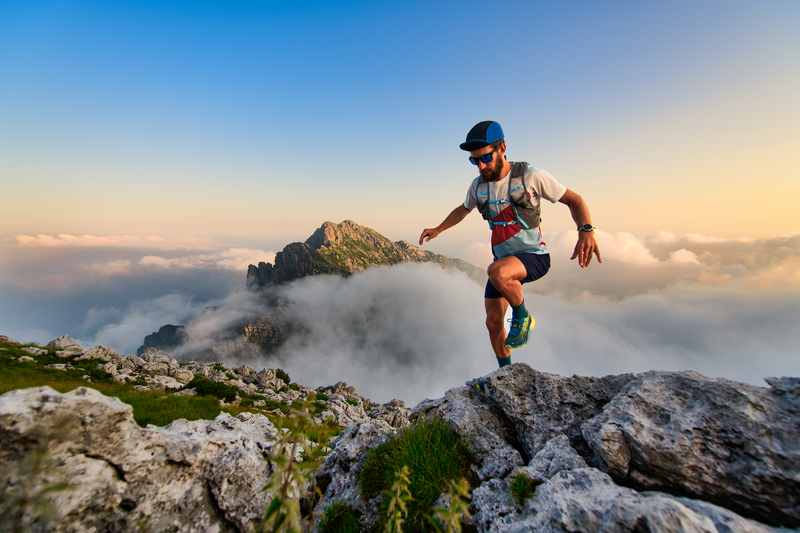
95% of researchers rate our articles as excellent or good
Learn more about the work of our research integrity team to safeguard the quality of each article we publish.
Find out more
REVIEW article
Front. Energy Res. , 17 December 2020
Sec. Bioenergy and Biofuels
Volume 8 - 2020 | https://doi.org/10.3389/fenrg.2020.598803
This article is part of the Research Topic Plant Seed Oils and Their Potential for Biofuel Production View all 8 articles
The microalgal biotechnology industry is expanding rapidly and currently gaining attention due to multiple availabilities of high-value products such as pigments, carbohydrates, proteins, nutraceuticals, biopharmaceuticals, and unique oleaginous compounds fractionated by biomass biorefinery. Microalgae are efficient primary producers in the terrestrial and marine biotopes. They are major sources of global oxygen and are gaining topical prominence due to their concomitant role in the phycoremediation of wastewater effluents and biomass production. Despite their minuscule size, microalgae critically contribute to climate change mitigation through carbon fixation and play a major role in bioenergy applications. Furthermore, carotenoids and phycobiliproteins are the main accessory light-harvesting complexes in microalgae and cyanobacteria. The topical biomedical and pharmaceutical applications of microalgae include anticancer, antidiabetic, antiHIV, antimalarial, antimicrobial, inter alia. The endowment of unique indigenous microalgae and utilization of these biological resources must be harnessed by the biorefinery industry to exploit microalgal biomass opportunities. Therefore, this manuscript factually and critically explores the current status of the biorefinery approach, topical biomedical and pharmaceutical applications, biofuel applications, genetic manipulation of microalgae for enhancement of product yield, challenges and presents prospects, pros and cons, and outlook of the microalgal biotechnology industry.
Microalgae are natural biofactories that are rapidly gaining topical prominence due to their long-term sustainable and versatile applications as food and feed, for biochemical and bioenergy production and in the mitigation of global climate change (Ratnapuram et al., 2018; Vu et al., 2018). Microalgal biomass is a natural food source for many important aquaculture organisms such as mollusks, shrimps, and fish (Selvarajan et al., 2015). The microalgal biotechnology industry has received significant attention in recent years owing to biorefinery whereby a variety of products e.g., chlorophylls, carotenoids, carbohydrates, lipids, proteins, nucleic acids and nutraceuticals inter alia, are generated from the same harvested microalgal biomass within a few days (Vu et al., 2018; Wang et al., 2018; Nur and Buma, 2019). These high-value products are synthesized by numerous microalgae as storage components as well as functional compounds and have applications in the bioenergy and pharmaceutical industries (Figueroa-Torres et al., 2017). Currently, the production of omega-3 fatty acids, carotenoids, and phycobiliproteins (PBPs) from microalgal biomass is receiving a lot of attention due to their biomedical applications such as potential cancer treatment agents. High costs and limited biomass productivity associated with microalgal bioprocessing are significantly constraining economic competitiveness and restricting the industrialization of microalgal biotechnology, hence high-value products are proposed for microalgal biorefinery (Banu et al., 2020; Bhatia et al., 2021). Since microalgal biodiesel production is currently not yet economically viable, it is desirable to also explore the potential applications of the unique microalgal intracellular biomolecules and compounds (Wang et al., 2018). Besides, a direct substantial reduction of the production costs of microalgal lipids can be achieved by combining lipid production with other applications e.g., extraction of high-value metabolites and also CO2 sequestration (Lee et al., 2018).
