- 1School of Biological Sciences, Illinois State University, Normal, IL, United States
- 2Department of Biochemistry and Center for Plant Science Innovation, University of Nebraska-Lincoln, Lincoln, NE, United States
- 3Department of Biochemistry and Molecular Biophysics, Kansas State University, Manhattan, KS, United States
- 4School of Agriculture, Western Illinois University, Macomb, IL, United States
Thlaspi arvense L. (pennycress) is a cold-tolerant Brassicaceae that produces large amounts of seeds rich in triacylglycerols and protein, making it an attractive target for domestication into an offseason oilseed cash cover crop. Pennycress is easily genetically transformed, enabling synthetic biology approaches to tailor oil properties for specific biofuel and industrial applications. To test the feasibility in pennycress of producing TAGs and acetyl-TAGs rich in medium-chain fatty acids (MCFAs; C6–C14) for industrial, biojet fuel and improved biodiesel applications, we generated transgenic lines with seed-specific expression of unique acyltransferase (LPAT and diacylglycerol acyltransferase) genes and thioesterase (FatB) genes isolated from Cuphea viscosissima, Cuphea avigera var. pulcherrima, Cuphea hookeriana, Coco nucifera, and Umbellularia californica. Wild-type pennycress seed TAGs accumulate no fatty acids shorter than 16C and less than 5 mol percent C16 as palmitic acid (16:0). Co-expressing UcFatB and CnLPAT produced up to 17 mol% accumulation of lauric acid (12:0) in seed TAGs, whereas CvFatB1 CvLPAT2 CpDGAT1 combinatorial expression produced up to 27 mol% medium chain FAs Medium Chain Fatty Acids mostly in the form of capric acid (10:0). CpFatB2 ChFatB2 combinatorial expression predominantly produced, in equal parts, up to 28 mol% myristic acid (14:0) and palmitic acid. Genetically crossing the combinatorial constructs into a fatty acid elongation1 (fae1) mutant that produced no 22:1 erucic acid, and with an Euonymus alatus diacylglycerol acetyltransferase (EaDAcT)-expressing line that produced 60 mol% acetyl-TAGs, had no or relatively minor effects on MCFAs accumulation, suggesting fluxes to MCFAs were largely unaltered. Seed germination assays revealed no or minor delays in seed germination for most lines, the exception being CpFatB2 ChFatB2-expressing lines, which had substantially slower seed germination rates. Taken together, these data show that pennycress can be engineered to produce seeds accumulating modest amounts of MCFAs of varying carbon-chain length in TAGs and acetyl-TAGs, with rates of seed germination being delayed in only some cases. We hypothesize that increasing MCFAs further may require functional reductions to endogenous transferases and/or other FA elongases.
Introduction
Brassicaceae seeds accumulate high levels of triacylglycerols (TAGs, oil) in embryos where they function as an energy source and carbon skeletons for the germinating seedlings (Murphy and Cummins, 1989; Chapman and Ohlrogge, 2012). TAGs are composed of three fatty acids (FAs) of various carbon-chain lengths and degrees of unsaturation that are attached by ester bonds to the three stereospecific positions (sn-1, sn-2, and sn-3) of a glycerol backbone (Chapman and Ohlrogge, 2012; Bansal and Durrett, 2016). The seeds of Thlaspi arvense (Field Pennycress, pennycress), which is a Brassicaceae species being developed as an oilseed cash cover crop (Isbell, 2009; Sedbrook et al., 2014), accumulate ∼33% TAGs by dry weight composed primarily of the FAs erucic acid (22:1; ∼33%), oleic acid (18:1; ∼13%), linoleic acid (18:2; ∼20%), and linolenic acid (18:3; ∼15%) (Moser et al., 2009). Pennycress TAGs contain no Medium Chain Fatty Acids (MCFAs), which are defined as having carbon chain lengths of C8–C14 (Drenth et al., 2015).
Pennycress has a relatively small diploid genome (Dorn et al., 2015) and can be genetically transformed using an Agrobacterium tumefaciens floral dip method (McGinn et al., 2019). These features make this species relatively easy to manipulate genetically, for example by employing Ethyl methanesulfonate (EMS) mutagenesis (Chopra et al., 2018), Clustered Regularly Interspaced Short Palindromic Repeats (CRISPR) gene editing, and/or introduction of transgenes conferring traits of interest such as seed acetyl-TAGs production (McGinn et al., 2019). Scientists at the start-up company, CoverCress, Inc., in partnership with academic and government researchers are employing these molecular tools in conjunction with breeding to domesticate pennycress into an oilseed cash cover crop. Pennycress crop varieties will produce seed oil having low erucic acid content for food, feed, and fuel uses (Chopra et al., 2018; McGinn et al., 2019; Chopra et al., 2020; Sedbrook and Durrett, 2020), high erucic acid for industrial uses including for conversion to biojet fuel and biodiesel (Nieschlag and Wolff, 1971; Isbell et al., 2015; Tao et al., 2017; Altendorf et al., 2019), and high oleic acid desirable in food and fuels owing to its oxidative stability and cold-flow properties suitable for biodiesel (Moser et al., 2009; Moser, 2012; Chopra et al., 2020).
The genetic diversity available in pennycress and closely related species limits oil quality improvement through conventional breeding to modifying the relative amounts of existing fatty acids in pennycress oil. To expand genetic diversity for producing novel fatty acids that add value to pennycress oil, we took a biotechnological approach to introduce genes from non-related and non-agronomic plant species that produce extreme amounts of MCFAs and acetyl-TAGs that are not naturally accumulated in pennycress seeds. In this regard, some plant species, including the genus Cuphea which grow in temperate climates, contain seed TAGs with as much as 94 mol percent MCFAs (Graham and Kleiman, 1992). For example, Cuphea viscosissima (Cv) seed oil accumulates up to 69% capric acid (10:0) (Knothe et al., 2009; Kim et al., 2015b), Cuphea palustris (Cp) accumulates mainly myristic acid (14:0), while Cuphea avigera var. pulcherrima (Cpu) and Cuphea hookeriana (Ch) produce mainly caprylic acid (C8:0; Graham, 2016). Tropically grown coconut (Cocos nucifera; Cn) oil is also rich in MCFAs, most commonly, lauric acid (12:0). MCFAs are used in many industrial and consumer products including detergents, soaps, cosmetics, surfactants, and lubricants (Knaut and Richtler, 1985; Carlsson et al., 2011). MCFAs and the long chain fatty acid, palmitic acid (16:0), are also important as components of biojet fuels, as they have similar properties to petroleum-derived alkanes of the same carbon chain lengths (Kallio et al., 2014; Kim et al., 2015b). The market for jet fuel is considerable, with global consumption by commercial airlines reaching 96 billion gallons in 2019 (Mazareanu, 2020). There is impetus to develop low carbon-intensity biojet fuels and biodiesel to lower the carbon footprint of aviation and heavy transportation thereby mitigating climate change (Ringbeck and Koch, 2010; Morgan, 2014; Sindelar et al., 2017), hence the interest in engineering industrial oilseed crops such as pennycress and Camelina sativa (camelina) to produce MCFAs for direct use (Ringbeck and Koch, 2010; Morgan, 2014; Kim et al., 2015b).
Many of the key genes involved in MCFAs biosynthesis and incorporation into TAGs have been identified and characterized (Pollard et al., 1991; Tjellström et al., 2013; Kim et al., 2015a; Kim et al., 2015b; Iskandarov et al., 2017). Of importance are FatB homologues that function as MCFA-specific thioesterases, as well as two types of acyltransferases, namely lysophosphatidic acid acyltransferase (LPAT) and diacylglycerol acyltransferase (DGAT) (Dehesh et al., 1996). For example, the FatB homologues, ChFatB2 and CpFatB2, of Cuphea species are responsible for generating free MCFAs C10 and C14 in length, respectively, which become incorporated into seed TAGs (Kim et al., 2015a; Kim et al., 2015b). The LPAT homologous CnLPAT from coconut, and CvLPAT2 and CpuLPAT2a from C. viscosissima and C. avigera var. pulcherrima, preferentially incorporate MCFA at the sn-2 position of lysophosphatidic acid as part of the Kennedy pathway and TAG production (Knutzon et al., 1999; Kim et al., 2015a). DGAT is responsible for acylating the sn-3 position of diacylglycerol (DAG) thereby completing TAG formation. DGAT from C. avigera var pulcherrima (CpuDGAT1), when expressed in camelina seed microsomes, increased C10 incorporation into TAGs by 4-fold (Iskandarov et al., 2017).
