaron formation, which leads to a local lattice deformation (Neukirch et al., 2016; Zhou et al., 2016; Muscarella et al., 2020). The number of mobile ion species, their ionic conductivity, and their behavior under illumination are strongly dependent on the perovskite composition (Figure 1H) (Kim et al., 2020). In this regard, further development of suitable models for a more universal approach to the analysis of hybrid halide perovskites is required.
Mitigation
Despite the remarkable optoelectronic properties of hybrid halide perovskites in light-emitting and photovoltaic devices, their limited stability poses challenges that are in part closely related to their mixed conductivity (Wang R. et al., 2019). In particular, ion migration that is accelerated under the operating conditions of voltage bias, light, and elevated temperature contributes to the gradual degradation during operation (Correa-Baena et al., 2016; Domanski et al., 2018; Akbulatov et al., 2020; Yan et al., 2021). While some of these processes are reversible, others lead to irreversible changes that need to be mitigated (Domanski et al., 2017; Tress et al., 2019). For instance, elevated temperatures during operation were found to induce ion migration from the neighboring layers, such as the counter-electrodes (e.g., Au) as well as dopants of the hole-transporting material (e.g., Li+), which act as defects in the active layer (Domanski et al., 2016). Similarly, ion migration and dopant reactivity are found to induce transformations in the selective charge-transport layers, such as the hole-transporting materials (Magomedov et al., 2018; Boldyreva et al., 2020), whereas electron-transporting layers were also found to affect the stability of hybrid perovskite devices through various (photo) redox processes (Akbulatov et al., 2020; Zhidkov et al., 2020). Moreover, photoinduced halide segregation results in gradual changes of the optoelectronic properties of the active layer, thereby affecting the resulting performances (Gratia et al., 2016; Slotcavage et al., 2016; Barker et al., 2017; Samu et al., 2017; Yoon et al., 2017).
Therefore, there has been an ongoing effort to suppress ion migration in the perovskite materials and optoelectronic devices, which evolved into a number of strategies that are mostly based on either compositional or interface engineering (Figure 1D) (Shao and Loi, 2020), such as through molecular modulation (i.e. passivation and using interfacial blocking layers) (Milić et al., 2019b; Zhang et al., 2019), partially substituting ions (Ferdani et al., 2019; Gangishetty et al., 2019; Rybin et al., 2020), as well as using low-dimensional hybrid perovskites that suppress ion migration (Rudd and Huang, 2019). In the case of interfacial engineering, using molecular assemblies (Bai et al., 2019; Milić et al., 2019b; Zhang et al., 2019) and graphene composites (Arora et al., 2017; Milić et al., 2018) at the interface of charge selective transport layers was found to be particularly effective in stabilizing the perovskite devices (Ehrler and Hutter, 2020). This is often related to blocking the ions from the neighboring layers (Domanski et al., 2016), although very few systematic investigations demonstrate this particular mode of action (Merdasa et al., 2020; Ruiz-Preciado et al., 2020). Moreover, using appropriate selective charge-selective extraction layers can suppress the (photo)redox degradation pathways (Wei et al., 2020). In addition, the partial substitution of A-site ions, such as by using guanidinium, or B-site ions, such as via manganese, have shown promising increases in stabilities by suppressing ion migration (Ferdani et al., 2019; Futscher et al., 2020a). The reduction of ion migration may be related to local distortion leading to a lattice compression, which has recently shown to increase the activation energy for ion migration, enabling strain engineering (Hutter et al., 2020). Moreover, low-dimensional perovskites and their analogs (Connor et al., 2018; Milić et al., 2019a; Li Y. et al., 2019; Umeyama et al., 2020) were also found to be effective in suppressing ion migration due to the ion-impermeable organic spacer layers (Lin et al., 2017; Rudd and Huang, 2019). In this regard, in-depth analysis is required to elucidate the mechanisms and establish structure-property relationships that define guidelines for suitable molecular design toward addressing the challenges of controlling ion migration in various hybrid perovskite compositions. This further involves understanding the underlying polaronic effects on the properties of various compositions (Zhou et al., 2019; Meggiolaro et al., 2020) and dimensionalities (Srimath Kandada and Silva, 2020). Moreover, since the reported lifetimes of hybrid perovskite devices vary due to different assessment procedures, rigorous stability testing protocols are being established (Khenkin et al., 2020) that allow for a critical assessment of the operating conditions.
Utilization
While ion migration can be detrimental in the case of solar cells and light-emitting diodes, the mixed ionic-electronic nature of hybrid perovskites opens up possibilities for other emerging applications, such as in resistive switches and batteries (Tress, 2017; Zhao et al., 2019; Tress, 2017; Zhao et al., 2019). In particular, ion migration enable switching devices from a high resistive state (HRS) to a low resistive state (LRS; Figures 2A–D) (Zhu et al., 2017; Ma et al., 2020). These resistive switches enable the fabrication of ‘memristors’, which allows for simultaneous storage and processing of information with low energy consumption and high computing power, similar to the human brain. Such a response resembles biological synapses with low energy consumption that can be used to develop novel nonvolatile neuromorphic computing systems (Ma et al., 2020). We recognize the ongoing debate on the appropriate distinction between resistive switches and memristors that is beyond the scope of this article.