The microalgal biotechnology industry encompasses a whole range of activities such as upstream processes (bioprospecting of superior microalgal strains, isolation and strain purification, strain selection for specific purposes, cultivation systems, biomass harvesting) and downstream processes (microalgal disruption techniques and intracellular metabolite extraction, biochemical transformations and product recovery). High-throughput screening of the microalgal strains producing large amounts of the desired metabolite e.g., neutral lipids, starch, proteins, carotenoids, etc. is mandatory (Delrue et al., 2016; Gumbi et al., 2017). Therefore, it is crucial to select superior, robust, and nonfastidious microalgal strains for specific applications for the whole process to succeed. Furthermore, the biomass cultivation strategy must be optimized for the optimal production of the target metabolite (Lee et al., 2018). For optimal growth and metabolite accumulation, the following physico-chemical factors must be strictly controlled such as temperature, pH, nutrients, light quality and intensity, agitation, CO2 supply, photoperiod, etc. These factors are microalgal strain specific. Meticulous controlling of the key microalgal growth factors for optimal production of high value biomolecules such as carotenoids, special oils and biomass can be achieved through experimental designs involving one-factor-at-a-time-approach or through statistical modeling such as response surface methodology (RSM) (Thiruvenkadam et al., 2018). However, the former approach is tedious and time-consuming while the latter approach is proximate and can lead to unfounded errors in the selection and combination of variables.
A variety of high-value products, with potential biotechnological applications, can be extracted from microalgal biomass. The valuable products are mainly pigments (chlorophylls and carotenoids), PBPs, fatty acids, carbohydrates, vitamins, nutraceuticals, etc. These valuable compounds can be extracted from the microalgal biomass using the biorefinery approach. The synergistic and overlapping combination and interaction of various applications (e.g., food, medicine, wastewater treatment, and flue gas treatment) with biofuel production could enhance the sustainability and economics of the algal biofuel production system (Zhang et al., 2014).
The imminent shortage of fossil fuels due to the depletion of petroleum oil reserves is going to cause a major economic challenge in the current context characterized by high increasing energy demand. The industrial revolution cannot be possible without sustainable energy. Therefore, renewable energy resources are considered as an appropriate alternative to compete with the decreasing fossil led sources of energy. Currently, bioenergy endeavors are rapidly gaining importance and some applications are showing promising results. In the case of microalgae based fuels, many studies and applications have unequivocally demonstrated the opportunity that algae bio-oil presents in producing biodiesel and bio-jet fuel. Although there are some pertinent challenges related to lipid content for some microalgae strains, the quality of the final product is generally complying with the recommended standards. The generated algae biodiesel is known to have low carbon emissions with heat content that is not different from conventional diesel. Cost issues may be fixed by choosing relevant upstream and downstream strategies. For upstream processes, highly productive strains, relevant genetic engineering and metabolic engineering techniques should be selected and applied. For downstream processes, the aim is to generate high yields for biodiesel by increasing the strain’s lipid content and by improving the biodiesel production output from the conversion of microalgal biomass to biodiesel. To generate high biomass and high biodiesel production a two-stage culture strategy can be implemented as a win-win approach to solve the competition between cell growth, biomass accumulation and lipid increase. The first step can be the cell growth and biomass accumulation and the second can be the physiological modification to trigger the increase of lipid content by nutrients starvation or any other process. With regard to the aviation industry microalgae based jet fuel is perceived as a milestone that will assist in the reduction of carbon emissions, improve the aircraft fuel efficiency and better air traffic control to promote safe, efficient and sustainable air travel.
Many options or technologies to produce microalgae-based jet fuel technology exist but most of them have not reached the maturity level for commercialization (Bwapwa et al., 2019). This is due to the low lipid content for many microalgae strains, the operating costs related to cultivation and harvesting of microalgae biomass and the costs of some conversion processes and their complexity (Bwapwa et al., 2019). However, this situation is an opportunity for continued research and development in innovative bio jet fuel technologies. Therefore, the main aim of this review manuscript is to provide a succinct critique and synopsis of the current status of the biorefinery concept, jet fuel production, value-added products generated by the microalgal biotechnology, biomedical and pharmaceutical applications, current challenges and future prospects of the microalgal biotechnology industry.
Microalgae are ubiquitous eukaryotic photosynthetic microorganisms that are found in diverse normal and extreme aquatic habitats such as freshwater, lacustrine, soda lakes, riverine, marine, estuarine, brackish, thermophilic, saline and hyper-saline environments (Leliaert et al., 2012). Hence, microalgae can be found in almost all ecosystems (Selvarajan et al., 2015). However, cyanobacteria are blue-green photosynthetic prokaryotic microorganisms that are also included in this broad circumscription (Lee et al., 2014). Microalgae constitute important primary producers in nature and form the basis of the food chain in aquatic environments (Malapascua et al., 2014). With an estimated 200,000 to several million different strains, microalgae have immense biodiversity as compared to terrestrial higher plants with approximately 250,000 members only (Delrue et al., 2016). To date, less than 10,000 microalgal strains have been described and hence, there is a huge potential for this untapped and unexplored broad bioresource for potential biotechnological applications (Delrue et al., 2016). Taxonomically, algae are broadly classified as Rhodophyta (red algae), Phaeophyta (brown algae), and Chlorophyta (green algae) and grouped according to their size as macroalgae or microalgae (Khan et al., 2018). The macroalgae (seaweeds) are multicellular and large-size algae that are visible to the naked eye, while microalgae are microscopic single cells and may be prokaryotic (cyanobacteria) or eukaryotic, e.g., green algae (Chlorophyta).