Acetyl-TAGs are unusual TAG molecules produced primarily by members of the Euonymus genus, where the sn-3 position of the molecule is occupied by an acetate group rather than a long chain fatty acid. Acetyl-TAGs possess physical properties useful for biofuels, including reduced viscosity and lower melting points (Durrett et al., 2010; Liu et al., 2015a). Acetyl-TAGs are synthesized in the seeds of Euonymus alatus by the DAG acetyltransferase EaDAcT, which uses acetyl-CoA to acetylate DAG (Durrett et al., 2010). High levels of acetyl-TAGs can be synthesized in transgenic plants, including pennycress, through the expression of the EaDAcT acetyl-transferase (Liu et al., 2015a; Liu et al., 2015b; McGinn et al., 2019).
For this study, we aimed to determine if Thlaspi arvense (pennycress) seeds can accumulate MCFAs when co-expressing combinations of the above-highlighted FatB and acyltransferase genes in a seed-specific manner, and how well that MCFA accumulation would be tolerated as measured by seed viability, rates of germination, and overall plant growth and seed yields. We also examined how MCFA accumulation is affected by knockout of erucic acid production and/or in combination with synthesis of acetyl-TAGs. Our results showed that MCFAs of various chain lengths (C8–C16) can be produced and incorporated into pennycress seed oil at modest levels, but may result in reductions in total seed oil content (from 9 to 43 percent reductions under our conditions) and reduced rates of seed germination when MCFAs are incorporated in TAGs at high levels.
Materials and Methods
Plant Materials, Growth Conditions and Genetic Crosses
The pennycress “Spring 32” spring-type ecotype was used for this study. Plants were grown in growth chambers cycling 16–8 h light and dark, respectively, at 21 or 22°C. Four plants per four-inch pot were planted as described previously (McGinn et al., 2019). DsRed-derived red fluorescence was used to track the MCFA constructs in plants. Putative T1 transformants expressing DsRed were used for genetic crosses with a CRISPR-Cas9-induced fae1-3 mutant line and a transgenic line expressing EaDAcT that also expressed DsRed as the marker (Chopra et al., 2018; McGinn et al., 2019). Genomic DNA from F2 segregants were extracted using a modified CTAB (cetyl trimethylammonium bromide) method (Doyle and Doyle, 1987) followed by PCR amplification for identifying the 4 bp deletion in the fae1-3 mutant allele and the presence of EaDAcT and MCFA biosynthetic genes Primer sequences and expected PCR product sizes are listed in Supplementary Table S1.
Binary Vectors and Transformation
Previously reported binary vectors containing four combinations of LPAT, DGAT and FatB, UcFatB + CnLPAT (Kim et al., 2015b), CvFatB1 + CvLPAT2 (Kim et al., 2015b; Iskandarov et al., 2017), CvFatB1 + CvLPAT2 + CpuDGAT1 (Iskandarov et al., 2017), and CpFatB2 (Thio14) + ChFatB2 (Kim et al., 2015b; Iskandarov et al., 2017) were introduced into Agrobacterium tumefaciens strain GV3101. Pennycress plants were transformed using a floral dip/vacuum infiltration method, and DsRed fluorescence protein marker was used to screen putative transgenic T1 seedlings, as previously described (McGinn et al., 2019). Homozygous T2 transgenic lines were identified by screening for T3 seedlings that all fluoresced red due to expression of the DsRed transgenic marker.
Seed Germination and Plant Health Analyses
Pennycress seeds were surface sterilized with a 70% ethanol rinse and 12 min 30% bleach +0.01% sodium dodecyl sulfate (SDS) soak then sterile water rinse as described in McGinn et al. (2019), then sown onto growth media plates consisting of 0.8% agar (BioWORLD Cat. #30620001-4) and one-half strength Murashige and Skoog (MS) salts (Caisson Laboratories Cat. #MSP09-50LT; Murashige and Skoog, 1962). MS salts are a mixture of macronutrients and micronutrients commonly used in tissue culture work. The plates were placed into a Percival Scientific CU-36L5 incubator (22°C, 16 h 4,100K fluorescent light ∼150–200 μE/m2/s/8 h dark) for approximately 2–3 weeks. Seed germination rates were measured every day at a certain time for each subsequent generation. Eight T2-generation homozygous plants per line grown alongside wild type were measured for their heights upon senescing and their T3 seeds were used for weight analysis. Single-seed sizes and weights were determined using the dimensional measuring and counting functions of a MARViTECH MARViN 5.0 Seed Analyzer instrument along with an Ohaus STX223 balance (Single-seed weight = total seed weight/number of seeds). R Studio software was used to perform the statistical analyses. Averages and standard deviations were calculated for heights of T2-generation plants at maturity and total and single seed lengths, widths, areas, and weights of T3 seed produced. t-tests comparing insertional events to wild type were performed on these averages.
Fatty Acids Analysis
Oil fatty acid analysis of dry pennycress seeds was performed as described previously (Kim et al., 2015b). One milligram of tri 17:0-TAG (Nu-Chek Prep, MN, United States) in toluene (10 mg ml−1) was used as an internal standard. Acetyl-TAG content and FA composition were quantified by extracting total lipids from seeds with a hexane-isopropanol-based method (Li et al., 2006). Thin layer chromatography was then used to separate the total lipid extract. TAG and acetyl-TAG bands were scraped and subject to base-catalyzed transesterification, followed by gas chromatography using a DB-23 column (30 m by 0.25 mm diameter, 0.25 µm film) on a Shimadzu GC2010plus gas chromatograph (Bansal et al., 2018).
Total Seed Oil and Moisture Determination
Total oil content and moisture were determined by nondestructive TD-NMR on 150 or 450 mg samples of whole pennycress seed, using a Bruker Minispec MQ40 with a 39.95 MHz NMR frequency and 40 C magnet temperature. The 90 and 180° pulse lengths were 11.48 and 22.98 µs, respectively. A 23° detection angle, gain of 44 dB, pulse attenuation of 15 dB, recycle delay of 2 s, window 1 of 0.055 ms, window 2 (τε) of 7 ms, and 724 magnetic field steps were set to employ a Hahn spin echo effect for signal collection. Each 150 and 450 mg seed sample and standard analysis employed 32 and 16 scans with receiver gains of 68 and 59, respectively. The percent oil and moisture for each sample was calculated using the Bruker Oil and Moisture in Seeds application in minispec Plus version 7.0.0 software.
Percent oil calibration curves were constructed within the Bruker software by measuring the signal for 12 oil standards between 20 and 40% oil concentration based on 150 and 450 mg samples. Crude pennycress oil in six amounts ranging from 30 to 60 mg for the 150 mg calibration and six amounts ranging from 90 to 180 mg for 450 mg calibration were carefully dropped down 12 10 mm flat bottom NMR tubes containing a piece of kimwipe in the bottom of each tube. After the oil standards were tempered at 40°C in an Organomation MULTIVAP 48 heat block for 30 min, they were scanned in the calibration mode to create signal vs. concentration calibration curves for percent oil for 150 and 450 mg samples.
Since free water behaves differently than bound water, the moisture calibration curves were constructed by running 150 and 450 mg pennycress seed samples with varying known moisture levels. The moisture levels of six different reference standards were determined by recording the mass of six 20 ml glass scintillation vials, adding 10 g of seed from each of the six reference samples into vials, recording the total mass of the seed and vial for each reference sample. Samples were dried in open vials in a convection oven at 105°F for 3 h, placing the lids on the vials immediately after removing them from the oven, cooling the vials in a sealed desiccator, and recording the masses of the vials and seeds after cooling. An Excel spreadsheet was used to calculate the percent moisture for the six reference standards. The mass lost during drying divided by the mass of the seed before drying times 100 produced a percent moisture value for each reference standard. A percent moisture calibration curve was constructed within the Bruker software by measuring the signal for six moisture reference standards with moisture levels between 7.8 and 14.1% moisture. An analytical balance was used to measure 150 and 450 mg of seed from each of the six moisture reference standards into 12 10 mm flat bottom NMR tubes. After the tubes were tempered at 40°C in a heat block for 30 min, they were scanned in the calibration mode to create a signal vs. concentration calibration curve for percent moisture.
To determine percent oil and moisture in seeds from the sample plots, an analytical balance was used to weigh 150 or 450 mg of seed from each construct into 10 mm flat bottom NMR tubes. After tempering the samples at 40°C for 30 min, they were scanned and the percent oil and moisture were calculated using our calibration curves and the Bruker Oil and Moisture in Seeds application in minispec Plus software. The results from minispec Plus were exported to an Excel file. Using Excel, the percent oil on a dry weight basis was calculated by dividing the milligrams of oil in each sample by the dry weight of each sample and multiplying by 100.
Analysis of variance (ANOVA) was performed on % oil dry weight basis (DWB) using R Studio software. Levels of statistically significant factors at the 0.05 significance level were compared with Tukey’s HSD method for multiple comparisons. A box plot was also created in R Studio.
