- 1Circonica Circular Energy B.V., Leiden, Netherlands
- 2Process and Energy Department, Faculty of 3mE, Delft University of Technology, Delft, Netherlands
- 3Energy and Sustainability Research Institute Groningen, Faculty of Science and Engineering, University of Groningen, Groningen, Netherlands
Negative emission technologies have recently received increasing attention due to climate change and global warming. One among them is bioenergy with carbon capture and storage (BECCS), but the capture process is very energy intensive. Here, a novel pathway is introduced, based on second-generation biofuels followed by carbon circulation in an indefinitely closed chain, effectively resulting in a sink. Instead of using an energy-intensive conventional CCS process, the application of an on-board solid oxide fuel cell (SOFC) running on biofuels in an electric vehicle (FCEV) could result in negative emissions by capturing a concentrated stream of CO2, which is readily stored in a second tank. A CO2 recovery system at the fuel station then takes the CO2 from the tank to be transported to storage locations or to be used for local applications such as CO2-based concrete curing and synthesis of e-fuels. Incorporating CO2 utilization technologies into the FCEVs-SOFC system can close the carbon loop, achieving carbon neutrality through feeding the CO2 in a reverse-logistic to a methanol plant. The methanol produced is also used in SOFCs, leading to an infinite repetition of this carbon cycle till a saturation stage is reached. It is determined this pathway will reach typical Cradle-to-Grave negative emissions of 0.515 ton CO2 per vehicle, and total negative CO2 emission of 138 Mt for all passenger cars in the EU is potentially achievable. All steps comprise known technologies with medium to high technology readiness level (TRL) levels, so principally this system can readily be applied in the mid-term.
Introduction
There is a concern that global CO2 emission reductions will not meet targets. Measures in the field of energy efficiency, bio-based fuels, material recycling, and decarbonization may not be sufficient to reach the 1.5–2 °C targets set for global warming. Thus, additional measures need to be taken to mitigate this. Negative emission technologies (NETs) have so far been thought of as attractive and promising ways to reduce greenhouse gas (GHG) emissions. To date, various technologies have been applied for achieving negative emissions. One of the important negative emission pathways is bioenergy carbon capture and storage (BECCS). This route comprises power generation combusting biomass into energy or biomass digestion into biogas. The output, which is a dilute CO2-containing gas, requires separation by means of a CCS process, which in general comprises an amine solvent process requiring a significant amount of energy. After purification and compression, the captured CO2 is then stored subsurface (Cuccia et al., 2018). In addition to traditional amine-based CO2 capture, there are numerous CO2 capture technologies being developed in order to reduce the energy penalty accompanying the CCS process. For example, one of the options is the direct capture of CO2 from air (DAC) followed by CO2 storage; subsurface or using mineralization. However, because of low ppm CO2 levels in the air the energy efficiency is not yet high enough to bring the cost to a competitive level (Fasihi et al., 2019). CO2 mineralization is another interesting option. The attractive point of CO2 mineralization is the lack of any energy input requirement (being an exothermal reaction), but it is at an early stage of development (Kainiemi et al., 2015). The routes above are either limited by the supply of biomass to be used for energy or the high cost of CCS.
For the foregoing reasons, we propose a new route for negative emissions for transportation and distributed power applications with less of the above limitations, by using fuel cell technologies. Among a range of fuel cell types, using solid oxide fuel cells (SOFCs) for power generation and simultaneously CO2 capture is a prospective and attractive approach. SOFCs are low-emission, modular, fuel-flexible, and high electrical efficiency devices with the capability of converting diverse fuels into heat and power (Thattai et al., 2017). In the SOFC unit, the electrochemical fuel oxidation involves the automatic separation of nitrogen from oxygen, and the anode output is passed through an oxy-fuel combustion system in which the unused fuel (since fuel utilization is not 100% in SOFCs) is burned with pure oxygen (with the oxygen taken from a separate tank, and possibly coming from electrolysers), thereby producing a CO2-enriched gas. In this way, very high-purity CO2 can easily be separated by condensing water vapor (Thattai et al., 2017; Slater et al., 2019).