Microalgae are diverse micro-eukaryotic entities that produce a variety of metabolites under photoautotrophic, heterotrophic and mixotrophic growth conditions. Autochthonous marine and freshwater microalgae are an extremely heterogeneous group of oligotrophic organisms with minimum nutrient requirements hence they can thrive in any natural environment (Vu et al., 2018). Depending on the species, their sizes can range from a few micrometers (µm) to a few hundreds of micrometers (Suganya et al., 2016). The growth kinetics of microalgae vary due to prevailing key growth controlling factors. Microalgae are desirable candidates for high-value metabolite production due to their fast growth rates as compared to some terrestrial plants such as jatropha and coconut (Kirrolia et al., 2013). By focusing on oil, microalgae are reported to produce 58,700 L/ha as compared to corn (172 L/ha), soybean (446 L/ha), jatropha (1892 L/ha), coconut (2689 L/ha), and palm (5950 L/ha) (Chisti, 2007). Microalgae are carbon neutral and therefore serve as better candidates for climate change mitigation. Therefore, microalgae can serve as suitable and attractive candidates as biofactories for the production of a wide range of compounds. According to Hildebrand et al. (2013), microalgal productivity is dependent on the efficiency of carbon fixation and the downstream cellular processes that convert photosynthate into useful precursors and final metabolites.
The microalgal biotechnology industry is currently booming due to food security concerns since microalgal cultivation and metabolite extraction does not interfere with food security. Microalgal cultivation does not require large tracks of arable land as compared to some crops such as maize, sunflower, cotton, and soybean. Microalgal cultivation does not necessarily require copious volumes of freshwater resources. Microalgal systems have a higher photon conversion efficiency, can be harvested batch-wize nearly all-year-round, can utilize salt and wastewater streams, can couple CO2-neutral fuel production with CO2 sequestration and produce non-toxic and highly biodegradable biofuels (Cobos et al., 2017). Wastewater streams such as municipal, domestic, agricultural wastewaters can be coupled and harnessed for microalgal cultivation as long as there are sufficient concentrations of nitrates, phosphates and trace elements (Zhou et al., 2014). The search for superior and unique microalgal strains is an ongoing exercise and currently bioprospecting for indigenous strains is an intense activity among research communities. However, the major drawback is the apparent low yield of desirable metabolites e.g., pigments and neutral lipids. The low yield of the desirable metabolites can be overcome by carefully manipulating environmental stresses and optimizing growth conditions for maximal product yield (Chen et al., 2017).
Biorefinery is defined as the fractionation of the harvested pre-treated biomass into a variety of useful compounds by applying biochemical processes and is reported to be cost-effective as compared to the sole production of biofuel which fails to meet current market requirements (Moreno-Garcia et al., 2017). Biorefinery allows for the extraction of multiple types of metabolites from a single microalgal biomass making it the most attractive approach for successful establishment of the microalgal biotechnology industry and could be the most sustainable and economically viable approach for the generation of microalgal biomass (Rawat et al., 2011). The biorefinery approach is schematically illustrated in Figure 1. According to Zhu (2015), biorefinery is an industrial process, where biomass is converted into a range of biochemicals, materials and energy products and this concept is analogous to the current oil refinery, where multiple fuels and products are realized from fossil oil. Also, according to Suganya et al. (2016), the term “biorefinery” was coined to describe the production of biofuels as well as high-value co-products from biomass by the integration of bioprocessing and appropriate low environmental impacting chemical technologies in a cost-effective and environmentally sustainable manner.
A suitable microalgal biomass propagation technique is necessary to generate sufficient biomass for the extraction of the desired end products. There are two commonly used methods for the cultivation of microalgae, namely, the open raceway pond system and the closed photobioreactor system (Brennan and Owende, 2010). The open raceway system involves the cultivation of microalgae in circular oblong channels and shallow recirculating ponds with semicircular ends with deflector baffles ensuring uniformity of flow and minimizing the formation of dead zones (Chisti, 2016). Open raceway ponds can be cascading or other performance designs and configurations with a central wall or baffle. In the open raceway cultivation system, recirculation, flow, and mixing are typically generated by a single slowly rotating paddle wheel. The two microalgal cultivation methods are associated with inherent advantages and disadvantages. The open raceway ponds are widely used for the commercial production of microalgal biomass. Though open raceway ponds are generally effective and inexpensive, they suffer from relatively low productivity and exposed to vagaries of weather as well as constant contamination (Chisti, 2016). To date, raceway ponds remain the microalgal biomass production system of choice despite their low productivity since they require a relatively low investment in capital (Zhou et al., 2014; Chisti, 2016). Earthrize Nutritionals produces Spirulina using the raceway pond system. The closed photobioreactor system is a cultivation system in which all growth factors such as CO2, light intensity, pH, axenicity, etc. are properly controlled and the microalgal suspension is not prone to contamination since it is enclosed. The closed photobioreactors (PBRs) can be placed outdoors or inside buildings with sufficient illumination. However, the photobioreactor cultivation system suffers from being capital and power-intensive, therefore, constraining its economic competitiveness. However, the main advantage of the photobioreactor cultivation system is fairly high biomass productivity which surpasses that of the open raceway ponds. The technical viability of each system is influenced by intrinsic characteristics of the selected microalgal strain, prevailing regional climatic conditions and the costs of the available land and water (Brennan and Owende, 2010).
The success of the biorefinery process depends largely upon appropriate and careful selection of microalgal strains for the production of target compounds (Mutanda et al., 2011; Gumbi et al., 2017; Gifuni et al., 2018). According to Wilkie et al. (2011), specific criteria of selection for the production of biofuels from indigenous microalgae should encompass biomass productivity, lipid productivity, harvestability of the microorganism, and oil extractability. The key phrases for successful microalgal biomass biorefinery of target compounds involve a series of sequential steps (Figure 2).
FIGURE 2. Major phases involved for successful biorefinery of microalgal biomass (Kim et al., 2018).