Results and Discussion
Introduction of MCFA Biosynthetic Gene Combinations into Pennycress Results in MCFA Incorporation into Seed TAGs
The fatty acids (FAs) incorporated into Thlaspi arvense L. (pennycress) seed triacylglycerols (TAGs; oils) are primarily 18, 20, and 22 carbons in length and are mostly mono- and poly-unsaturated (18:1, 18:2, 18:3, 20:1, and 22:1; Figure 1; Table 1). Pennycress seed TAGs contain no medium chain fatty acids (MCFAs; C8–C14) and only about 4 mol percent C16 fatty acids primarily as the saturated FA, palmitic acid (16:0; Figure 1; Table 1). To determine if pennycress seed TAGs could be engineered to accumulate MCFAs of different chain lengths and, if so, how seed germination and viability would be affected, stable transgenic lines containing the following four gene combinations were generated and analyzed: CvFatB1 + CvLPAT2; CvFatB1 + CvLPAT2 + CpuDGAT1; UcFatB + CnLPAT; and CpFatB2 (also known as Thio14) + ChFatB2. These constructs were previously shown to be functional in Camelina sativa (camelina) (Kim et al., 2015b; Iskandarov et al., 2017). All genes were expressed under control of the seed-specific soybean Glycinin-1 promoter, to avoid possible toxicity in vegetative tissues.
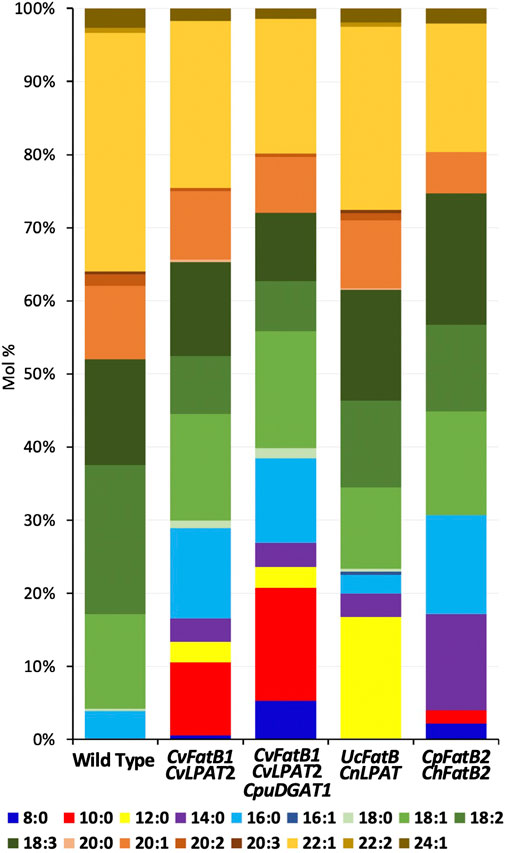
FIGURE 1. Fatty acid (FA) compositions of seed TAGs of transgenic lines harboring one of the four MCFA biosynthetic gene combinations. Shown are data from the lines having the highest MCFA accumulation. Average FA mol% amounts along with standard deviations are shown in Table 1.
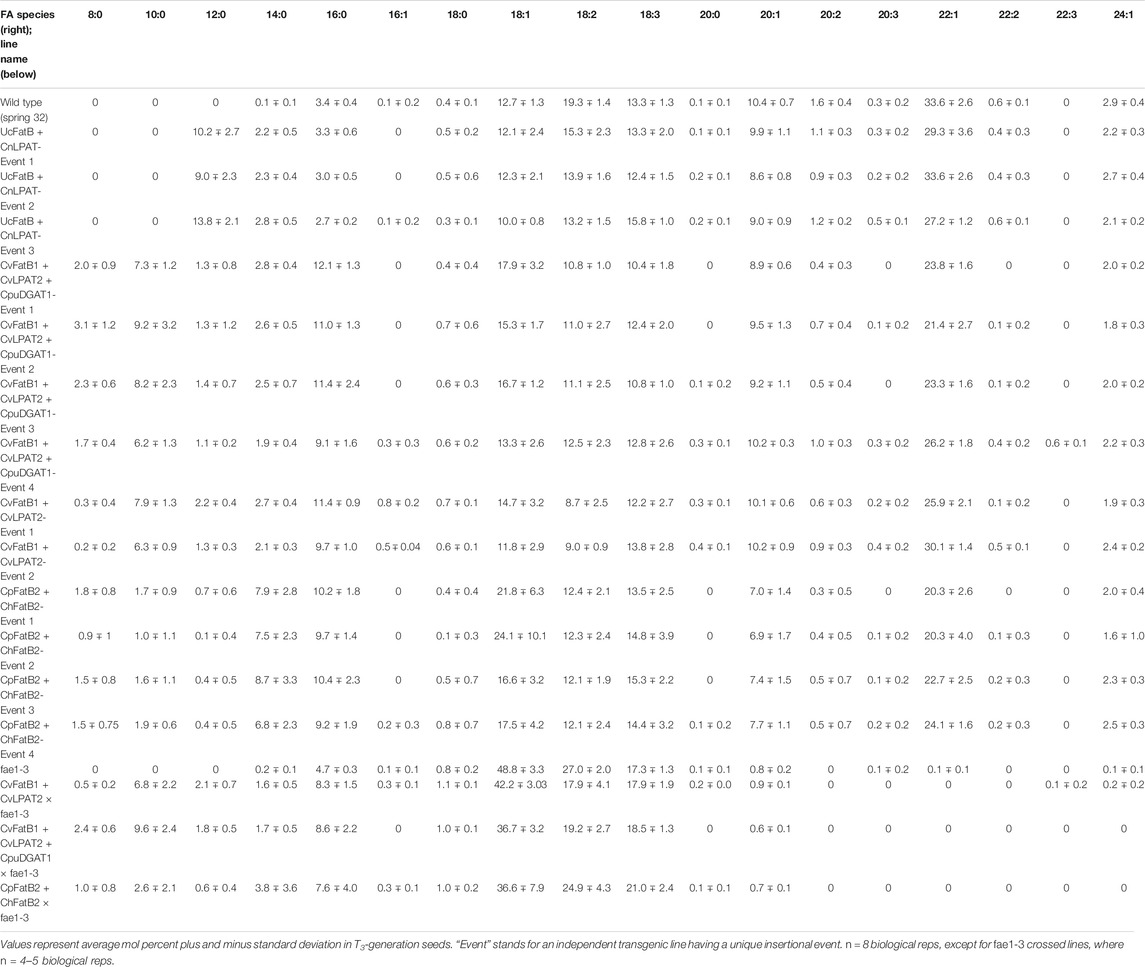
TABLE 1. Amounts of fatty acid (FA) species in TAGs or acetyl-TAGs of pennycress MCFA biosynthetic genes-containing transgenic lines as well as stacks of those lines with an fae1-3 knockout mutation or seed-specific-expressed EaDAcT transgene.
Seeds from T2-generation pennycress plants expressing CvFatB1 + CvLPAT2 in the T3 developing embryos produced TAGs containing from 7.5 to 16.6 mol% MCFAs (Figure 1; Table 1; Supplementary Figure S1). The most abundant MCFA produced was 10:0, which accumulated at levels up to 10 mol%. Smaller amounts of 14:0, 12:0, and 8:0 were also synthesized. In addition, the amount of 16:0 incorporation into seed TAGs also increased significantly in these lines (5.6–12.7 mol% vs. 3.1–3.9 mol% in wild type). In transgenic pennycress plants also containing the CpuDGAT1 gene in addition to the CvFatB1 + CvLPAT2 genes (CvFatB1 + CvLPAT2 + CpuDGAT1), 8:0 and 10:0 incorporation increased further, up to 5.3 mol% 8:0 and up to 15.5 mol% 10:0; Figure 1; Table 1; Supplementary Figure S2), whereas the ranges of 12:0, 14:0, and 16:0 MCFAs incorporation were about the same as observed with CvFatB1 + CvLPAT2 transgenic lines. Total MCFA incorporation in the three-gene-expressing seeds was from 6.7 to 26.9 mol%. When taking into account C16, which can also be used in biojet fuels, total MCFAs plus C16 was from 13.5 to 38.5 mol%.
The pennycress CvFatB1 + CvLPAT2 as well as CvFatB1 + CvLPAT2 + CpuDGAT1 seed oil MCFA compositions (with respect to MCFA species incorporated into TAGs) were comparable to those observed in camelina seeds transgenic for these same gene combinations (Iskandarov et al., 2017). A somewhat surprising finding made by this earlier study was that the higher 8:0 and 10:0 incorporation observed in camelina plants, when expressing CpuDGAT1 in combination with CvFatB1 + CvLPAT2, was found to be due, at least in part, to higher incorporation of 10:0 at the sn-2 position of the TAGs (Iskandarov et al., 2017). One would have expected 8:0 and 10:0 incorporation to occur mainly if not exclusively at the sn-3 position, given the catalytic activity of DGAT enzymes. The authors interpreted this result as meaning CpuDGAT1 selectively channels diacylglycerol (DAG) containing 10:0 in the sn-2 position for TAG biosynthesis. Our results suggest this phenomenon may also occur in pennycress.