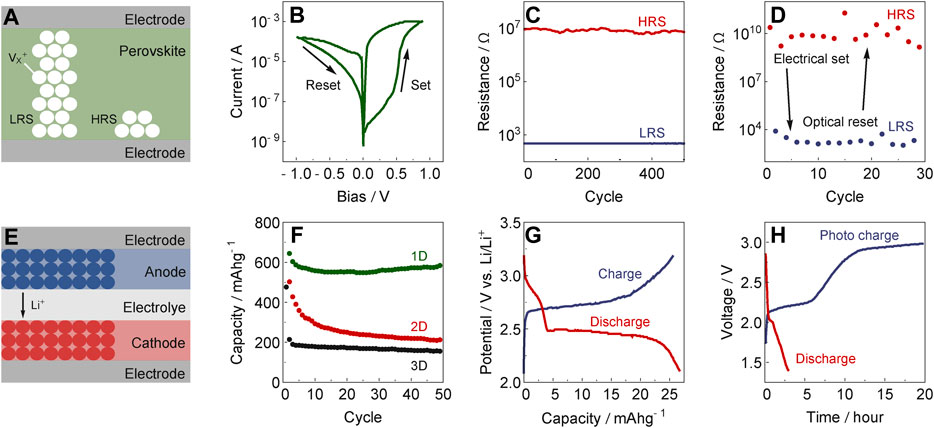
FIGURE 2. Hybrid halide perovskites as (A–D) resistive switches and (E–H) rechargeable batteries. (A) Schematic representation of low resistance states (LRS) and high resistance states (HRS) as a result of the presence or absence of conductive filaments from the contact electrodes or contributing ion vacancies (VX+). (B) Current-voltage characteristics and (C) endurance test for 500 switching cycles of a resistive switch. Adapted from (Solanki et al., 2020). (D) Light-tunable resistance switch set by an electric pulse and reset by a light pulse. Adapted from (Zhu et al., 2017). (E) Schematic representation of a rechargeable battery. (F) Perovskite-based anode performance for rechargeable batteries based on different dimensionalities (1D, 2D and 3D). Adapted from (Tathavadekar et al., 2017). (G) Charge–discharge curves of perovskite-based cathodes. Adapted from (Jaffe and Karunadasa, 2014). (H) Charge–discharge curves of perovskite-based photocathode. Adapted from (Ahmad et al., 2018).
A perovskite-based resistive switch from MAPbI3-xClx has been demonstrated for the first time in 2015, which could be switched for more than 100 cycles with a ratio of HRS/LRS of 4 (Yoo et al., 2015). This performance has considerably improved since then to an HRS/LRS ratio of up to 107, stability of more than 103 cycles, low power consumption in the order of pW, and a retention time up to 105 s (Tian et al., 2017; Zhao et al., 2019). The mechanism that dominates such a ‘memory’ behavior, is intensely debated and appears to be strongly dependent on the composition. For example, in the case of MAPbBr3 the change in resistance was found to be continuous with the applied voltage, whereas for MAPbI3 it is abrupt (Guan et al., 2018). Both these changes can be attributed to a common underlying process that is the migration of mobile ions due to the application of an external electric bias (Kim et al., 2019). This increase of ion density at the interface leads to a linear change in charge-carrier injection barrier with the applied voltage that is measured (Xiao and Huang, 2016). In addition, chemical reactions of the ions from the (e.g., metal) contacts can also lead to a continuous change in resistance with applied bias (Solanki et al., 2020). In contrast to these continuous changes, abrupt resistance changes can occur due to the formation of conductive filaments of halide vacancies or metal ions within the perovskite (Figure 2A) (Zhu et al., 2017; Sun et al., 2018). Another possible reason that can lead to ‘memristive’ behavior, which has to the best of our knowledge not yet been discussed in hybrid perovskites, is the formation of ferroelectric tunneling barriers (Chanthbouala et al., 2012) that remain to be explored. Moreover, light illumination can further suppress and even reverse the resistive switching behavior due to a reduced halide migration barrier (Figure 2D) (Zhu et al., 2017; Zhao et al., 2020). This opens the possibility of devices that can be set, reset, modified, and read out using a combination of electrical and optical stimuli. This light-tunable synaptic effect allows perovskite-based memristors to mimic the behavior of many biological systems, such as those of the dopamine-mediated synaptic activity (Ham et al., 2019).
In addition, as other ions (such as Li+) can diffuse within hybrid perovskites, they can also be used in rechargeable batteries. In a Li-ion battery, energy is stored and recovered by moving Li+ ions between a cathode and an anode via an electrolyte (Figure 2E). The use of MAPbI3 and MAPbBr3 as anodes in Li-ion batteries was demonstrated for the first time in 2015 (Xia et al., 2015). It was found that MAPbBr3 has a much higher capacity (more than 200 mAhg−1) than MAPbI3 (44 mAhg−1). This shows that composition plays an important role in storage performance. While it was initially assumed that Li+ ions intercalate into MAPbBr3 (Vicente and Garcia-Belmonte, 2017), it was later found that a conversion reaction with Li+ is most likely responsible for the observed capacity (Dawson et al., 2017). The capacity of perovskite-based anodes can be further improved by reducing the dimensionality from 3D to 2D and from 2D to 1D, due to the better accessibility of Li+ ions to Pb2+ sites to reversibly form LixPb alloys (Figure 2F) (Ramirez et al., 2018). For 1D C6H9I3NOPb perovskites, capacities of 453 mAhg−1 at 500 mAg−1 were demonstrated for up to 250 cycles, higher than the theoretical capacity of graphite (372 mAhg−1) (Tathavadekar et al., 2017). Besides anodes, perovskites can also be used as cathodes (Smith et al., 2017), which was first demonstrated in 2014 with an open-circuit voltage of 3.2 V using a lead-free 2D halide perovskite (Figure 2G) (Jaffe and Karunadasa, 2014). One particularly interesting feature is that perovskites can act as photoactive electrodes and thus as a power source to drive Li+ ions to the anode under illumination, thus converting the irregular solar power into a stable power supply in rechargeable photobatteries (Figure 2H) (Ahmad et al., 2018). This opens a new path for the utility of mixed conductivity of hybrid perovskites that is yet to be exploited. To achieve this goal, a targeted control of the desired ion migration process is necessary, and the suppression of other detrimental ion migration processes that contribute to device degradation and reduce the lifetime of these devices.
Discussion
Mixed ionic-electronic conductivity of hybrid perovskites has emerged as one of the defining features of this unique class of materials (Yang et al., 2015; Tress, 2017). While this poses challenges to the intrinsic stabilities of light-emitting and photovoltaic device as a result of accelerated ion migration during operation, these properties are simultaneously gaining interest in alternative applications, such as in rechargeable batteries and resistive switches for memory elements.