The major thrust of the biorefinery process is to effectively separate and recover the target metabolites (e.g., lipids, carbohydrates, proteins, and pigments) from the same batch of microalgal biomass, therefore significantly reducing the production costs (Kim et al., 2018). Therefore, in order to select superior target microalgae, it is crucial to embark on a rigorous high-throughput screening exercise so as to accurately identify and select the best performing microalgal strains (Pereira et al., 2018). The crucial question to ask is: are microalgal strains from culture collection centers more suitable and superior than the indigenous strains from the local aquatic habitats? Hence, it is desirable to bioprospect, assess and evaluate the indigenous microalgal strains that are already acclimatized to the local climatic conditions and biota especially if outdoor cultivation is envisaged. Besides, the exploitation of microalgal strains from local habitats has been demonstrated to ensure dominance and high adaptability to local environmental and climatic conditions and should be the preferred option to prevent the invasion of non-indigenous species in the local environment (Duong et al., 2015; Thao et al., 2017). Indigenous microalgal strains, however, require isolation, the establishment of optimal culturing conditions, and determination of the feasibility of production by experimental techniques and this is time-consuming, labor intensive and is costlier than strain selection from culture collection centers (Rawat et al., 2013). Table 1 depicts the pros and cons of microalgal strains sourced from local natural habitats as compared to culture collection centers.
TABLE 1. Advantage and disadvantages of microalgal strains obtained from indigenous natural aquatic habitats compared to other culture sources (Wilkie et al., 2011).
The isolation and purification of microalgal strains from diverse aquatic and terrestrial habitats are associated with several challenges such as poor growth on solid agar media, and non-culturability in artificial media. The strains that are purchased from culture collection centers can be used as reference strains especially for screening microalgal strains producing desirable metabolites. Microalgal strains can be purchased from various reputable and specialized culture collection repositories around the world such as Culture Collection of Algae at The University of Texas (UTEX, United States), Australian National Algae Culture Collection (ANACC, Australia), The Culture Collection of Algae and Protozoa (CCAP, United Kingdom), Microbial Culture Collection at the National Institute for Environmental Studies (NIES Collection, Tsukuba, Japan), The Sammlung von Algenkulturen der Universität Göttingen (Culture Collection of Algae at Göttingen University, (SAG, Germany), Canadian Phycological Culture Collection (CPCC, Canada), etc. The main disadvantage of sourcing microalgal cultures from these centers is that any deviation from the strict storage conditions can lead to inactivation or death of the microalgal cultures. Besides, the cultures sourced from these centers have strict nutritional and environmental condition requirements and may not adapt easily to new environmental conditions. In spite of these drawbacks, the cultures sourced from repositories have been fully characterized and their growth and nutritional requirements are known so mass production of these cultures is not technically difficult. However, the pros and cons of microalgal strains sourced from indigenous habitats and culture collection centers depend on the viewpoint of the researcher. Hence it is desirable to bioprospect and source unique indigenous microalgal strains producing the target metabolites. Selection of suitable microalgal strains can be based on their robustness to a wide range of nutrient loading, salinity, pH, light intensity, and temperature. However, diverse microalgal strains have different metabolic and physiological attributes; therefore, location is a key determining factor for the selection of microalgae used to produce biomass (Lee et al., 2014).
Another key question to ask is: are marine microalgal strains superior to and more suitable for the production of bioactive compounds than freshwater strains? Literature indicates that the bulk of characterized and identified microalgal strains producing desirable metabolites with anticancer properties have been isolated from marine habitats (Andrade et al., 2018). Marine microalgae have metabolic plasticity which can trigger the production of several compounds with potential biotechnological applications (Andrade et al., 2018). Also, marine microalgae have additional advantages over their freshwater counterparts as they do not require freshwater, so they cannot compete with food crops for this resource (Salam et al., 2016). However, the utilization of freshwater microalgae is still not economically attractive and cannot support a large scale biodiesel production due to a huge freshwater footprint required (Salam et al., 2016). Therefore, it is desirable to use marine species due to smaller freshwater footprint (Salam et al., 2016). However, freshwater microalgal species can be adapted to grow in industrial, municipal and textile wastewater and can efficiently treat the wastewater while concomitantly propagating biomass for the production of desirable metabolites for various applications such as biodiesel production (Fazal et al., 2018). The integration of bioremediation and biodiesel production processes is reported to potentially improve biodiesel production and wastewater treatment but this process coupling needs to be thoroughly investigated to identify and optimize crucial process factors such as algal species, cultivation and harvesting methods, bioremediation mechanism inter alia (Fazal et al., 2018).
Essentially, there are four biotechnologically important microalgal species, namely: Chlamydomonas, Haematococcus, Chlorella, and Nannochloropsis spp. (Lee et al., 2017). Other microalgal strains of major biotechnological and commercial importance are Spirulina (Arthrospira, Chlorella vulgaris, Dunaliella salina, and Haematococcus pluvialis (Suganya et al., 2016; Mobin and Alam, 2017). Furthermore, among the microalgae, Nannochloropsis salina, Pheaodactylum tricornutum, and Isochrysis galbana are the dominant photosynthetic phytoplankton in the marine environment that are reported to produce high amounts of bioactive compounds (Sirisuk et al., 2018). However, the search and screening of unique marine and freshwater microalgae amenable for biotechnological exploitation are still ongoing. It is, therefore, crucial to select robust microalgal strains for subsequent biotechnological applications. Two key determinants for the selected microalgal strains are high biomass productivity as well as adaptation to regional climatic conditions (Selvarajan et al., 2015).