Proposed Technology for Negative Emissions
Bio-Carbon Circularity in Automotive and Distributed Power
The energy density of liquid fuels is superior to that of electric vehicle (EV) battery storage (Ong et al., 2017). Liquid fuels offer ultra-fast “charging” of vehicles at a conventional fuel station in the order of 10 MWchem. But EVs pose a solution for local and global emissions of carbon, fine particulate matter, and NOx. Combining the strong features of both principles would result in a powerful alternative for both low-carbon and clean transportation. It has been known that fuel cell-powered electric vehicles (FCEVs) offer such combined features (Fernandes et al., 2016). Hydrogen FCEVs offer some of the above advantages, but the distribution and fuelling of liquid fuels are more attractive and use current and inexpensive fuel infrastructure with little losses. Moreover, the current efforts to introduce new second-generation biofuels would already stagnate after 2030, with the planned exit of vehicles with combustion engines. Unlike polymer electrolyte membrane fuel cells (PEMFCs), SOFCs can directly use hydrocarbons and do not rely exclusively on purified hydrogen. The combination of biofuels with SOFCs ensures efficient and low-emission use of these biofuels, also after 2030. Nissan is a front-runner in the use of bioethanol-fed SOFC in FCEVs (Nissan Motor Corporation [NMC], 2021). The existing cradle-to-grave carbon footprint can be described with the following pathways:
(1) Fossil fuels in an internal combustion engine (ICE) or SOFC fuel cell (Figure 1A, pathway1): Fossil carbon is extracted as oil and natural gas, refined into a fossil fuel, distributed, and finally used, emitting CO2 in all steps. Being more efficient, an SOFC results in lower carbon emissions during use. In this aspect, an integration of ICE with a temperature swing adsorption (TSA) for on-board CCS in the transportation sector has been proposed (Sharma and Maréchal, 2019), where it was reported that 90% of the CO2 emitted from combustion of on-board fuels can be captured without any energy penalty. Such a system is adequately applied for train and ship transports (Sharma and Maréchal, 2019).
(2) Biofuel in an internal combustion engine (ICE) or SOFC fuel cell (Figure 1B, pathway 2): Carbon is extracted from air by biomass, refined, distributed, and used in an ICE or SOFC. Being more efficient, an SOFC results in lower carbon emissions during use. Although CO2 is emitted in the last three steps, this is mitigated by the fixation in the first step, resulting in an emission reduction compared to fossil fuel consumption (pathway 1). To achieve negative emissions or carbon circularity in the use of transportation fuels, we postulate the following solutions. While concentrated CO2 is emitted at the anode output of the SOFC, this can readily be recovered after condensation of the water vapor and stored in an on-board pressurized tank. While the vehicle is being refueled with biofuels, the CO2 is returned to a pressurized tank at the filling station. The CO2 then needs to be stored somewhere to achieve negative emissions.