In this regard, a number of strategies has been developed to address the stabilities of perovskite light-emitting diodes and solar cells, which primarily rely on interfacial and compositional engineering and the use of low-dimensional perovskites. To this end, eliminating the toxic lead toward lead-free and air-stable perovskites remains a pressing issue. Furthermore, an in-depth analysis of their operation is required to unravel the underlying processes responsible for the degradation toward understanding the structure-property relationships that could guide advanced material design. For this purpose, further development of analytical methods is needed, in particular those that can directly relate the structural and optoelectronic properties, while monitoring the changes during operation. Methods based on solid-state NMR spectroscopy (Piveteau et al., 2020) and photoluminescence mapping (Doherty et al., 2020) that can be related to the structure at the nanoscale are particularly promising. These studies should be complemented with temperature-dependent analysis as well as establishing appropriate models for the analysis of optoelectronic properties to further deepen the fundamental understanding of the coupling between electronic and ionic processes.
Such a critical analysis is further required to pave the way for realizing the potential of hybrid perovskites in rechargeable batteries and in resistive switches for neuromorphic computer systems. Low-dimensional perovskites are of particular interest in this respect, as their organic and inorganic layers can be individually adapted to optimize stability, energy storage, and ion conductivity. Since ion migration in hybrid perovskites is light-activated, ionic and electronic processes can be coupled with light, which holds great potential for light-activated artificial synapses and photobatteries. However, research on perovskite-based materials for rechargeable batteries and resistive switches is still at an early stage and further joint efforts are needed to harness their full potential. In particular, the underlying mechanisms of resistive switching remain elusive and are subject of continuous evaluations. Similarly, deciphering the function of hybrid perovskites for use as cathodes and anodes in rechargeable batteries and photobatteries stimulates ongoing investigations. Such developments may benefit from the emerging field of optoionics, which will further broaden research horizons (Senocrate et al., 2020).
These research efforts collectively promise to provide critical level of understanding of hybrid perovskites toward their applications. We envisage that mixed conductivity of hybrid halide perovskites will play a key role in the development of innovative nanotechnologies in the future.
Author Contributions
All authors listed have made a substantial, direct, and intellectual contribution to the work and approved it for publication.
Conflict of Interest
The authors declare that the research was conducted in the absence of any commercial or financial relationships that could be construed as a potential conflict of interest.
Acknowledgments
MF is supported by a Rubicon Fellowship from the Netherlands Organisation for Scientific Research (NWO). JM acknowledges the support of the Swiss National Science Foundation PRIMA grant number 193174.
References
Abdi-Jalebi, M., Andaji-Garmaroudi, Z., Cacovich, S., Stavrakas, C., Philippe, B., Richter, J. M., et al. (2018). Maximizing and stabilizing luminescence from halide perovskites with potassium passivation. Nature 555, 497–501. doi:10.1038/nature25989
Ahmad, S., George, C., Beesley, D. J., Baumberg, J. J., and De Volder, M. (2018). Photo-rechargeable organo-halide perovskite batteries. Nano Lett. 18, 1856–1862. doi:10.1021/acs.nanolett.7b05153
Akbulatov, A. F., Frolova, L. A., Dremova, N. N., Zhidkov, I., Martynenko, V. M., Tsarev, S. A., et al. (2020). Light or heat: what is killing lead halide perovskites under solar cell operation conditions? J. Phys. Chem. Lett. 11, 333–339. doi:10.1021/acs.jpclett.9b03308
Almora, O., García-Batlle, M., and Garcia-Belmonte, G. (2019). Utilization of temperature-sweeping capacitive techniques to evaluate band gap defect densities in photovoltaic perovskites. J. Phys. Chem. Lett. 10, 3661–3669. doi:10.1021/acs.jpclett.9b00601
Almora, O., Guerrero, A., and Garcia-Belmonte, G. (2016). Ionic charging by local imbalance at interfaces in hybrid lead halide perovskites. Appl. Phys. Lett. 108, 043903. doi:10.1063/1.4941033
Arora, N., Dar, M. I., Hinderhofer, A., Pellet, N., Schreiber, F., Zakeeruddin, S. M., et al. (2017). Perovskite solar cells with CuSCN hole extraction layers yield stabilized efficiencies greater than 20. Science 358, 768–771. doi:10.1126/science.aam5655
Bai, S., Da, P., Li, C., Wang, Z., Yuan, Z., Fu, F., et al. (2019). Planar perovskite solar cells with long-term stability using ionic liquid additives. Nature 571, 245–250. doi:10.1038/s41586-019-1357-2
Barker, A. J., Sadhanala, A., Deschler, F., Gandini, M., Senanayak, S. P., Pearce, P. M., et al. (2017). Defect-assisted photoinduced halide segregation in mixed-halide perovskite thin films. ACS Energy Lett. 2, 1416–1424. doi:10.1021/acsenergylett.7b00282