The objective of scaling-up is to increase the production rate with similar or higher productivity and product quality. Scaling-Up is critical when it comes to developing a viable bioprocess. All operations must start from the laboratory scale to define and establish the crucial techniques as well as the operating conditions. Expanding microalgae culture from the laboratory to commercial scale will always face the challenge related to the adaptation of operating conditions associated with large microalgal operations. The operating conditions influencing microalgal cultivation at large scale include temperature, mixing, microbial contamination, pH, oxygen build-up, photoperiod, light intensity, biofouling, and salinity. Consequently, several large-scale operations produce lower biomass yields than those realized under laboratory conditions (Hsu and Wu, 2002). However, the main advantage of large-scale microalgae operations is the capture of a significant amount of carbon dioxide and prominent potential harmful greenhouse gases. Microalgae can absorb these potentially harmful compounds and use them as components for growth.
The challenges associated with laboratory to large scale-up encompass both technical and economic barriers. Because of the generality of matters related to scaling up, there is a need to investigate further to foster microalgae cultivation research. Nutrient sources and water recycling are technically trivial and affordable for small scale microalgal cultivation and to date, they represent the main technical and economic problems at large scale operations. The acceptable sustainable option is to tap into existing agricultural or municipal wastewater streams to lower nutrient costs. However, there is a risk of introducing harmful substances, pathogens, chemicals or heavy metals into the biomass stream (Hoffman et al., 2008; Wilson et al., 2009). Furthermore, little is known about artificial pond ecology or pathology, more studies into these areas are vital to developing large-scale cultivation risk mitigation and remediation strategies. Four broad cultivation challenges have emerged that are important to address for economically viable, commercial-scale microalgal cultivation:
• Culture stability;
• Standardized metrics for system-level productivity analysis;
• Nutrient source scaling, sustainability and management; and
• Water conservation, management, and recycling.
These four challenges can be overcome by undertaking more experimental and modeling studies to predict the optimum operating conditions and parameters. It is also important to know that each microalgal strain has its unique characteristics, and its behavior at the laboratory level can be affected at a large scale. Furthermore, the economics related to these challenges require more studies and have to be taken into consideration. Each aspect has financial implications when scaling up. Scaling-up influences the stability of the culture because operating conditions will be affected during cultivation due to size expansion. The productivity rate, the amount of nutrients and water usage, recycling will also be influenced because of the size expansion from the laboratory to a large scale. The size expansion has a significant impact on the operating and environmental conditions during cultivation.
Cultivation of microalgae can be done in open ponds or PBRs. In open pond systems, the culture is exposed to the environment. Solar energy is commonly used as the power source for light to allow the process of photosynthesis. The temperature of the culture is regulated by water evaporation from the pond. This system is made of paddle wheels for the mixing and circulation of the microalgal cell suspension (Chen et al., 2011). Open ponds are known to be cost-effective since their construction uses low-cost raw materials and is energy efficient. Two major weaknesses of open ponds are ineffective control of temperature and light intensity. Open ponds require a relatively large area and only a handful of microalgal strains can be cultivated using this system. There is also a possibility and a higher risk of culture contamination and low microalgal biomass density realized using open ponds (Carvalho et al., 2006). PBRs are closed systems that are frequently used for commercial-scale cultivation of microalgae. Using PBRs, microalgal growth conditions are controlled to achieve specific biological modification (Richmond and Cheng-Wu, 2001). PBRs are easy to operate as compared to open ponds. Therefore, effective mixing and mass transfer of gas or liquid can be achieved easily. PBRs are more productive than open ponds. This is due to the most effective use of the cultivation area and efficient energy consumption (Carvalho et al., 2006; Sierra et al., 2008). Previous studies have reported that the cultivation of microalgae in PBRs can yield higher lipid content in comparison to biomass generated from open ponds (Steen et al., 2010). However, PBRs use artificial illumination, therefore, it can be energy-intensive. Furthermore, light conversion performance for PBRs is restricted, because of heat generation due to contact with light sources (Steen et al., 2010). The facility installation expenditure higher and their operating costs are higher since they require more power (Widjaja et al., 2009). More studies are needed to optimize operational costs.
Microalgal biomass harvesting is an important costs component because of energy consumption and capital costs of the equipment used for harvesting. There are many options available for microalgae harvesting, all of them are based on solid-liquid separation. Major harvesting processes used for microalgal biomass harvesting are centrifugation, filtration, sedimentation, and flotation (Danquah et al., 2009; Chen et al., 2011). Table 2 summarizes some advantages and disadvantages of these microalgal biomass harvesting techniques.
TABLE 2. Strengths and limitations of harvesting techniques adapted from (Mohn, 1988; Grima et al., 2003; Shen et al., 2009).
Microalgal milking techniques are harvest methods that target products from cells which remain viable while classical methods involve extraction of products from dead microalgal biomass (Miazek et al., 2017). Depending on the microalgal species, several high-value products can be extracted from both viable and dead microalgal biomass. Various commercial products can be derived from microalgae. These include products for human and animal nutrition, polyunsaturated fatty acids (PUFAs), anti-oxidants, coloring substances, fertilizers, soil conditioners, and a variety of specialty products such as flocculants, biodegradable polymers, cosmetics, pharmaceuticals, polysaccharides, and stable isotopes for research purposes.
The synthesis of these products can be achieved through a biorefinery unit. The primary components of microalgal biomass which are carbohydrates, fats (oils), proteins and a variety of inorganic and complex organic molecules can be converted into different products, either through chemical, enzymatic or microbial conversion. This implies that the extraction or production from microalgae biomass is achieved via metabolic reactions, synthesis or/and separation processes. Metabolite extraction depends on identifying the particular biological component for extraction, which is dependent on the algal species and growth status. Figure 3 represents the biorefinery conceptual model involving main aspects related to the production of microalgal extracts. There is a need for novel technologies with increased efficiencies and reduced environmental impacts to be developed to handle the large amount of waste that is predicted to be generated by the extraction processes. Figure 4 represents an overview of the five potential options for the recovery and use of microalgal co-products. These options are achieved via chemical, biological conversion or processing as indicated earlier.