Kim et al. (2015b) previously showed that seed-specific expression of UcFatB + CnLPAT in camelina produced nearly 30 mol% 12:0 in seed TAGs. To test if this level of 12:0 incorporation could also be engineered into pennycress, we stably transformed the same construct into pennycress and analyzed TAG FA content in T3-generation seeds. Compositional analysis of FAs in TAGs from seeds of 23 lines representing three independent insertional events identified the highest 12:0 accumulation to be 16.7 mol%, with the average 12:0 accumulation for seeds from plants of the three lines being 13.9 (event 3), 10.2 (event 1), and 9.0 (event 2) mol%, respectively (Figure 1; Table 1; Supplementary Figure S3). Expression of this construct produced no 8:0 and no 10:0 MCFAs (consistent with what was observed in camelina) and, on average, 2.7–3.3 mol% 16:0, which is similar to wild-type levels.
As was observed with the CvFatB1 + CvLPAT2 pennycress data vs. the previously published camelina data (Kim et al., 2015b; Iskandarov et al., 2017), the levels of MCFAs accumulation in pennycress seed oil derived from UcFatB + CnLPAT expression did not attain those observed in camelina containing the same construct. This could possibly be due to the fact that the location where a DNA construct randomly inserts into a chromosome has a large effect on gene expression levels. As such, screening for additional pennycress transformants might increase the probability of uncovering pennycress lines having insertional events in chromosomal locations promoting higher gene expression and related MCFAs accumulation comparable to that observed in camelina. In addition, the endogenous pennycress FatB, LPAT, and DGAT enzymes might have higher activity than the equivalent camelina enzymes allowing them to better outcompete the introduced enzymes. Introducing mutations that reduce the endogenous genes’ functions would test this hypothesis. Finally, too high expression of the introduced enzymes could be relatively more toxic/lethal in pennycress vs. camelina or otherwise inhibit germination, resulting in high-expressing pennycress insertional events not being recovered. To address this third possibility, we plated seeds of the various transgenic events on Murashige and Skoog salts-containing agar media and scored seed germination over time, observing that all but one CvFatB1 + CvLPAT2, CvFatB1 + CvLPAT2 + CpuDGAT1, and UcFatB + CnLPAT transgenic lines had 100% or near-100% seed germination (Figures 2A–D). While the seeds of many lines germinated at the same rate as wild type, some lines did exhibit slight delays in seed germination. Seeds from one UcFatB + CnLPAT line (Event 3; Figure 2C) germinated considerably slower and had only about 50% germination after 8 days whereas the other lines and wild type had 100% germination by day 6. Taken together, these data suggest that the levels of MCFA production and incorporation into pennycress seed TAGs of the CvFatB1 + CvLPAT2 and CvFatB1 + CvLPAT2 + CpuDGAT1 lines were minimally inhibitory to germination and therefore it seems likely that lines with higher MCFA production would likely be viable.
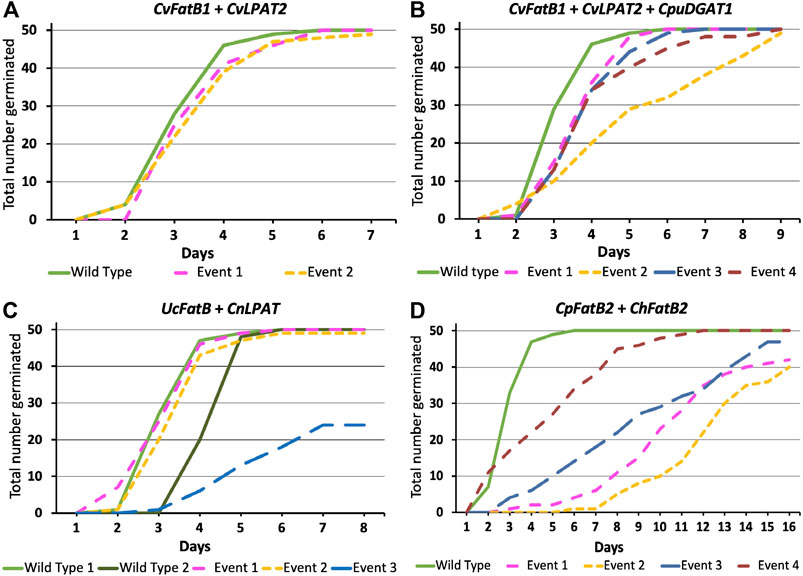
FIGURE 2. Germination rates of T3-generation seeds from each of the MCFA biosynthetic genes combination lines. Genotypes for each panel are as follows: (A)CvFatB1 + CvLPAT2; (B)CvFatB1 + CvLPAT2 + CpuDGAT1; (C)UcFatB + CnLPAT; (D)CpFatB2 + ChFatB2. “Event” stands for each line arising from an independent transgenic insertional event. The X-axis represents days from plating surface-sterilized seeds on Murashige and Skoog (MS) salts-containing agar media. Fifty seeds in total were plated for each line. For UcFatB + CnLPAT(C), note that Wild Type 1 plants were grown alongside Event 1 and 2 plants whereas Wild Type 2 plants were grown in a different chamber alongside Event 3 plants.
CpFatB2 + ChFatB2-Driven Seed MCFAs Accumulation is Less Well Tolerated
The CpFatB2 + ChFatB2 construct differed from the other three constructs introduced into pennycress in that it contained no LPAT or DGAT genes while containing two FatB genes (from Cuphea palustris and Cuphea hookeriana) which were different from those in the other constructs. Figure 1; Table 1; Supplementary Figure S4 show that seeds transgenic for CpFatB2 + ChFatB2 contained up to 13.1 mol% 14:0 but lower amounts of the other MCFAs. These results are interesting in that they show the endogenous GPAT, LPAT and/or DGAT enzymes were able to incorporate considerable amounts of MCFAs into TAGs (4.3–16.9 mol% MCFAs in total), even though these fatty acids are not typically found in pennycress TAGs. Also noteworthy is that rates of seed germination for all lines were delayed, with Event 2 seed germination being delayed the most (Figure 2D), even though the MCFA content in Event 2 seed TAGs was not appreciably higher (Table 1; Supplementary Figure S4). It is possible that toxicity may be occurring due to a buildup of free fatty acids from suboptimal incorporation of the MCFAs into TAGs by the pennycress endogenous acyltransferases. To test this, one could combine expression of the acyltransferases CvLPAT2 and CpuDGAT1 genes with CpFatB2 and ChFatB2 in order to enhance MCFAs incorporation into TAGs. Alternatively, CpFatB2 + ChFatB2 transgenic seeds contained relatively more 14:0 compared to that produced by the other three constructs, which may somehow inhibit seed germination possibly due to adverse effects on seed embryonic health and/or mechanisms controlling seed dormancy (Yang and Benning, 2018).
Amounts of Seed Oil in MCFA-Producing Lines are Significantly Reduced Compared to Wild Type
MCFA-containing TAG molecules theoretically have reduced mass, compared to the long chain and very long chain fatty acids (LCFA and VLCFA)-containing TAGs normally present in pennycress seed oil, owing to the relatively shorter fatty acid chain lengths. To test if the MCFA-producing transgenic lines had concomitant reduced total amounts of oil in their seeds or instead compensated by producing relatively more TAG molecules, we employed pulsed-field NMR to quantify seed oil percentages on a dry weight basis. Figure 3; Supplementary Figure S5 show that F3 seeds from all lines had, on average, from 9 to 43 percent reductions in seed oil amounts compared to wild type. Interestingly, total seed oil amounts in the CpFatB2 + ChFatB2 transgenic seeds were significantly less than oil amounts in seeds of the lines transgenic with the other constructs, suggesting relatively fewer TAG molecules were being synthesized, perhaps because of relatively poor incorporation of MCFAs into the TAGs due to no MCFA-specific LPAT and DGAT being introduced as noted above. It will be worthwhile to determine if stacking CvLPAT2 and/or CpuDGAT1 with CpFatB2 and ChFatB2 would increase TAG production.