Bertoluzzi, L., Belisle, R. A., Bush, K. A., Cheacharoen, R., McGehee, M. D., and O’Regan, B. C. (2018). In situ measurement of electric-field screening in hysteresis-dree PTAA/FA0.83Cs0.17Pb(I0.83Br0.17)3C60 perovskite solar cells gives an ion mobility of ∼3 × 10-7 cm2. J. Am. Chem. Soc. 140, 12775–12784. doi:10.1021/jacs.8b04405
Bertoluzzi, L., Boyd, C. C., Rolston, N., Xu, J., Prasanna, R., O’Regan, B. C., et al. (2020). Mobile ion concentration measurement and open-access band diagram simulation platform for halide perovskite solar cells. Joule 4, 109–127. doi:10.1016/j.joule.2019.10.003
Birkhold, S. T., Precht, J. T., Giridharagopal, R., Eperon, G. E., Schmidt-Mende, L., and Ginger, D. S. (2018). Direct observation and quantitative analysis of mobile frenkel defects in metal halide perovskites using scanning kelvin probe microscopy. J. Phys. Chem. C 122, 12633–12639. doi:10.1021/acs.jpcc.8b03255
Boldyreva, A. G., Zhidkov, I. S., Tsarev, S., Akbulatov, A. F., Tepliakova, M. M., Fedotov, Y. S., et al. (2020). Unraveling the impact of hole transport materials on photostability of perovskite films and p-i-n solar cells. ACS Appl. Mater. Inter. 12, 19161–19173. doi:10.1021/acsami.0c01027
Brennan, M. C., Ruth, A., Kamat, P. V., and Kuno, M. (2020). Photoinduced anion segregation in mixed halide perovskites. Trends Chem. 2, 282–301. doi:10.1016/j.trechm.2020.01.010
Bruno, A., Cortecchia, D., Chin, X. Y., Fu, K., Boix, P. P., Mhaisalkar, S., et al. (2017). Temperature and electrical poling effects on ionic motion in MAPbI3 photovoltaic cells. Adv. Energy Mater. 7, 1700265. doi:10.1002/aenm.201700265
Caram, J., García-Batlle, M., Almora, O., Arce, R. D., Guerrero, A., and Garcia-Belmonte, G. (2020). Direct observation of surface polarization at hybrid perovskite/Au interfaces by dark transient experiments. Appl. Phys. Lett. 116, 183503. doi:10.1063/5.0006409
Chanthbouala, A., Garcia, V., Cherifi, R. O., Bouzehouane, K., Fusil, S., Moya, X., et al. (2012). A ferroelectric memristor. Nat. Mater. 11, 860–864. doi:10.1038/nmat3415
Choi, J., Han, J. S., Hong, K., Kim, S. Y., and Jang, H. W. (2018). Organic–inorganic hybrid halide perovskites for memories, transistors, and artificial synapses. Adv. Mater. 30, 1704002–1704021. doi:10.1002/adma.201704002
Connor, B. A., Leppert, L., Smith, M. D., Neaton, J. B., and Karunadasa, H. I. (2018). Layered halide double perovskites: dimensional reduction of Cs2AgBiBr6. J. Am. Chem. Soc. 140, 5235–5240. doi:10.1021/jacs.8b01543
Correa-Baena, J. P., Anaya, M., Lozano, G., Tress, W., Domanski, K., Saliba, M., et al. (2016). Unbroken perovskite: interplay of morphology, electro-optical properties, and ionic movement. Adv. Mater. 28, 5031–5037. doi:10.1002/adma.201600624
Dawson, J. A., Chen, H., and Saiful Islam, M. (2018). Composition screening of lithium- and sodium-rich anti-perovskites for fast-conducting solid electrolytes. J. Phys. Chem. C 122, 23978–23984. doi:10.1021/acs.jpcc.8b08208
Dawson, J. A., Naylor, A. J., Eames, C., Roberts, M., Zhang, W., Snaith, H. J., et al. (2017). Mechanisms of lithium intercalation and conversion processes in organic-inorganic halide perovskites. ACS Energy Lett. 2, 1818–1824. doi:10.1021/acsenergylett.7b00437
Doherty, T. A. S., Winchester, A. J., Macpherson, S., Johnstone, D. N., Pareek, V., Tennyson, E. M., et al. (2020). Performance-limiting nanoscale trap clusters at grain junctions in halide perovskites. Nature 580, 360–366. doi:10.1038/s41586-020-2184-1
Domanski, K., Alharbi, E. A., Hagfeldt, A., Grätzel, M., and Tress, W. (2018). Systematic investigation of the impact of operation conditions on the degradation behaviour of perovskite solar cells. Nat. Energy. 3, 61–67. doi:10.1038/s41560-017-0060-5
Domanski, K., Correa-Baena, J. P., Mine, N., Nazeeruddin, M. K., Abate, A., Saliba, M., et al. (2016). Not all that glitters is gold: metal-migration-induced degradation in perovskite solar cells. ACS Nano. 10, 6306–6314. doi:10.1021/acsnano.6b02613
Domanski, K., Roose, B., Matsui, T., Saliba, M., Turren-Cruz, S.-H., Correa-Baena, J.-P., et al. (2017). Migration of cations induces reversible performance losses over day/night cycling in perovskite solar cells. Energy Environ. Sci. 10, 604–613. doi:10.1039/C6EE03352K
Duijnstee, A., Le Corre, V. M., Johnston, M. B., Koster, L. J. A., Lim, J., and Snaith, H. J. (2021). Understanding dark current-voltage characteristics in metal-halide perovskite single crystals. Phys. Rev. Applied 15, 014006. doi:10.1103/PhysRevApplied.15.014006
Eames, C., Frost, J. M., Barnes, P. R., O'Regan, B. C., Walsh, A., and Islam, M. S. (2015). Ionic transport in hybrid lead iodide perovskite solar cells. Nat. Commun. 6, 7497. doi:10.1038/ncomms8497
Ehrler, B., Alarcón-Lladó, E., Tabernig, S. W., Veeken, T., Garnett, E. C., and Polman, A. (2020). Photovoltaics reaching for the Shockley-Queisser limit. ACS Energy Lett. 5, 3029–3033. doi:10.1021/acsenergylett.0c01790
Ehrler, B., and Hutter, E. M. (2020). Routes toward long-term stability of mixed-halide perovskites. Matter 2, 800–802. doi:10.1016/j.matt.2020.03.005
Ferdani, D. W., Pering, S. R., Ghosh, D., Kubiak, P., Walker, A. B., Lewis, S. E., et al. (2019). Partial cation substitution reduces iodide ion transport in lead iodide perovskite solar cells. Energy Environ. Sci. 12, 2264–2272. doi:10.1039/C9EE00476A