FIGURE 3. Biorefinery concept model adapted from (U.S. DOE, 2010).
FIGURE 4. Potential options for the recovery and use of co-products adapted from (U.S. DOE, 2010).
The production of microalgal based-fuels is still not yet cost-effective because of the low oil output from many microalgal species. To solve the issue of low oil content, physiological modification can be undertaken after cultivation to stimulate the increase of oil output in microalgal cells. This can be done through nutrients deprivation. However, many microalgal metabolite extracts mentioned earlier can be produced at affordable costs and they have significant potential on the market. Table 3 presents a summary of microalgal products that are low cost and have very large potential markets are developed.
There is a possibility to have an integrated process that can lead to the production of microalgae based biodiesel and jet fuel. Cultivation, harvesting and physiological modification for microalgal biomass are undertaken prior to conversion processes. Transesterification is the main process that lead to the production of biodiesel when using microalgae oil. Furthermore, catalytic unit processes such as decarboxylation, deoxygenation, hydrothermal liquefaction can be used for jet fuel.
Biodiesel and Jet fuel from microalgae can be described as renewable and low carbon fuels, they may have similar performance indicators compared to conventional fuels from fossil origin. It is well known that the most important characteristic of fossil fuels lies on the fact that they have high energy content, this is related to a very low oxygen content close to zero. Crude bio-oil from biomasses including microalgae have generally molecules with relatively high levels of oxygen compared to crude oil from fossil fuels (Saber et al., 2016). Therefore, the removal of oxygen during the conversion processes is more than a necessity while maximizing the final energy content. Therefore, the current trends will use processes such thermal or catalytic cracking, catalytic hydrocracking and hydrotreating, and catalytic structural isomerization. Various producers are exploring the conversion of fatty acids to biodiesel and jet fuel using some processing steps mentioned earlier. These processes have managed to produce biodiesel at the commercial scale but for jet fuel once the challenge related to lipid is overcome the cost effective production will not be a problem. However, with jet fuel there is still more to be done to reach the maturity and commercial scales. The technical feasibility is more than a success but the operating costs are still not effective. However, the best option for jet fuel is the blending with conventional jet fuel that seems to be a more successful option because some commercial jet test flights have been tested (Bwapwa et al., 2019).
Hydrothermal treatment is a chemical reductive process; it can also be used for the conversion of microalgae lipids into green fuels. The process aims mainly to reduce double bonds to yield hydrocarbons essential in a fuel content. The major challenge to use microalgae oils through hydrotreatment is to have a relevant catalyst that can assist in producing an efficient green or renewable fuel. Catalysts in petroleum processes are optimized and have a specific objective and action in order to perform the required reaction in a cost-effective way. Therefore, there is a necessity to adapt the catalysts used in petroleum processes to microalgal oil conversion into green fuels. This is possible because microalgal oil as many similarities in terms of content compared to petroleum crude oil. The main difference between both crude oils is such that microalgal oil has low carbon emissions compared to fossil crude oil. The use of catalysts will allow the attack on the oxygen-bearing carbon atoms to reduce the loss of CO, CO2, and H2 used during the process. Microalgal oil may have some levels of phosphorous from phospholipids, nitrogen from proteins and metals (especially magnesium) from chlorophyll. The optimization of the level of purification for microalgal lipid and the catalyst acceptance for the contaminants are required to achieve the cost-effectiveness of the process.
Transesterification is a reaction between methanol or ethanol and triacylglycerols from microalgae oil in order to produce biodiesel (methyl esters) (Demirbas, 2009). Transesterification can be performed via catalytic or non-catalytic reaction under defined temperature. The technology has reached the adequate maturity and commercial level for the conversion of vegetable oils into biodiesel (Hossain et al., 2008).
Transesterification of microalgal oil can be completed with ethanol using sodium ethanolate as a based-catalyst (Zhou and Boocock, 2006). Ether and salt water should be added to the solution with adequate mixing to allow the separation of the products from the transesterification reaction. Biodiesel is then separated from the ether by a vaporizer under a high vacuum. Transesterification of microalgal oil can also be achieved via acid-catalyzed reactions (Wahlen et al., 2008). The acid catalysts may include H2SO4, HCl or H3PO4, they are known to be less sensitive in the presence of water and free acids, consequently, alleviating saponification and emulsification. As a result, the product recovery is enhanced (Ataya et al., 2008). Generally, acid catalysts have a weakness of lower activity compared to alkaline catalysts. It is reported that there is a requirement for higher temperature and longer reaction times when using acid catalysts (Mumtaz et al., 2017). Microalgal oil produces compliant biodiesel compared to relevant standards involving physico-chemical parameters. For instance, the cetane number of transesterified vegetable/microalgae oil is slightly higher than diesel fuel. Therefore, there is an improvement of ignition quality of biodiesel in the engine. Transesterification of microalgal oil is a key process for biodiesel production, as it can adapt the viscosity of the microalgal oil and improve the heat of combustion to a level closer to the conventional diesel (Math and Chandrashekhara, 2016). Chemical transesterification presents a number of weaknesses such as formation of inorganic precipitates that require their removal, high temperature reactions, possibility of catalysts contamination and probable occurrence of secondary reactions. the current trends are focusing on biochemical transesterification using enzymes catalyzed reactions that present many advantages such as enzyme specificity, reuse ability, genetically improved efficiency, mild reaction conditions, ability to accept new substrates, natural and thermal stability, and capability to catalyze green reactions (Mumtaz et al., 2017). It is possible to produce jet fuel from biodiesel by processing biodiesel through decarboxylation/deoxygenation. During these catalytic processes carbonyl group, oxygen and methyl esters are removed from biodiesel via catalysts such as Ni/C and Pd/C for the conversion to jet fuel. It is an alternative way offering a number of important advantages over hydrotreating. Given that microalgal/biomass led fuels have high oxygen content, methods to deoxygenate biomass-derived oils are currently being pursued to eliminate the excessive amount of oxygen in the fuel. Oxygen is removed in the form of CO2 or CO (Santillan-Jimenez et al., 2015) Decarboxylation/deoxygenation does not require high hydrogen pressures. Catalytic cracking or isomerization is also used to breakdown long chain molecules of hydrocarbons into short alkanes which are relevant for the production of Jet fuel or any other microalgae based fuel. It is a very important process that takes place after carboxylation/deoxygenation. After these processes the crude bio-oil from microalgae should go through the fractionation unit for the separation of relevant carbon fractions related to green gasoline, jet fuel and diesel.