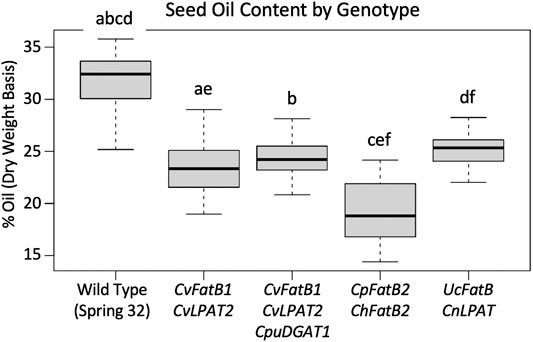
FIGURE 3. Comparison of seed oil (TAGs) amounts in T3-generation seeds of the various transgenic MCFA-producing lines vs. each other and wild type. The data is presented as percent oil per seed on a dry-weight basis. Solid bars in the Box Plots represent each median, gray boxes demarcate the upper and lower quartile, horizontal lines connected by the dotted lines represent the maximum and minimum. Box Plots with the same letter above have means that are significantly different (p < 0.05 as determined by one-way ANOVA followed by Tukey’s HSD test). N = seeds samples from 14 to 28 plants, with those plants representative of the two to four transgenic events. See Supplementary Figure S5 for the seed oil percentages for plants from each transgenic event graphed separately.
MCFA-Producing Lines Grow Comparable to Wild Type, but Many Produce Lighter Seeds
To determine if MCFA production had any negative effects on overall plant growth and seed yields, plant morphologies of T1 and T2-generation transgenic plants were inspected during growth, and overall plant heights and total seed yields were measured when the plants were fully senesced (Figures 4A–H; Supplementary Figures S7–S14). Normal plant growth was anticipated due to transgene expression being limited to seeds by use of the soybean glycinin-1 seed-specific promoter. No morphological differences were observed, and measurement data showed that average plant heights did not differ markedly between the transformants and the wild-type controls grown alongside them, except for UcFatB + CnLPAT Event-3 plants which were on average about three-quarters the height of wild-type plants (Figure 4G; Supplementary Figure S11).
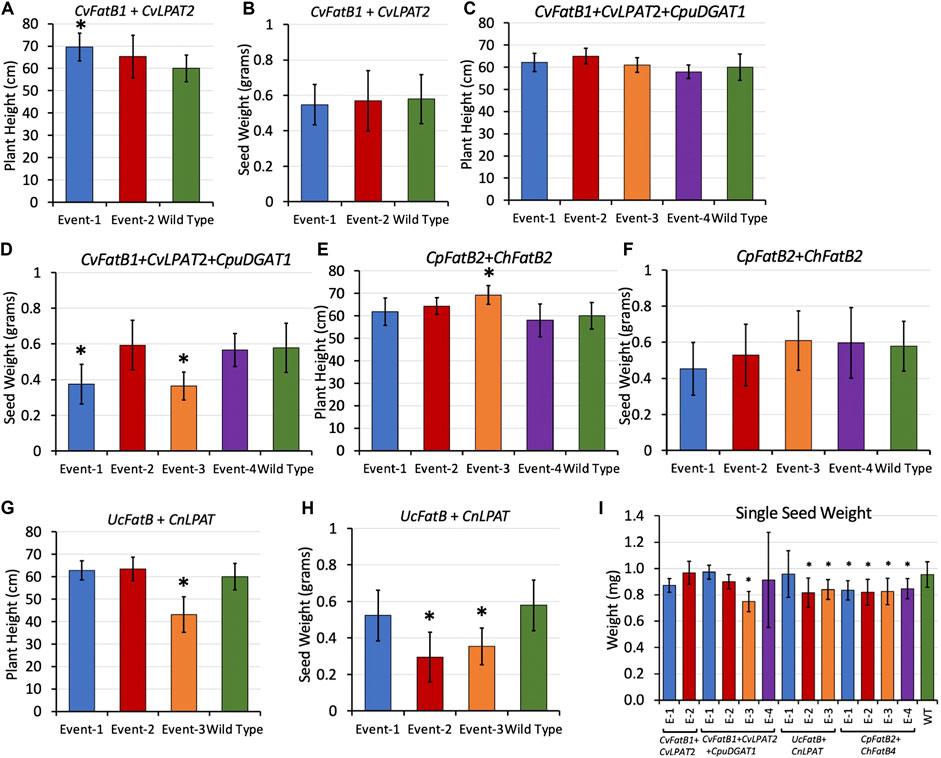
FIGURE 4. Average heights (A,C,E,G) of T2-generation plants at maturity, average total weights (B,D,F,H) of the T3 seeds those plants produced, and (I) average weight per seed for each line. “Event” stands for each line arising from an independent transgenic insertional event. Bars are standard deviation. Asterisks delineate averages that were significantly different from that of wild type grown alongside (p < 0.05 as determined by t-test). For (A–H), n = 8 biological reps. For (I), from 1,519 to 5,532 seeds were measured per plant, 5–11 biological reps. Heights and total seed weights of individual plants can be found in Supplementary Figures S8–S15.
The total weight of seeds produced by each plant were measured, revealing yields similar to wild type except for two CvFatB1 + CvLPAT2 + CpuDGAT1 lines (Events 1 and 3) and two UcFatB + CnLPAT lines (Events 2 and 3). To determine if the lower yields could be attributed at least in part to lighter seeds possibly due to containing MCFA-TAGs having relatively less mass than the LCFA-VLCFA-TAGs normally produced by pennycress, we used a MARViN optical seed analyzer and weight balance to count seeds and measure seed dimensions in determining average weight per seed values. Figure 4I shows that more than half of the transgenic lines produced, on average, seeds that were significantly lighter (from 10 to 20% lighter) than wild-type seeds. MARViN optical analyses of seed dimensions found that CvFatB1 + CvLPAT2 + CpuDGAT1 Event 3 seeds which weighed the least were also significantly albeit slightly smaller than wild type (Supplementary Table S2). Visualization of these seeds using a dissecting microscope found no obvious morphological differences other than the MCFA seeds appeared slightly less plump (Supplementary Figures 9C,D). Taken together, these results suggest seed set of the various transgenic plant lines was largely unaffected, whereas embryos were likely lighter and perhaps smaller in some instances perhaps due to lower mass and volume of MCFA-TAGs.
Abolishing Erucic Acid (22:1) Production has Little Effect on MCFAs Accumulation
Pennycress seed TAGs contain high amounts of erucic acid (22:1), on average 34 mol%, which is in stark contrast with the 2 mol% found in camelina seed TAGs (Kim et al., 2015b; McGinn et al., 2019). To determine if MCFA incorporation in pennycress seed TAGs could be increased by abolishing erucic acid production, we crossed fae1-3 plants with CvFatB1 + CvLPAT2, CvFatB1 + CvLPAT2 + CpuDGAT1, and CpFatB2 + ChFatB2 transgenic lines. Our analyses of F3-generation siblings containing each of the MCFA-producing constructs and homozygous for the fae1-3 knockout mutation found that, while the seed TAGs of some of the siblings contained higher mol percents of MCFAs than the original MCFA construct-containing parents (Figure 5), most did not have appreciably higher incorporation (Supplementary Figures S6A–C; Table 1). These results suggest that the relatively higher MCFA incorporation in camelina seed TAGs compared to pennycress is likely not due to camelina’s much lower erucic acid production. This result is not entirely surprising, given that very long chain fatty acid (VLCFA) synthesis occurs in the cytosol through the elongation of oleoyl-CoA and thus is a distinct process from the elongation of MCFAs bound to ACP that occurs during fatty acid synthesis in the plastid (Chapman and Ohlrogge, 2012).
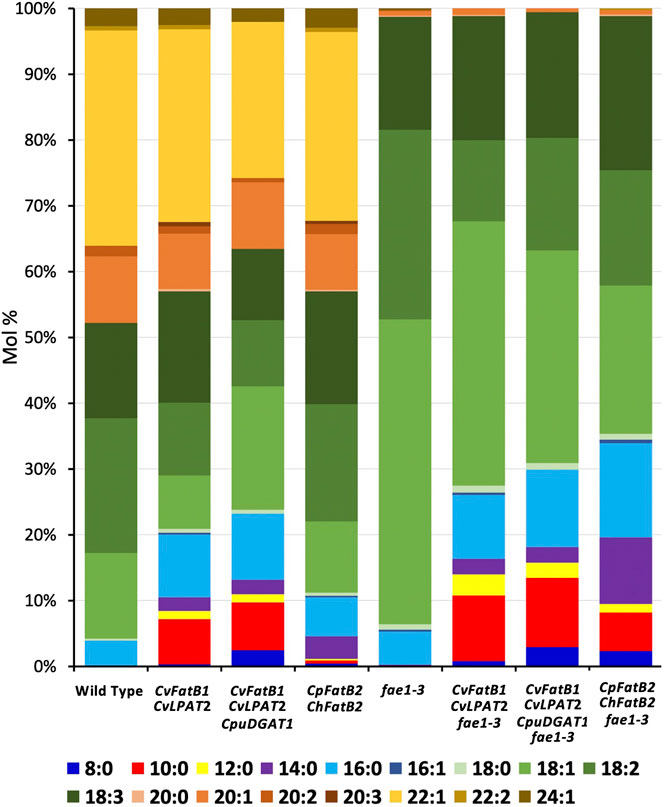
FIGURE 5. Comparison of seed TAG FA amounts in transgenic lines stacked with an fae1-3 knockout mutation compared to the parental lines. Average FA mol% amounts along with standard deviations are shown in Table 1.