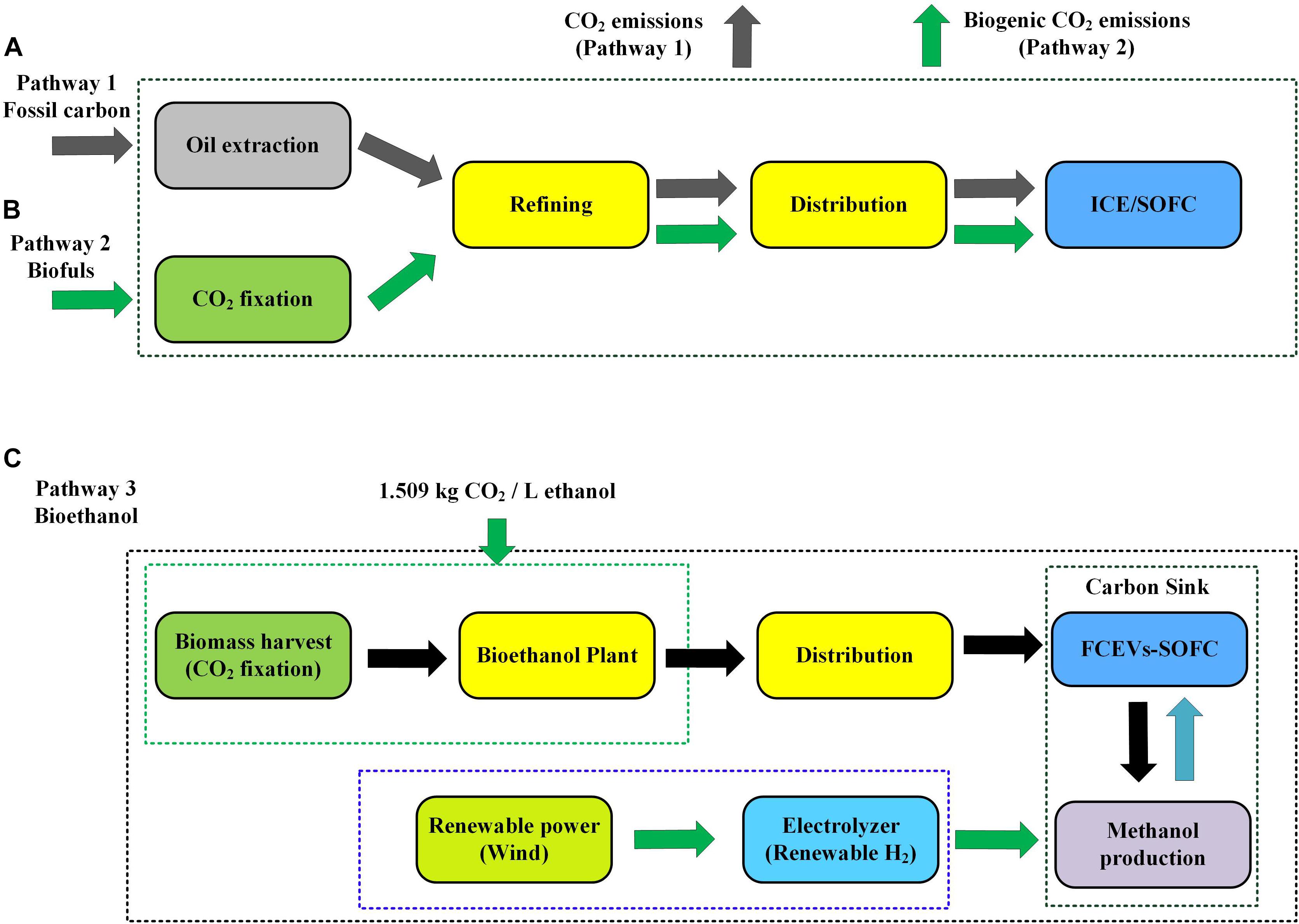
Figure 1. The various pathways of the transportation and distributed power system. (A) Pathway 1—fossil carbon. (B) Pathway 2—biofuels. (C) Pathway 3—bioethanol.
Incorporating CO2 Utilization Into the FCEVs-SOFC for Negative Emissions
The principle of negative emissions is shown in the following pathway:
(3) Biofuels in an SOFC with carbon recycle (Figure 1C, pathway 3): Carbon is extracted from air by biomass, refined, distributed, and used in an SOFC. Now, the carbon is not emitted, but separated, compressed, returned to a methanol plant, converted into methanol-based fuel, and redistributed for use in the SOFC with an infinite repetition of this carbon cycle. Here, an e-fuel like methanol or dimethyl ether (DME) is synthesized using renewable hydrogen from an electrolyzer powered by wind or solar energy. This produced e-fuel contains carbon from a biogenic origin from the bioethanol and it can then be used as a fuel in FCEVs with SOFC. The overall concept of a negative emission system is depicted in Figure 1C including the following steps: (1) uptake of CO2 from the atmosphere by lignocellulosic biomass; (2) conversion of lignocellulosic biomass into bioethanol; (3) power generation in on-board SOFC charging FCEV batteries; (4) recovery of CO2 for return to a methanol plant; (5) hydrogen electrolysis fed by renewable power sources; and (6) conversion of returned CO2 into methanol using renewable hydrogen. This cycle (steps 3–6) can be repeated infinitely, resulting in an artificial sink for CO2 within the cars, methanol plant, and associated components.