Hydrothermal liquefaction is a process used for the conversion of wet microalgal biomass to a range of liquid fuels (Hu Y. et al., 2019). The technology uses super critical chemistry under 450–500°C and 350 bar to allow the transformation of low energy density microalgae slurry or other biomass into valuable high energy liquid fuels such as green diesel and jet fuel (Hu Y. et al., 2019). Oxygen is removed from the organic molecular structure, increasing the H/C ratio and the energy density of the hydrocarbon equivalent bio crude. During the process there is harnessing of the high activity of water in a subcritical environment which decomposes microalgal biomass into smaller molecules of higher energy density (Hu Y. et al., 2019). Bio-crude oil is the main product generated from the process, it has energy content that is equivalent to diesel and can be upgraded further. The thermal efficiency of bio-crude oil as high as 80%. Once crude bio-oil is produced, fractionation follows thereafter to get various fractions related to gasoline, jet fuel and diesel. Some previous studies using thermal liquefaction of microalgae species such as Dunaliella tertiolecta and Botryococcus braunii have shown promising results in terms of the quality of produced fuels (Minowa et al., 1995; Sawayama et al., 1995). Also some comparative studies have reported that the liquefaction of microalgal biomass was more effective for the production of biodiesel compared to the use of supercritical carbon dioxide (Aresta et al., 2005). Hydrothermal liquefaction approach is a very promising one but the commercial level has not yet reached the maturity, currently it is close to commercial level because the pilot level has been successful, however, more research is required to make it commercially viable option. It is possible to produce biodiesel and jet fuel by integrating some of the processes mentioned earlier in one set-up.
Microalgal biomass is rich in various compounds that have important applications in the biomedical and pharmaceutical industries (Chew et al., 2017; Katiyar et al., 2017). Microalgae produce valuable by-products in the form of high-value products like proteins, pigments, carbohydrates and PUFAs (e.g., eicosapentaenoic acid [EPA], docosahexaenoic acid [DHA], and carotenoids including antioxidant substances for the commercial or pharmaceutical purpose (Kirrolia et al., 2013; Suganya et al., 2016; Hu H. et al., 2019). These products are produced by the biorefinery concept which involves almost 100% utilization of the biomass accumulated i.e. no biomass material is wasted. The lipids, proteins, and carbohydrates are the most important biomolecules that are used for biofuel production. Intracellular biomolecules are produced in varying quantities by different microalgae depending on the growth conditions i.e. nutrient supply, light quality, and intensity, etc. Table 4 indicates the quantities of the lipids, proteins, and carbohydrates produced by marine and freshwater microalgae.
TABLE 4. Biochemical composition of important freshwater and marine microalgal and cyanobacterial strains for biofuel production (% of dry matter) (Mata et al., 2010; Katiyar et al., 2017; Shuba and Kifle, 2018).
Microalgal lipids are fast gaining popularity due to their wide applications such as biofuel precursors and pharmaceutical applications as antimicrobial and anticancer agents (Sharma and Sharma, 2017). PUFAs have been shown to prevent diseases such as cardiovascular disorders, cancer, asthma, arthritis, kidney, and skin disorders, depression and schizophrenia (Sharma and Sharma, 2017). Of all the microalgal strains investigated so far, Chlorella vulgaris is reportedly the best candidate for lipid production (Sarayloo et al., 2017). This is because C. vulgaris is a fast-growing freshwater microalga that can grow in harsh conditions and can accumulate up to 58% of triacyglycerols of the total lipid content in a very short space of time (Yeh and Chang, 2012). The lipids produced consist of palmitic acid C16:0, stearic acid C18:0, palmitoleic acid C16:1 and oleic acid C18:1, which are suitable constituents for biodiesel production (Sarayloo et al., 2017). A wide range of microalgal species can synthesize C14:0, C16:0, C18:1, C18:2 and C18:3 free fatty acids, while other fatty acids including C16:2, C16:3, C16:4, C18:4, C20:5, C22:6 are reported to be strain-specific and culture growth conditions also have a contributory effect on the microalgal lipid profile and composition (Salam et al., 2016).