To determine if seed germination was at all affected by stacking the fae1-3 mutation with the MCFA biosynthetic transgenes, germination studies were performed, with the rates of germination for the stacked low erucic-MCFA TAGs lines being found to be similar to those observed for the MCFA transgenic lines by themselves, suggesting abolishing erucic acid production did not affect seed viability and vigor under our growth conditions (Supplementary Figure S15).
Synthesis of Tailored Lipids With a Targeted Structure
The ability to metabolically engineer fatty acid production in pennycress enables the generation of transgenic plants designed to produce molecules with a targeted structure that can be used for specific applications. To further alter the properties of acetyl-TAG produced in pennycress, we crossed lines producing high levels of MCFA with existing lines expressing EaDAcT (Durrett et al., 2010; McGinn et al., 2019) to generate plants capable of synthesizing acetyl-TAG with MCFA. The segregating F2 population was genotyped to identify plants with transgenes for both MCFA and acetyl-TAG production. Quantification of TAG and acetyl-TAG in the seeds from these plants revealed a range of acetyl-TAG levels (Figure 6). Plants expressing CvFatB1 + CvLPAT2 + CpDGAT1 in combination with EaDAcT failed to produce levels of acetyl-TAG similar to that of the EaDAcT parent line. This result is consistent with the additional expression of CpDGAT1, which likely competes with EaDAcT for DAG substrate (Liu et al., 2015a). In contrast, some seeds co-expressing either UcFatB + CnLPAT or CpFatB2 + ChFatB2 with EaDAcT possessed acetyl-TAG levels commensurate with the EaDAcT parent. However, certain individual plants with these genotypes also produced seed with considerably lower levels of acetyl-TAG (20 mol% or less; Figure 6). The reason for this decrease is unclear; future work examining the acetyl-TAG levels in subsequent generations derived from these plants will determine whether these differences are heritable.
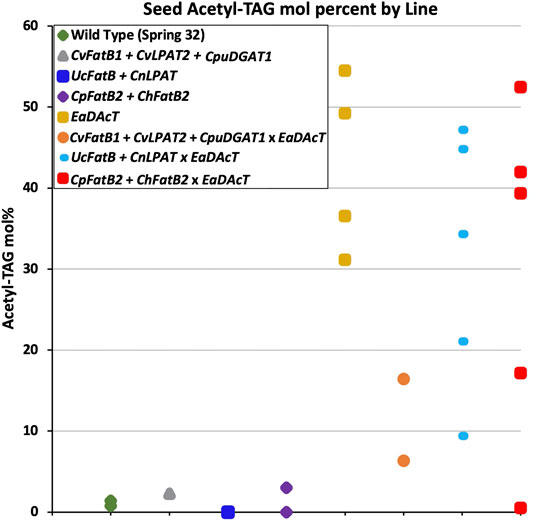
FIGURE 6. Comparison of F3 seed acetyl-TAG amounts in lines generated by crossing a pennycress transgenic EaDAcT line with transgenic lines harboring one of three MCFA biosynthetic genes combinations.
We also quantified the fatty acid composition of the TAG and acetyl-TAG components of the seed oil from the two genotypes (UcFatB + CnLPAT × EaDAcT and CpFatB2 + ChFatB2 × EaDAcT) that were capable of producing high levels of acetyl-TAG. For lines expressing CpFatB2 + ChFatB2, acetyl-TAG contained lower levels of MCFA shorter than 14:0 compared to TAG. However, at least one line appeared to incorporate higher levels of 14:0 into acetyl-TAG (Figure 7A). In contrast, seed that combined expression of UcFatB + CnLPAT with EaDAcT tended to contain higher levels of 8:0 and 12:0 in acetyl-TAG compared to lcTAG (Figure 7B). The accumulation of these particular fatty acids in these genotypes is similar to results obtained generating acetyl-TAG with MCFA in camelina (Bansal et al., 2018).
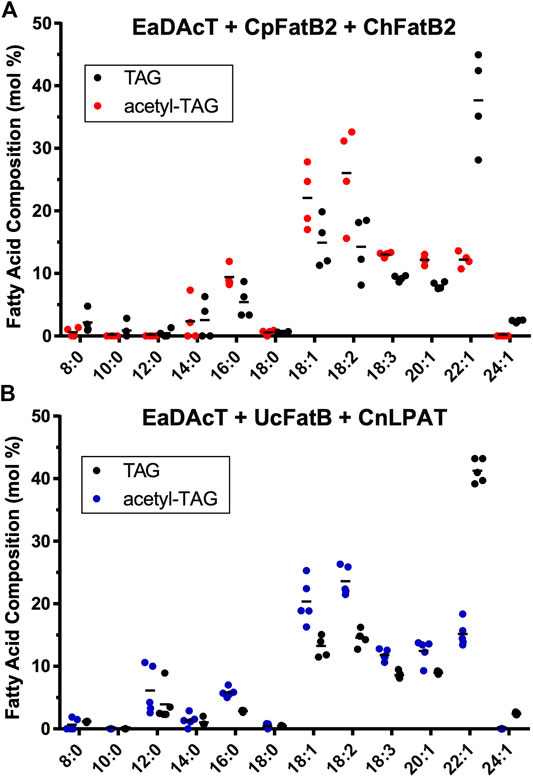
FIGURE 7. Fatty acid composition of acetyl-TAG and TAG extracted from F3 seeds of lines expressing EaDAcT in combination with (A)CpFatB2 + ChFatB2 or (B)UcFatB + CnLPAT. Horizontal lines represent the mean value.
Consistent with previous studies expressing EaDAcT in pennycress (McGinn et al., 2019), acetyl-TAG from these transgenic lines contained higher levels of 16:0, 18:1, 18:2, 18:3, and 20:1. In contrast, the VLCFA 22:1 was found at much higher levels in TAG compared to acetyl-TAG (Figure 7). The higher level of 22:1 in TAG is likely due to preferential incorporation at the sn-3 position of the molecule, as is the case for VLCFA in other Brassicaceae (Takagi and Ando, 1991; Taylor et al., 1995). In acetyl-TAG though, the sn-3 position is occupied by the acetate group, making it unavailable for the incorporation of 22:1.
To further confirm the incorporation of MCFA into acetyl-TAG, we used ESI-MS to analyze the molecular species composition of the neutral lipids extracted from the seeds from these lines. Seeds expressing MCFA biosynthetic genes in combination with EaDAcT produced many additional molecular species of TAGs and acetyl-TAGs, consistent with the availability of a wider range of fatty acids to incorporate into TAG (Figure 8). For example, the mass spectrum of neutral lipids from CpFatB2 + ChFatB2 × EaDAcT possesses a novel peak with an m/z value of 626.6 which most likely corresponds to an acetyl-TAG molecule that contains 14:0 and 18:1 in addition to the sn-3 acetate (34:1 in Figure 8). The presence of this particular molecular species is consistent with the higher accumulation of 14:0 in the acetyl-TAG from this gene combination (Figure 7A). Likewise, the mass spectrum of UcFatB + CnLPAT × EaDAcT contained additional novel molecular species corresponding to the incorporation of either 12:0 or 14:0 in acetyl-TAGs, again consistent with the fatty acid composition of the acetyl-TAG fraction (Figure 7B). The masses of these novel molecular species also indicate that the incorporated acyl groups possess one double bond among them (32:1 and 34:1) or even two (32:2 and 34:2). As the MCFA synthesized in these lines are saturated (Figure 7), this observation suggests that only one MCFA is being incorporated into the acetyl-TAG molecules. Biochemical characterization of EaDAcT substrate specificity has demonstrated that the enzyme prefers more unsaturated DAG substrates (Bansal and Durrett, 2016), possibly explaining the absence of acetyl-TAG possessing two MCFA.