Futscher, M. H., Gangishetty, M. K., Congreve, D. N., and Ehrler, B. (2020a). Manganese doping stabilizes perovskite light-emitting diodes by reducing ion migration. ACS Appl. Electron. Mater. 2, 1522–1528. doi:10.1021/acsaelm.0c00125
Futscher, M. H., Gangishetty, M. K., Congreve, D. N., and Ehrler, B. (2020b). Quantifying mobile ions in perovskite-based devices with temperature-dependent capacitance measurements: frequency versus time domain. J. Chem. Phys. 152, 044202. doi:10.1063/1.5132754
Futscher, M. H., Lee, J. M., McGovern, L., Muscarella, L. A., Wang, T., Haider, M. I., et al. (2019). Quantification of ion migration in CH3NH3PbI3 perovskite solar cells by transient capacitance measurements. Mater. Horizons. 6, 1497–1503. doi:10.1039/c9mh00445a
Gangishetty, M. K., Sanders, S. N., and Congreve, D. N. (2019). Mn2+ doping enhances the brightness, efficiency, and stability of bulk perovskite light-emitting diodes. ACS Photon. 6, 1111–1117. doi:10.1021/acsphotonics.9b00142
Grätzel, M. (2017). The rise of highly efficient and stable perovskite solar cells. Acc. Chem. Res. 50, 487–491. doi:10.1021/acs.accounts.6b00492
Graetzel, M., and Milić, J. V. (2019). The advent of molecular photovoltaics and hybrid perovskite solar cells. Substantia 3, 27–43. doi:10.13128/Substantia-697
Grancini, G., and Nazeeruddin, M. K. (2019). Dimensional tailoring of hybrid perovskites for photovoltaics. Nat. Rev. Mater. 4, 4–22. doi:10.1038/s41578-018-0065-0
Gratia, P., Grancini, G., Audinot, J. N., Jeanbourquin, X., Mosconi, E., Zimmermann, I., et al. (2016). Intrinsic halide segregation at nanometer scale determines the high efficiency of mixed cation/mixed halide perovskite solar cells. J. Am. Chem. Soc. 138, 15821–15824. doi:10.1021/jacs.6b10049
Guan, X., Hu, W., Haque, M. A., Wei, N., Liu, Z., Chen, A., et al. (2018). Light-responsive ion-redistribution-induced resistive switching in hybrid perovskite Schottky junctions. Adv. Funct. Mater. 28, 1704665. doi:10.1002/adfm.201704665
Ham, S., Choi, S., Cho, H., Na, S. I., and Wang, G. (2019). Photonic organolead halide perovskite artificial synapse capable of accelerated learning at low power inspired by dopamine-facilitated synaptic activity. Adv. Funct. Mater. 29, 1806646. doi:10.1002/adfm.201806646
Hutter, E. M., Muscarella, L. A., Wittmann, F., Versluis, J., McGovern, L., Bakker, H. J., et al. (2020). Thermodynamic stabilization of mixed-halide perovskites against phase segregation. Cell Rep. Phys. Sci. 1, 100120. doi:10.1016/j.xcrp.2020.100120
Jaffe, A., and Karunadasa, H. I. (2014). Lithium cycling in a self-assembled copper chloride-polyether hybrid electrode. Inorg. Chem. 53, 6494–6496. doi:10.1021/ic500860t
Jena, A. K., Kulkarni, A., and Miyasaka, T. (2019). Halide perovskite photovoltaics: background, status, and future prospects. Chem. Rev. 119, 3036–3103. doi:10.1021/acs.chemrev.8b00539
Katan, C., Mohite, A. D., and Even, J. (2018). Entropy in halide perovskites. Nat. Mater. 17, 377–379. doi:10.1038/s41563-018-0070-0
Khenkin, M. V., Katz, E. A., Abate, A., Bardizza, G., Berry, J. J., Brabec, C., et al. (2020). Consensus statement for stability assessment and reporting for perovskite photovoltaics based on ISOS procedures. Nat. Energy. 5, 35–49. doi:10.1038/s41560-019-0529-5
Kim, G. W., and Petrozza, A. (2020). Defect tolerance and intolerance in metal-halide perovskites. Adv. Energy Mater. 10, 2001959. doi:10.1002/aenm.202001959
Kim, G. Y., Senocrate, A., Wang, Y., Moia, D., and Maier, J. (2020). Photo‐effect onion transport in mixed cation and halide perovskites and implications for photo demixing. Angew. Chem. Int. Ed. 60, 820–826. doi:10.1002/anie.202005853
Kim, G. Y., Senocrate, A., Yang, T. Y., Gregori, G., Grätzel, M., and Maier, J. (2018). Large tunable photoeffect on ion conduction in halide perovskites and implications for photodecomposition. Nat. Mater. 17, 445–449. doi:10.1038/s41563-018-0038-0
Kim, H., Han, J. S., Kim, S. G., Kim, S. Y., and Jang, H. W. (2019). Halide perovskites for resistive random-access memories. J. Mater. Chem. C 7, 5226–5234. doi:10.1039/C8TC06031B
Kim, H. S., Lee, C. R., Im, J. H., Lee, K. B., Moehl, T., Marchioro, A., et al. . (2012). Lead iodide perovskite sensitized all-solid-state submicron thin film mesoscopic solar cell with efficiency exceeding 9%. Sci. Rep., 2, 591. doi:10.1038/srep00591
Lee, M. M., Teuscher, J., Miyasaka, T., Murakami, T. N., and Snaith, H. J. (2012). Efficient hybrid solar cells based on meso-superstructured organometal halide perovskites. Science 338, 643–647. doi:10.1126/science.1228604
Lei, L., Seyitliyev, D., Stuard, S., Mendes, J., Dong, Q., Fu, X., et al. (2020). Efficient energy funneling in quasi-2D perovskites: from light emission to lasing. Adv. Mater. 32, 1906571–1906579. doi:10.1002/adma.201906571
Li, X., Liang, J., Yang, X., Adair, K. R., Wang, C., Zhao, F., et al. (2020). Progress and perspectives on halide lithium conductors for all-solid-state lithium batteries. Energy Environ. Sci. 13, 1429–1461. doi:10.1039/c9ee03828k
Li, Y., Milić, J. V., Ummadisingu, A., Seo, J. Y., Im, J. H., Kim, H. S., et al. (2019). Bifunctional organic spacers for formamidinium-based hybrid Dion-Jacobson two-dimensional perovskite solar cells. Nano Lett. 19, 150–157. doi:10.1021/acs.nanolett.8b03552