Here, a cradle-to-grave system boundary is taken into account to address the total GHG emissions of the proposed system. Each liter of ethanol is equivalent to 1.509 kg CO2, which is captured by biomass. Using the ethanol in FCEVs-SOFC results in a concentrated CO2 stream. The concentrated CO2, and H2 from electrolysis are used as a feedstock to synthesize methanol (which can be further recycled to SOFC-EVs). Renewable methanol production (including electrolysis) can be carried out with around 50% efficiency according to literature (Bos et al., 2020), consuming a large amount of energy [45.4 MJ/kg-MeOH with higher heating value (HHV)-basis (Richards and Coley, 2005), or around 33.0 MJ/kg-CO2]. But the methanol production and future transport processes of biomass and fuels are all assumed to be powered by renewable H2, while a next-generation bioethanol refinery is assumed to utilize its own biogenic by-products (e.g., lignin). Thus all these processes are assumed to be carbon neutral. The energy cost of CO2 compression is also low, and can be accounted for in the tank-to-wheel (TTW) efficiency of an SOFC car, as shown in the notes of Table 1. With these assumptions, preliminary indicative calculations are made for the total negative emissions. These indicative calculations are shown in Table 1 and its notes. However, more detailed calculations will be required for feasibility assessment or design to be carried out in future.
The proposed pathway can attain typical negative CO2 emissions of 1.509 kg CO2 per liter of bioethanol consumed. Assuming there is a lag time of 1 year between the CO2 from a car being captured and being provided to a car again as methanol, maximum negative emission of 0.515 ton CO2 is achievable for each SOFC-equipped car that uses bioethanol with CCU (see Table 1 and its notes for calculations). This means possible total negative CO2 emissions of 138 Mt for all passenger cars in the EU. A single bioethanol plant producing 80 kt ethanol annually can eliminate fossil fuel emissions from 9.35 million Dutch passenger cars in just under 32 years, capturing 4.82 Mt CO2 in that time. This quantity of CO2 can be held in this artificial sink indefinitely, as long as these cars are in operation. It no longer causes negative emissions after being saturated in 32 years but after this, production of sustainable polymers (via methanol-to-olefins process, polyols and di-isocyanates from CO2 and H2) can be further used as a carbon sink. The saturation time for that can be several times higher, since the annual plastic production in the Netherlands is of the order of 5.3 Mt/yr (Plastics Europe, 2020), equivalent to 16.7 Mt CO2/yr. It is unclear how carbon loops of exported plastics will be closed in the future by (chemical) recycling and carbon capture at incineration, thus actual sink capacity for plastics in the future needs to be determined. Hence, such an integrated FCEVs-SOFC system with CO2 utilization is a sustainable way to close the carbon loop and has great potential in achieving negative emissions.
The use of SOFC makes it possible to readily capture pure CO2 from a car. However, there are also some challenges to the use of SOFCs in small applications like cars. Start-up and shut-down times are quite long, and thick insulation and large heat exchangers are needed for high efficiency. At present, the cost and durability of SOFCs also need improvement. On the other hand, long-term operation (several hours/days) of a downscaled SOFC (charging a larger battery), insulated with industrial ceramic fiber can mitigate this. Moreover, a prototype bioethanol-powered SOFC car was already produced by Nissan in 2016 (Nissan Motor Corporation [NMC], 2016). The costs for the CO2 compressor and other on-board components required for implementing the proposed system will be calculated as part of future work.