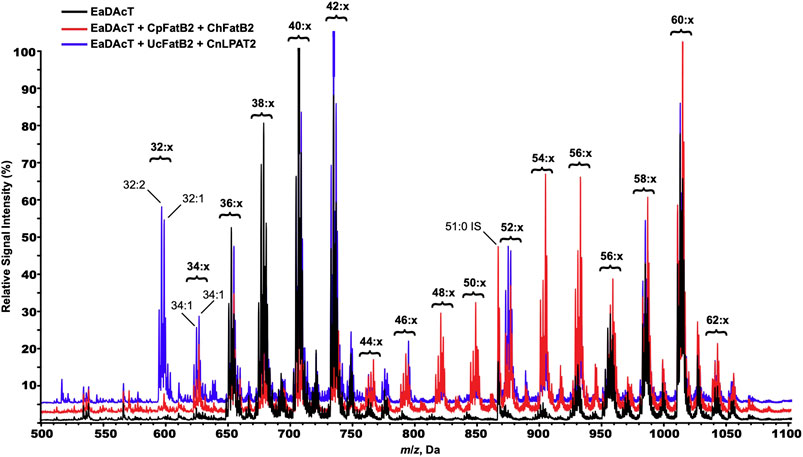
FIGURE 8. ESI-MS spectra of neutral lipids extracted from F3 seeds of representative lines expressing EaDAcT by itself (black), or in combination with CpFatB2 + ChFatB2 (red) or UcFatB + CnLPAT (blue). Signal peaks possess the m/z value of the [M + NH4]+ adduct. For clarity, only the number of acyl carbons and not the number of double bonds (x) in each series of acetyl-TAG and TAG molecular species is indicated.
Conclusion
This study shows that MCFA production at modest levels can be engineered into Thlaspi arvense (pennycress) TAGs and acetyl-TAGs. For the most part, our data show that seed-specific MCFA production was tolerated, although there were signs that embryo health may be affected based on delays in seed germination. It seems likely that these delays and even seed inviability will become problematic with the engineering of higher MCFA production. However, Cuphea and other plant species have evolved to accumulate over 90 mol% MCFAs in TAGs, suggesting that higher MCFA accumulation in pennycress seed TAGs may also be possible without compromising plant and embryo health. How Cuphea plant species successfully accumulate such high levels of MCFAs remains unclear. For example, work remains in determining if Cuphea species effectively target MCFAs solely to TAGs thereby avoiding their incorporation into cellular membranes whose function could be compromised. Work also remains in determining how efficiently MCFAs are being incorporated into pennycress TAGs, as free fatty acids can be toxic to cells and tissues (Yang et al., 2015; Yang and Benning, 2018).
From an economic perspective, the production of MCFA-containing TAGs and acetyl-TAGs in pennycress seeds may be commercially challenging for biofuels production, given the significant decreases in seed oil amounts observed in the different MCFA-producing lines. Techno-economic analyses will be necessary to determine if, even at reduced oil production levels, pennycress production of MCFAs could be economical for, e.g., more lucrative uses such as in consumer products including cosmetics. Regardless, a focus on engineering and/or breeding for increased total seed oil production would be useful, such as by increasing synthesis of the glycerol backbone to which FAs become acylated, which is an approach showing some promise (Chhikara et al., 2018).
Data Availability Statement
The raw data supporting the conclusions of this article will be made available by the authors, without undue reservation.
Author Contributions
ME generated and managed the transgenic pennycress lines. ME and MF performed seed germination and growth studies. TN and GM performed lipids analyses while MP, ME, and WP performed total oil analyses. All authors analyzed the data. ME, TD, EC, and JS designed and interpreted experiments. ME, MF, TN, MP, WP, EC, TD, and JS wrote and edited the manuscript.
Funding
This material is based upon work that is supported by the National Institute of Food and Agriculture, U.S. Department of Agriculture, under award numbers 2014-67009-22305 and 2018-67009-27374 to JCS. TPD was funded by an award from USDA-NIFA (No. 2015-67013-22815). EBC was funded by grants from USDA-NIFA (Nos: 2018-38821-28111 and 2019-67021-29946).
Conflict of Interest
The authors declare that the research was conducted in the absence of any commercial or financial relationships that could be construed as a potential conflict of interest.
Acknowledgments
We thank Michaela McGinn and Brice Jarvis for designing primers, providing germplasm and for fruitful conversations, Jennifer Woodworth, Kaitlin Janowiak, Taylor Suo, Katy Haag, Nick Bretz, and Ashley King for helping manage plant populations and assisting with phenotypic and genotypic analyses, and Pam Tamura for ESI-MS analysis of TAG samples.
Supplementary Material
The Supplementary Material for this article can be found online at: https://www.frontiersin.org/articles/10.3389/fenrg.2021.620118/full#supplementary-material
References
Altendorf, K., Isbell, T., Wyse, D. L., and Anderson, J. A. (2019). Significant variation for seed oil content, fatty acid profile, and seed weight in natural populations of field pennycress (Thlaspi arvense L.). Ind. Crop. Prod. 129, 261–268. doi:10.1016/j.indcrop.2018.11.054
Bansal, S., and Durrett, T. P. (2016). Rapid quantification of low-viscosity acetyl-triacylglycerols using electrospray ionization mass spectrometry. Lipids 51, 1093–1102. doi:10.1007/s11745-016-4179-0
Bansal, S., Kim, H. J., Na, G., Hamilton, M. E., Cahoon, E. B., Lu, C., et al. (2018). Towards the synthetic design of camelina oil enriched in tailored acetyl-triacylglycerols with medium-chain fatty acids. J. Exp. Bot. 69, 4395–4402. doi:10.1093/jxb/ery225
Carlsson, A. S., Yilmaz, J. L., Green, A. G., Stymne, S., and Hofvander, P. (2011). Replacing fossil oil with fresh oil—with what and for what? Eur. J. Lipid Sci. Technol. 113, 812–831. doi:10.1002/ejlt.201100032
Chapman, K. D., and Ohlrogge, J. B. (2012). Compartmentation of triacylglycerol accumulation in plants. J. Biol. Chem. 287, 2288–2294. doi:10.1074/jbc.R111.290072
Chhikara, S., Abdullah, H. M., Akbari, P., Schnell, D., and Dhankher, O. P. (2018). Engineering Camelina sativa (L.) Crantz for enhanced oil and seed yields by combining diacylglycerol acyltransferase1 and glycerol-3-phosphate dehydrogenase expression. Plant Biotechnol. J. 16, 1034–1045. doi:10.1111/pbi.12847
Chopra, R., Johnson, E. B., Daniels, E., McGinn, M., Dorn, K. M., Esfahanian, M., et al. (2018). Translational genomics using Arabidopsis as a model enables the characterization of pennycress genes through forward and reverse genetics. Plant J. 96, 1093–1105. doi:10.1111/tpj.14147
Chopra, R., Johnson, E. B., Emenecker, R., Cahoon, E. B., Lyons, J., Kliebenstein, D. J., et al. (2020). Identification and stacking of crucial traits required for the domestication of pennycress. Nat. Food 1, 84–91. doi:10.1038/s43016-019-0007-z
Dehesh, K., Jones, A., Knutzon, D. S., and Voelker, T. A. (1996). Production of high levels of 8:0 and 10:0 fatty acids in transgenic canola by overexpression of Ch FatB2, a thioesterase cDNA from Cuphea hookeriana. Plant J. 9, 167–172. doi:10.1046/j.1365-313x.1996.09020167.x
Dorn, K. M., Fankhauser, J. D., Wyse, D. L., and Marks, M. D. (2015). A draft genome of field pennycress (Thlaspi arvense) provides tools for the domestication of a new winter biofuel crop. DNA Res. 22, 121–131. doi:10.1093/dnares/dsu045
Doyle, J., and Doyle, J. L. (1987). Genomic plant DNA preparation from fresh tissue‐CTAB method. Phytochem. Bull. 19, 11–15.