Li, Y. T., Ding, L., Li, J. Z., Kang, J., Li, D. H., Ren, L., et al. (2019). Light-enhanced ion migration in two-dimensional perovskite single crystals revealed in carbon nanotubes/two-dimensional perovskite heterostructure and its photomemory application. ACS Cent. Sci. 5, 1857–1865. doi:10.1021/acscentsci.9b00839
Lin, K., Xing, J., Quan, L. N., de Arquer, F. P. G., Gong, X., Lu, J., et al. (2018). Perovskite light-emitting diodes with external quantum efficiency exceeding 20 per cent. Nature 562, 245–248. doi:10.1038/s41586-018-0575-3
Lin, Y., Bai, Y., Fang, Y., Wang, Q., Deng, Y., and Huang, J. (2017). Suppressed ion migration in low-dimensional perovskites. ACS Energy Lett. 2, 1571–1572. doi:10.1021/acsenergylett.7b00442
Lv, Z., Wang, Y., Chen, J., Wang, J., Zhou, Y., and Han, S. T. (2020). Semiconductor quantum dots for memories and neuromorphic computing systems. Chem. Rev. 120, 3941–4006. doi:10.1021/acs.chemrev.9b00730
Ma, F., Zhu, Y., Xu, Z., Liu, Y., Zheng, X., Ju, S., et al. (2020). Optoelectronic perovskite synapses for neuromorphic computing. Adv. Funct. Mater. 30, 1908901. doi:10.1002/adfm.201908901
Magomedov, A., Kasparavičius, E., Rakstys, K., Paek, S., Gasilova, N., Genevičius, K., et al. (2018). Pyridination of hole transporting material in perovskite solar cells questions the long-term stability. J. Mater. Chem. C 6, 8874–8878. doi:10.1039/c8tc02242a
Mao, L., Stoumpos, C. C., and Kanatzidis, M. G. (2019). Two-dimensional hybrid halide perovskites: principles and promises. J. Am. Chem. Soc. 141, 1171–1190. doi:10.1021/jacs.8b10851
McGovern, L., Futscher, M. H., Muscarella, L. A., and Ehrler, B. (2020). Understanding the stability of MAPbBr3 versus MAPbI3: suppression of methylammonium migration and reduction of halide migration. J. Phys. Chem. Lett. 11, 7127–7132. doi:10.1021/acs.jpclett.0c01822
Meggiolaro, D., Ambrosio, F., Mosconi, E., Mahata, A., and De Angelis, F. (2020). Polarons in metal halide perovskites. Adv. Energy Mater. 10, 1902715–1902748. doi:10.1002/aenm.201902748
Meggiolaro, D., Mosconi, E., and De Angelis, F. (2019). Formation of surface defects dominates ion migration in lead-halide perovskites. ACS Energy Lett. 4, 779–785. doi:10.1021/acsenergylett.9b00247
Merdasa, A., Tsarev, S., Akbulatov, A. F., Troshin, P., and Unger, E. L. (2020). Microscopic insight into the reversibility of photodegradation in MAPbI3 thin films. J. Lumin. 219, 116916. doi:10.1016/j.jlumin.2019.116916
Milić, J. V., Kubicki, D. J., Emsley, L., and Grätzel, M. (2019b). Multifunctional molecular modulation for efficient and stable hybrid perovskite solar cells. Chimia 73, 317–323. doi:10.2533/chimia.2019.317
Milić, J. V., Arora, N., Dar, M. I., Zakeeruddin, S. M., and Grätzel, M. (2018). Reduced graphene oxide as a stabilizing agent in perovskite solar cells. Adv. Mater. Inter. 5, 1800416–1800418. doi:10.1002/admi.201800416
Milić, J. V., Im, J. H., Kubicki, D. J., Ummadisingu, A., Seo, J. Y., Li, Y., et al. (2019a). Supramolecular engineering for formamidinium based layered 2D perovskite solar cells: structural complexity and dynamics revealed by solid-state NMR spectroscopy. Adv. Energy Mater. 9, 1900284. doi:10.1002/aenm.201900284
Mizusaki, J., Arai, K., and Fueki, K. (1983). Ionic conduction of the perovskite-type halides. Solid State Ionics 11, 203–211. doi:10.1016/0167-2738(83)90025-5
Moia, D., Gelmetti, I., Calado, P., Fisher, W., Stringer, M., Game, O., et al. (2019). Ionic-to-electronic current amplification in hybrid perovskite solar cells: ionically gated transistor-interface circuit model explains hysteresis and impedance of mixed conducting devices. Energy Environ. Sci. 12, 1296–1308. doi:10.1039/c8ee02362j
Motti, S. G., Meggiolaro, D., Barker, A. J., Mosconi, E., Perini, C. A. R., Ball, J. M., et al. (2019). Controlling competing photochemical reactions stabilizes perovskite solar cells. Nat. Photon. 13, 532–539. doi:10.1038/s41566-019-0435-1
Muscarella, L. A., Hutter, E. M., Wittmann, F., Woo, Y. W., Jung, Y. K., McGovern, L., et al. (2020). Lattice compression increases the activation barrier for phase segregation in mixed-halide perovskites. ACS Energy Lett. 5, 3152–3158. doi:10.1021/acsenergylett.0c01474
Neukirch, A. J., Nie, W., Blancon, J. C., Appavoo, K., Tsai, H., Sfeir, M. Y., et al. (2016). Polaron stabilization by cooperative lattice distortion and cation rotations in hybrid perovskite materials. Nano Lett. 16, 3809–3816. doi:10.1021/acs.nanolett.6b01218
Piveteau, L., Morad, V., and Kovalenko, M. V. (2020). Solid-state NMR and NQR spectroscopy of lead-halide perovskite materials. J. Am. Chem. Soc. 142, 19413–19437. doi:10.1021/jacs.0c07338
Ramirez, D., Suto, Y., Rosero-Navarro, N. C., Miura, A., Tadanaga, K., and Jaramillo, F. (2018). Structural and electrochemical evaluation of three- and two-dimensional organohalide perovskites and their influence on the reversibility of lithium intercalation. Inorg. Chem. 57, 4181–4188. doi:10.1021/acs.inorgchem.8b00397
Reichert, S., An, Q., Woo, Y. W., Walsh, A., Vaynzof, Y., and Deibel, C. (2020a). Probing the ionic defect landscape in halide perovskite solar cells. Nat. Commun. 11, 6098. doi:10.1038/s41467-020-19769-8