Conclusion
A novel pathway for negative emissions was postulated based on second-generation biofuel followed by carbon circularity in a closed chain, effectively resulting in a sink. Application of an on-board SOFC in an FCEV results in a concentrated stream of CO2, which is readily stored in a second tank. No energy-intensive CO2 separation and capture process is required. A CO2 recovery system at the fuel station takes the CO2 from the tank, stores it, or feeds it in a reverse-logistic routing back to a methanol plant. Using renewable H2, the recovered CO2 is synthesized into an e-fuel like methanol, which is also used as a fuel in SOFCs, fully closing the carbon loop with indefinite carbon recycling. We determined this pathway can reach typical negative emissions of 0.515 ton CO2 per car converted to SOFC using bioethanol fuel with CCU and a total negative emission of 138 Mt for all passenger cars in the EU. Such an integrated FCEV-SOFC system with CO2 utilization is a sustainable way to close the carbon loop and offers great potential in achieving negative emissions. All steps comprise known technologies with medium to high TRL levels and do not require costly system changes, so principally this system can readily be applied in the mid-term. The assumption for the lag time (between car exhaust collection and methanol production) is very important to decide the total negative emissions, and needs further investigation to decide an appropriate value (1 year has been assumed here as an indicative value). Detailed calculations for such a carbon sink will be carried out as future work.
Author Contributions
BCJ: conceptualization, investigation, and writing—original draft, review, and editing. P-CK, AA, and PVA: conceptualization, investigation, and writing—review and editing. WvN: conceptualization, methodology, and writing—review. All authors contributed to the article and approved the submitted version.
Conflict of Interest
BCJ and WvN were employed by company Circonica Circular Energy B.V.
Circonica Circular Energy B.V. has a commercial interest in the development of solid oxide fuel cells.
The remaining authors declare that the research was conducted in the absence of any commercial or financial relationships that could be construed as a potential conflict of interest.
Acknowledgments
A preprint version of this manuscript has been uploaded to OSF Preprints (Jaspers et al., 2021).
References
Biondoil (2021). Biondoil Website, Page: Bioethanol Refinery. Available online at: https://biondoil.eu/bioethanolrefinery (accessed February 07, 2021).
Bos, M. J., Kersten, S. R. A., and Brilman, D. W. F. (2020). Wind power to methanol: renewable methanol production using electricity, electrolysis of water and CO2 air capture. Appl. Energy 246:114672. doi: 10.1016/j.apenergy.2020.114672
CBS (2021). Verkeersprestaties Personenauto’s; Kilometers, Brandstofsoort, Grondgebied. 2020-11-04. Available online at: https://www.cbs.nl/nl-nl/cijfers/detail/80428ned (accessed February 07, 2021).
Cuccia, L., Dugay, J., Bontemps, D., Louis-Louisy, M., and Vial, J. (2018). Analytical methods for the monitoring of post-combustion CO2 capture process using amine solvents: a review. Int. J. Greenhouse Gas Cont. 72, 138–151. doi: 10.1016/j.ijggc.2018.03.014
Devin Serpa (2021). Tank to Wheel Efficiency. Available online at: http://www.afteroilev.com/Pub/EFF_Tank_to_Wheel.pdf (accessed February 07, 2021).
European Automobile Manufacturers Association [EAMA] (2019). European Automobile Manufacturers Association. Report: Vehicles in Use. Available online at: https://www.acea.be/uploads/publications/ACEA_Report_Vehicles_in_use-Europe_2019.pdf (accessed February 07, 2021).
Fasihi, M., Efimova, O., and Breyer, C. (2019). Techno-economic assessment of CO2 direct air capture plants. J. Clean. Prod. 224, 957–980. doi: 10.1016/j.jclepro.2019.03.086
Fernandes, A., Woudstra, T., van Wijk, A., Verhoef, L., and Aravind, P. V. (2016). Fuel cell electric vehicle as a power plant and SOFC as a natural gas reformer: an exergy analysis of different system designs. Appl. Energy 173, 13–28.
Jaspers, B. C., Kuo, P., Amladi, A., van Neerbos, W., and Aravind, P. V. (2021). Negative CO2 Emissions for Transportation. OSF Preprints, 14-02-2021. Available online at: https://doi.org/10.31219/osf.io/t8c9p (accessed February 15, 2021).