Drenth, A. C., Olsen, D. B., and Denef, K. (2015). Fuel property quantification of triglyceride blends with an emphasis on industrial oilseeds camelina, carinata, and pennycress. Fuel 153, 19–30. doi:10.1016/j.fuel.2015.02.090
Durrett, T. P., McClosky, D. D., Tumaney, A. W., Elzinga, D. A., Ohlrogge, J., and Pollard, M. (2010). A distinct DGAT with sn-3 acetyltransferase activity that synthesizes unusual, reduced-viscosity oils in Euonymus and transgenic seeds. Proc. Natl. Acad. Sci. U.S.A. 107, 9464–9469. doi:10.1073/pnas.1001707107
Graham, S. A., and Kleiman, R. (1992). Composition of seed oils in some Latin American Cuphea (Lythraceae). Ind. Crop. Prod. 1, 31–34. doi:10.1016/0926-6690(92)90042-t
Isbell, T. A. (2009). US effort in the development of new crops (Lesquerella, Pennycress Coriander and Cuphea). OCL 16, 205–210. doi:10.1051/ocl.2009.0269
Isbell, T. A., Evangelista, R., Glenn, S. E., Devore, D. A., Moser, B. R., Cermak, S. C., et al. (2015). Enrichment of erucic acid from pennycress (Thlaspi arvense L.) seed oil. Ind. Crop. Prod. 66, 188–193. doi:10.1016/j.indcrop.2014.12.050
Iskandarov, U., Silva, J. E., Kim, H. J., Andersson, M., Cahoon, R. E., Mockaitis, K., et al. (2017). A specialized diacylglycerol acyltransferase contributes to the extreme medium-chain fatty acid content of Cuphea seed oil. Plant Physiol. 174, 97–109. doi:10.1104/pp.16.01894
Kallio, P., Pásztor, A., Akhtar, M. K., and Jones, P. R. (2014). Renewable jet fuel. Curr. Opin. Biotechnol. 26, 50–55. doi:10.1016/j.copbio.2013.09.006
Kim, H. J., Silva, J. E., Iskandarov, U., Andersson, M., Cahoon, R. E., Mockaitis, K., et al. (2015a). Structurally divergent lysophosphatidic acid acyltransferases with high selectivity for saturated medium chain fatty acids from Cuphea seeds. Plant J. 84, 1021–1033. doi:10.1111/tpj.13063
Kim, H. J., Silva, J. E., Vu, H. S., Mockaitis, K., Nam, J. W., and Cahoon, E. B. (2015b). Toward production of jet fuel functionality in oilseeds: identification of FatB acyl-acyl carrier protein thioesterases and evaluation of combinatorial expression strategies in Camelina seeds. J. Exp. Bot. 66, 4251–4265. doi:10.1093/jxb/erv225
Knaut, J., and Richtler, H. J. (1985). Trends in industrial uses of palm and lauric oils. J. Am. Oil Chem. Soc. 62, 317–327. doi:10.1007/bf02541398
Knothe, G., Cermak, S. C., and Evangelista, R. L. (2009). Cuphea oil as source of biodiesel with improved fuel properties caused by high content of methyl decanoate†. Energy Fuels 23, 1743–1747. doi:10.1021/ef800958t
Knutzon, D. S., Hayes, T. R., Wyrick, A., Xiong, H., Maelor Davies, H. H., and Voelker, T. A. (1999). Lysophosphatidic acid acyltransferase from coconut endosperm mediates the insertion of laurate at the sn-2 position of triacylglycerols in lauric rapeseed oil and can increase total laurate levels. Plant Physiol. 120, 739–746. doi:10.1104/pp.120.3.739
Li, Y., Beisson, F., Pollard, M., and Ohlrogge, J. (2006). Oil content of Arabidopsis seeds: the influence of seed anatomy, light and plant-to-plant variation. Phytochemistry 67, 904–915. doi:10.1016/j.phytochem.2006.02.015
Liu, J., Rice, A., McGlew, K., Shaw, V., Park, H., Clemente, T., et al. (2015a). Metabolic engineering of oilseed crops to produce high levels of novel acetyl glyceride oils with reduced viscosity, freezing point and calorific value. Plant Biotechnol. J. 13, 858–865. doi:10.1111/pbi.12325
Liu, J., Tjellström, H., McGlew, K., Shaw, V., Rice, A., Simpson, J., et al. (2015b). Field production, purification and analysis of high-oleic acetyl-triacylglycerols from transgenic Camelina sativa. Ind. Crop. Prod. 65, 259–268. doi:10.1016/j.indcrop.2014.11.019
Mazareanu, E. (2020). Commercial airlines worldwide fuel consumption 2005–2021. Available at: https://www.statista.com/statistics/655057/fuel-consumption-of-airlines-worldwide/.
McGinn, M., Phippen, W. B., Chopra, R., Bansal, S., Jarvis, B. A., Phippen, M. E., et al. (2019). Molecular tools enabling pennycress (Thlaspi arvense) as a model plant and oilseed cash cover crop. Plant Biotechnol. J. 17, 776–788. doi:10.1111/pbi.13014
Morgan, N. (2014). Aviation biofuels: getting ready for take-off. Biofuels Bioprod. Bioref. 8, 739–742. doi:10.1002/bbb.1527
Moser, B. R. (2012). Biodiesel from alternative oilseed feedstocks: camelina and field pennycress. Biofuels 3, 193–209. doi:10.4155/bfs.12.6
Moser, B. R., Knothe, G., Vaughn, S. F., and Isbell, T. A. (2009). Production and evaluation of biodiesel from field pennycress (Thlaspi arvense L.) oil†. Energy Fuels 23, 4149–4155. doi:10.1021/ef900337g
Murashige, T., and Skoog, F. (1962). A revised medium for rapid growth and bio assays with tobacco tissue cultures. Physiol. Plantarum 15, 473–497. doi:10.1111/j.1399-3054.1962.tb08052.x
Murphy, D. J., and Cummins, I. (1989). Biosynthesis of seed storage products during embryogenesis in rapeseed, Brassica napus. J. Plant Physiol. 135, 63–69. doi:10.1016/s0176-1617(89)80225-1
Nieschlag, H. J., and Wolff, I. A. (1971). Industrial uses of high erucic oils. J. Am. Oil Chem. Soc. 48, 723–727. doi:10.1007/bf02638529
Pollard, M. R., Anderson, L., Fan, C., Hawkins, D. J., and Davies, H. M. (1991). A specific acyl-ACP thioesterase implicated in medium-chain fatty acid production in immature cotyledons of Umbellularia californica. Arch. Biochem. Biophys. 284, 306–312. doi:10.1016/0003-9861(91)90300-8
Ringbeck, J., and Koch, V. (2010). Aviation biofuels: a roadmap towards more carbon-neutral skies. Biofuels 1, 519–521. doi:10.4155/bfs.10.35
Sedbrook, J. C., and Durrett, T. P. (2020). Pennycress, carbon wise: labeling experiments reveal how pennycress seeds efficiently incorporate carbon into biomass. J. Exp. Bot. 71, 2842–2846. doi:10.1093/jxb/eraa136
Sedbrook, J. C., Phippen, W. B., and Marks, M. D. (2014). New approaches to facilitate rapid domestication of a wild plant to an oilseed crop: example pennycress (Thlaspi arvense L.). Plant Sci. 227, 122–132. doi:10.1016/j.plantsci.2014.07.008
Sindelar, A. J., Schmer, M. R., Gesch, R. W., Forcella, F., Eberle, C. A., Thom, M. D., et al. (2017). Winter oilseed production for biofuel in the US Corn Belt: opportunities and limitations. GCB Bioenergy 9, 508–524. doi:10.1111/gcbb.12297
Takagi, T., and Ando, Y. (1991). Stereospecific analysis of triacyl-sn-glycerols by chiral high-performance liquid chromatography. Lipids 26, 542–547. doi:10.1007/bf02536601
Tao, L., Milbrandt, A., Zhang, Y., and Wang, W. C. (2017). Techno-economic and resource analysis of hydroprocessed renewable jet fuel. Biotechnol. Biofuels 10, 261. doi:10.1186/s13068-017-0945-3
Taylor, D. C., Giblin, E. M., Reed, D. W., and Hogge, L. R. (1995). Stereospecific analysis and mass spectrometry of triacylglycerols fromarabidopsis thaliana (L.) heynh. columbia seed. J. Am. Oil Chem. Soc. 72, 305–308. doi:10.1007/bf02541087
Tjellström, H., Strawsine, M., Silva, J., Cahoon, E. B., and Ohlrogge, J. B. (2013). Disruption of plastid acyl:acyl carrier protein synthetases increases medium chain fatty acid accumulation in seeds of transgenic Arabidopsis. FEBS Lett. 587, 936–942. doi:10.1016/j.febslet.2013.02.021
Yang, Y., and Benning, C. (2018). Functions of triacylglycerols during plant development and stress. Curr. Opin. Biotechnol. 49, 191–198. doi:10.1016/j.copbio.2017.09.003
Keywords: acetyl-TAG, acetyl-triacylglycerol, medium chain fatty acids, pennycress, TAG, Thlaspi arvense, triacylglycerol
Citation: Esfahanian M, Nazarenus TJ, Freund MM, McIntosh G, Phippen WB, Phippen ME, Durrett TP, Cahoon EB and Sedbrook JC (2021) Generating Pennycress (Thlaspi arvense) Seed Triacylglycerols and Acetyl-Triacylglycerols Containing Medium-Chain Fatty Acids. Front. Energy Res. 10:620118. doi: 10.3389/fenrg.2021.620118
Received: 22 October 2020; Accepted: 04 January 2021;
Published: 28 January 2021.
Edited by:
Subba Rao Chaganti, University of Michigan, United StatesReviewed by:
Balamurugan Srinivasan, Bharathidasan University, IndiaMuhammad Farooq, University of Engineering and Technology, Lahore, Pakistan
Copyright © 2021 Esfahanian, Nazarenus, Freund, Mcintosh, Phippen, Phippen, Durrett, Cahoon and Sedbrook. This is an open-access article distributed under the terms of the Creative Commons Attribution License (CC BY). The use, distribution or reproduction in other forums is permitted, provided the original author(s) and the copyright owner(s) are credited and that the original publication in this journal is cited, in accordance with accepted academic practice. No use, distribution or reproduction is permitted which does not comply with these terms.
*Correspondence: John C. Sedbrook, amNzZWRickBpbHN0dS5lZHU=