Reichert, S., Flemming, J., An, Q., Vaynzof, Y., Pietschmann, J. F., and Deibel, C. (2020b). Ionic-defect distribution revealed by improved evaluation of deep-level transient spectroscopy on perovskite solar cells. Phys. Rev. Appl. 13, 034018. doi:10.1103/PhysRevApplied.13.034018
Rong, Y., Hu, Y., Mei, A., Tan, H., Saidaminov, M. I., et al. et al. (2018). Challenges for commercializing perovskite solar cells. Science 361, eaat8235. doi:10.1126/science.aat8235
Rudd, P. N., and Huang, J. (2019). Metal ions in halide perovskite materials and devices. Trends Chem. 1, 394–409. doi:10.1016/j.trechm.2019.04.010
Ruiz-Preciado, M. A., Kubicki, D. J., Hofstetter, A., McGovern, L., Futscher, M. H., Ummadisingu, A., et al. (2020). Supramolecular modulation of hybrid perovskite solar cells via bifunctional halogen bonding revealed by two-dimensional 19F solid-state nmr spectroscopy. J. Am. Chem. Soc. 142, 1645–1654. doi:10.1021/jacs.9b13701
Rybin, N., Ghosh, D., Tisdale, J., Shrestha, S., Yoho, M., Vo, D., et al. (2020). Effects of chlorine mixing on optoelectronics, ion migration, and gamma-ray detection in bromide perovskites. Chem. Mater. 32, 1854–1863. doi:10.1021/acs.chemmater.9b04244
Samu, G. F., Janáky, C., and Kamat, P. V. (2017). A victim of halide ion segregation. How light soaking affects solar cell performance of mixed halide lead perovskites. ACS Energy Lett. 2, 1860–1861. doi:10.1021/acsenergylett.7b00589
Senocrate, A., Kotomin, E., and Maier, J. (2020). On the way to optoionics. Helv. Chim. Acta. 103, 268. doi:10.1002/hlca.202000073
Senocrate, A., Moudrakovski, I., Kim, G. Y., Yang, T.-Y., Gregori, G., Grätzel, M., et al. (2017). The nature of ion conduction in methylammonium lead iodide: a multimethod approach. Angew. Chem. Int. Ed. 56, 7755–7759. doi:10.1002/anie.201701724
Shao, S., and Loi, M. A. (2020). The role of the interfaces in perovskite solar cells. Adv. Mater. Inter. 7, 1901469. doi:10.1002/admi.201901469
Slotcavage, D. J., Karunadasa, H. I., and McGehee, M. D. (2016). Light-induced phase segregation in halide-perovskite absorbers. ACS Energy Lett. 1, 1199–1205. doi:10.1021/acsenergylett.6b00495
Smith, I. C., Smith, M. D., Jaffe, A., Lin, Y., and Karunadasa, H. I. (2017). Between the sheets: postsynthetic transformations in hybrid perovskites. Chem. Mater. 29, 1868–1884. doi:10.1021/acs.chemmater.6b05395
Smith, M. D., Connor, B. A., and Karunadasa, H. I. (2019). Tuning the luminescence of layered halide perovskites. Chem. Rev. 119, 3104–3139. doi:10.1021/acs.chemrev.8b00477
Snaith, H. J. (2018). Present status and future prospects of perovskite photovoltaics. Nat. Mater. 17, 372–376. doi:10.1038/s41563-018-0071-z
Solanki, A., Guerrero, A., Zhang, Q., Bisquert, J., and Sum, T. C. (2020). Interfacial mechanism for efficient resistive switching in Ruddlesden-Popper perovskites for non-volatile memories. J. Phys. Chem. Lett. 11, 463–470. doi:10.1021/acs.jpclett.9b03181
Srimath Kandada, S. A. R., and Silva, C. (2020). Exciton polarons in two-dimensional hybrid metal-halide perovskites. J. Phys. Chem. Lett. 11, 3173–3184. doi:10.1021/acs.jpclett.9b02342
Stranks, S. D. (2017). Nonradiative losses in metal halide perovskites. ACS Energy Lett. 2, 1515–1525. doi:10.1021/acsenergylett.7b00239
Sun, Y., Tai, M., Song, C., Wang, Z., Yin, J., Li, F., et al. (2018). Competition between metallic and vacancy defect conductive filaments in a CH3NH3PbI3-based memory device. J. Phys. Chem. C 122, 6431–6436. doi:10.1021/acs.jpcc.7b12817
Tathavadekar, M., Krishnamurthy, S., Banerjee, A., Nagane, S., Gawli, Y., Suryawanshi, A., et al. (2017). Low-dimensional hybrid perovskites as high performance anodes for alkali-ion batteries. J. Mater. Chem. A. 5, 8634–18642. doi:10.1039/c7ta04529h
Tian, H., Zhao, L., Wang, X., Yeh, Y. W., Yao, N., Rand, B. P., et al. (2017). Extremely low operating current resistive memory based on exfoliated 2D perovskite single crystals for neuromorphic computing. ACS Nano. 11, 12247–12256. doi:10.1021/acsnano.7b05726
Tress, W. (2017). Metal halide perovskites as mixed electronic-ionic conductors: challenges and opportunities-from hysteresis to memristivity. J. Phys. Chem. Lett. 8, 3106–3114. doi:10.1021/acs.jpclett.7b00975
Tress, W., Domanski, K., Carlsen, B., Agarwalla, A., Alharbi, E. A., Graetzel, M., et al. (2019). Performance of perovskite solar cells under simulated temperature-illumination real-world operating conditions. Nat. Energy. 4, 568–574. doi:10.1038/s41560-019-0400-8
Umeyama, D., Leppert, L., Connor, B. A., Manumpil, M. A., Neaton, J. B., and Karunadasa, H. I. (2020). Expanded analogs of three-dimensional lead-halide hybrid perovskites. Angew. Chem. Int. Ed. 59, 19087–19094. doi:10.1002/anie.202005012
Vicente, N., and Garcia-Belmonte, G. (2017). Methylammonium lead bromide perovskite battery anodes reversibly host high Li-ion concentrations. J. Phys. Chem. Lett. 8, 1371–1374. doi:10.1021/acs.jpclett.7b00189
Walsh, A., and Stranks, S. D. (2018). Taking control of ion transport in halide perovskite solar cells. ACS Energy Lett. 3, 1983–1990. doi:10.1021/acsenergylett.8b00764
Wang, H., Guerrero, A., Bou, A., Al-Mayouf, A. M., and Bisquert, J. (2019). Kinetic and material properties of interfaces governing slow response and long timescale phenomena in perovskite solar cells. Energy Environ. Sci. 12, 2054–2079. doi:10.1039/C9EE00802K