Kainiemi, L., Eloneva, S., Toikka, A., Levanen, J., and Jarvinen, M. (2015). Opportunities and obstacles for CO2 mineralization: CO2 mineralization specific frames in the interviews of Finnish carbon capture and storage (CCS) experts. J. Clean. Prod. 94, 352–358. doi: 10.1016/j.jclepro.2015.02.016
Nissan Motor Corporation [NMC] (2016). Nissan Unveils World’s First Solid-Oxide Fuel Cell vehicle. Available online at: https://global.nissannews.com/en/releases/nissan-unveils-worlds-first-solid-oxide-fuel-cell-vehicle (accessed February 12, 2021).
Nissan Motor Corporation [NMC] (2021). A fuel cell system that generates electricity from bioethanol to power a vehicle. Available online at: https://www.nissan-global.com/EN/TECHNOLOGY/OVERVIEW/e_bio_fuel_cell.html (accessed February 07, 2021)
Ong, B. C., Kamarudin, S. K., and Basri, S. (2017). Direct liquid fuel cells: a review. Int. J. Hydrogen Energy 42, 10142–10157.
Plastics Europe (2020). Levencyclus Van Plastics in Nederland, 2018. Available online at: https://www.plasticseurope.org/application/files/6915/7951/9883/PlasticEuropeNL_-_Infographic_2018_-_final.pdf (accessed September 11, 2020)
Richards, P., and Coley, T. (2005). Automotive Fuels Reference Book, ISBN 9780768087932, 3rd Edn. Warrendale, PA: SAE International.
Sharma, S., and Maréchal, F. (2019). Carbon dioxide capture from internal combustion engine exhaust using temperature swing adsorption. Front. Energy Res. 7:143. doi: 10.3389/fenrg.2019.00143
Slater, J. D., Chronopoulos, T., Panesar, R. S., Fitzgerald, F. D., and Garcia, M. (2019). Review and techno-economic assessment of fuel cell technologies with CO2 capture. Int. J. Greenhouse Gas Cont. 91:102818. doi: 10.1016/j.ijggc.2019.102818
Thattai, A. T., Oldenbroek, V., Schoenmakers, L., Woudstra, T., and Aravind, P. V. (2017). Towards retrofitting integrated gasification combined cycle (IGCC) power plants with solid oxide fuel cells (SOFC) and CO2 capture-A thermodynamic case study. Appl. Thermal Eng. 114, 170–185. doi: 10.1016/j.applthermaleng.2016.11.167
Travelcard (2020). Wat is uw Praktijkverbruik?. Available online at: https://www.praktijkverbruik.nl/default.aspx (accessed September 11, 2020).
Keywords: negative emissions, CO2 utilization, SOFC, BECCS technologies, FCEV, bioethanol (fuel alcohol)
Citation: Jaspers BC, Kuo P-C, Amladi A, van Neerbos W and Aravind PV (2021) Negative CO2 Emissions for Transportation. Front. Energy Res. 9:626538. doi: 10.3389/fenrg.2021.626538
Received: 06 November 2020; Accepted: 23 March 2021;
Published: 20 April 2021.
Edited by:
Ah-Hyung Alissa Park, Columbia University, United StatesReviewed by:
Shweta Singh, Purdue University, United StatesHyungwoong Ahn, University of Edinburgh, United Kingdom
Copyright © 2021 Jaspers, Kuo, Amladi, van Neerbos and Aravind. This is an open-access article distributed under the terms of the Creative Commons Attribution License (CC BY). The use, distribution or reproduction in other forums is permitted, provided the original author(s) and the copyright owner(s) are credited and that the original publication in this journal is cited, in accordance with accepted academic practice. No use, distribution or reproduction is permitted which does not comply with these terms.
*Correspondence: Po-Chih Kuo, cGNrdW8yMjVAZ21haWwuY29t; cC5jLmt1b0B0dWRlbGZ0Lm5s