Wang, R., Mujahid, M., Duan, Y., Wang, Z. K., Xue, J., and Yang, Y. (2019). A review of perovskites solar cell stability. Adv. Funct. Mater. 29, 1808843. doi:10.1002/adfm.201808843
Weber, S. A. L., Hermes, I. M., Turren-Cruz, S.-H., Gort, C., Bergmann, V. W., Gilson, L., et al. (2018). How the formation of interfacial charge causes hysteresis in perovskite solar cells. Energy Environ. Sci. 11, 2404–2413. doi:10.1039/C8EE01447G
Wei, J., Wang, Q., Huo, J., Gao, F., Gan, Z., Zhao, Q., et al. (2020). Mechanisms and suppression of photoinduced degradation in perovskite solar cells. Adv. Energy Mater. 11, 2002326. doi:10.1002/aenm.202002326
Xia, H. R., Sun, W. T., and Peng, L. M. (2015). Hydrothermal synthesis of organometal halide perovskites for Li-ion batteries. Chem. Commun. 51, 13787–13790. doi:10.1039/c5cc05053g
Xiao, Z., and Huang, J. (2016). Energy-efficient hybrid perovskite memristors and synaptic devices. Adv. Electron. Mater. 2, 1600100. doi:10.1002/aelm.201600100
Xing, J., Wang, Q., Dong, Q., Yuan, Y., Fang, Y., and Huang, J. (2016). Ultrafast ion migration in hybrid perovskite polycrystalline thin films under light and suppression in single crystals. Phys. Chem. Chem. Phys. 18, 30484–30490. doi:10.1039/C6CP06496E
Yan, Z., Zhai, W., Wang, F., Li, Q., Lin, L., Bao, C., et al. (2021). Reversible ionic polarization in metal halide perovskites. J. Phys. Chem. C 125, 283–289. doi:10.1021/acs.jpcc.0c10416
Yang, T. Y., Gregori, G., Pellet, N., Grätzel, M., and Maier, J. (2015). The significance of ion conduction in a hybrid organic-inorganic lead-iodide-based perovskite photosensitizer. Angew. Chem. Int. Ed. Engl. 54, 7905–7910. doi:10.1002/anie.201500014
Yoo, E. J., Lyu, M., Yun, J. H., Kang, C. J., Choi, Y. J., and Wang, L. (2015). Resistive switching behavior in organic-inorganic hybrid CH3NH3PbI3-xClx perovskite for resistive random access memory devices. Adv. Mater. 27, 6170–6175. doi:10.1002/adma.201502889
Yoon, S. J., Kuno, M., and Kamat, P. V. (2017). Shift happens. How halide ion defects influence photoinduced segregation in mixed halide perovskites. ACS Energy Lett. 2, 1507–1514. doi:10.1021/acsenergylett.7b00357
Yuan, Y., Chae, J., Shao, Y., Wang, Q., Xiao, Z., Centrone, A., et al. (2015). Photovoltaic switching mechanism in lateral structure hybrid perovskite solar cells. Adv. Energy Mater. 5, 1500615. doi:10.1002/aenm.201500615
Zhang, H., Nazeeruddin, M. K., and Choy, W. C. H. (2019). Perovskite photovoltaics: the significant role of ligands in film formation, passivation, and stability. Adv. Mater. 2, 1805702. doi:10.1002/adma.201805702
Zhao, X., Wang, Z., Li, W., Sun, S., Xu, H., Zhou, P., et al. (2020). Photoassisted electroforming method for reliable low-power organic–inorganic perovskite memristors. Adv. Funct. Mater. 30, 1910151. doi:10.1002/adfm.201910151
Zhao, X., Xu, H., Wang, Z., Lin, Y., and Liu, Y. (2019). Memristors with organic-inorganic halide perovskites. InfoMat 1, 183–210. doi:10.1002/inf2.12012
Zhidkov, I. S., Boukhvalov, D. W., Kukharenko, A. I., Finkelstein, L. D., Cholakh, S. O., Akbulatov, A. F., et al. (2020). Influence of ion migration from ITO and SiO3 substrates on photo and thermal stability of CH3NH3SnI3 hybrid perovskite. J. Phys. Chem. C 124, 14928–14934. doi:10.1021/acs.jpcc.0c04621
Zhou, L., Katan, C., Nie, W., Tsai, H., Pedesseau, L., Crochet, J. J., et al. (2019). Cation alloying delocalizes polarons in lead halide perovskites. J. Phys. Chem. Lett. 10, 3516–3524. doi:10.1021/acs.jpclett.9b01077
Zhou, Y., You, L., Wang, S., Ku, Z., Fan, H., Schmidt, D., et al. (2016). Giant photostriction in organic-inorganic lead halide perovskites. Nat. Commun., 7, 11193. doi:10.1038/ncomms11193
Zhu, X., Lee, J., and Lu, W. D. (2017). Iodine vacancy redistribution in organic-inorganic halide perovskite films and resistive switching effects. Adv. Mater. 29, 1700527. doi:10.1002/adma.201700527
Keywords: mixed ionic-electronic conductivity, hybrid perovskites, ionics, photovoltaics, LEDs, batteries, resistive switches, memristors
Citation: Futscher MH and Milić JV (2021) Mixed Conductivity of Hybrid Halide Perovskites: Emerging Opportunities and Challenges. Front. Energy Res. 9:629074. doi: 10.3389/fenrg.2021.629074
Received: 13 November 2020; Accepted: 26 January 2021;
Published: 16 March 2021.
Edited by:
Monica Lira-Cantu, Catalan Institute of Nanoscience and Nanotechnology (CIN2), SpainReviewed by:
Yana Vaynzof, Heidelberg University, GermanyHo Won Jang, Seoul National University, South Korea
Masoud Karimipour, Uppsala University, Sweden
Annalisa Bruno, Energy and Sustainable Economic Development (ENEA), Italy
Chang-Qi Ma, Suzhou Institute of Nano-tech and Nano-bionics (CAS), China
Copyright © 2021 Futscher and Milić. This is an open-access article distributed under the terms of the Creative Commons Attribution License (CC BY). The use, distribution or reproduction in other forums is permitted, provided the original author(s) and the copyright owner(s) are credited and that the original publication in this journal is cited, in accordance with accepted academic practice. No use, distribution or reproduction is permitted which does not comply with these terms.
*Correspondence: Jovana V. Milić, am92YW5hLm1pbGljQHVuaWZyLmNo; Moritz H. Futscher, bW9yaXR6LmZ1dHNjaGVyQGVtcGEuY2g=