In order to endure adverse environmental conditions e.g., nutrient limitation, microalgae typically store lipids in the form of triacylglycerides (Ratnapuram et al., 2018). Most lipids are synthesized by microalgae under ambient environmental conditions where light, CO2, and water are available for primary production via photosynthesis. However, it has been demonstrated that under physiologically stressful conditions such as nutrient limitation (specifically nitrogen limitation), substantial amounts of lipids are synthesized at the expense of other macromolecules (Gifuni et al., 2018; Hu H. et al., 2019). Although nutrient limitation is a popular strategy for enhancing lipid yield for the production of biodiesel, it suffers from compromised biomass productivity (Singh et al., 2017). Research indicates that manipulation and maneuvering of culture conditions (e.g., temperature, light intensity, pH, CO2, nitrogen starvation, and phosphate limitation) and subjecting microalgae to stress conditions significantly enhances bioproductivity efficiency (Hu H. et al., 2019).
A significant increase in lipid productivity was reported by subjecting microalgal cultures to diverse stress conditions such as low light intensity, glucose, and bicarbonate supplementation and nitrogen starvation (Adamakis et al., 2018; Ratnapuram et al., 2018). Optimized photoautotrophic, heterotrophic and mixotrophic cultivation modes are appropriate for achieving both higher biomass and lipid productivity for biodiesel applications (Ratnapuram et al., 2018). However, a two-stage cultivation strategy is proposed as an attractive option to resolve the conflict between cell growth and the production of valuable molecules. According to Fu et al. (2017), nitrogen starvation induces the genes that encode enzymes directly involved in lipid metabolism, while protein biosynthesis is reduced to acclimatize cells to the decreased availability of amino acids. The three microalgal cultivation modes are reported to produce substantial yields of macromolecules (Ratnapuram et al., 2018). As previously stated, the most important lipids for biofuel applications are the neutral lipids with suitable carbon chain length. The essential fatty acids specifically long-chain PUFAs (LC-PUFAs) are also important for the treatment of various diseases (cardiovascular, arthritis, cancer, etc.) and for human nutrition applications (Suganya et al., 2016). These fatty acids such as linolenic acid (LA), AA, EPA, and DHA are reported to play a crucial role in the physical, mental and visual developments in infants (Suganya et al., 2016). The LC-PUFAs are mainly obtained from fish but due to toxicity issues and the fishy smell, it is desirable to obtain these important oils from the marine microalgae which are the dominant primary producers. The major microalgae suited for DHA production are Crypthecodinium cohnii, Schizochytrium sp., and Ulkenia sp. (Suganya et al., 2016). However, to date, the major bottleneck for the economical production of microalgal lipids is low lipid productivity.
Marine, hypersaline and freshwater microalgae and cyanobacteria produce valuable carotenoids under suitable growth conditions (Fae Neto et al., 2018). Human beings cannot biosynthesize these compounds, therefore, they have to get them from their diet. Carotenoids are an interesting diverse group of accessory colourful lipophilic pigments with more than 600 members exhibiting different chemical structures. These compounds play key roles in light-harvesting, encompassing photo-protection in both photosynthetic plants and microorganisms (Paliwal et al., 2016). Besides, carotenoids can also be used as a biomarker for the chemotaxonomic identification and profiling of different strains of microalgae and have wide applications in photobiology, photochemistry, and photomedicine (Paliwal et al., 2016). The major microalgal producers of commercial products and carotenoids are dominated by Arthrospira sp., Dunaliella salina, Haematococcus pluvialis, and Aphanizomenon flosaquae inter alia (Suganya et al., 2016). Also, chlorophytes have biosynthetic and biochemical properties similar to land plants and therefore harbor potential for biotechnological production of carotenoids (Varela et al., 2015). The biosynthetic pathway for the biosynthesis of carotenoids is well established (Jaswir et al., 2011).
Carotenoids are divided into two major classes based on their structural elements; carotenes are constituted by carbon and hydrogen (e.g., β-carotene, α-carotene, and lycopene), and xanthophylls are constituted by carbon, hydrogen, and additionally oxygen (e.g., lutein, β-cryptoxanthin, zeaxanthin, astaxanthin, and fucoxanthin) (Jaswir et al., 2011). Of these, the major types of carotenoids are lycopene, β-carotene, and astaxanthin and these compounds are reported to harbor antioxidant activities and are therefore used as health food, supplements, and feeds. Carotenoids such as β-carotene are reported to have anti-tumor and cancer-preventive activity (Suganya et al., 2016). Both neutral lipid accumulation and carotenoid production during cyst formation by H. pluvialis is enhanced under stress conditions such as nutrient limitation and high light intensity (Liang et al., 2015). The yield of carotenoids by the major microalgal producers vary from approximately 0.1–2% of the dry weight of biomass depending on the ambient growth conditions.
PBPs are brightly colored, highly fluorescent, water-soluble, covalently attached linear tetrapyrolic pigments that are structurally related to the bile pigment biliverdin and have a spectrum of applications (Nair et al., 2018). These protein compounds constitute important components of light-harvesting complexes of the photosynthetic machinery in cyanobacteria (blue-green algae), red algae, and cryptomonads (Manirafasha et al., 2016). The PBPs have been purified by conventional techniques of protein fractionation such as sulfate precipitation, ion-exchange chromatography, and gel filtration chromatography to get pure PBPs (Kuddus et al., 2013). According to Chew et al. (2017), the aqueous two-phase extraction (ATPE) is a suitably efficient alternative to the commonly used tedious and time-consuming chromatographic techniques. The structure and function of PBPs have been elucidated (De Marsac, 2003).
PBPs have been isolated as distinct subunits as either trimers (αβ)3, of approximately Mr 110–120 kDa (e.g., allophycocyanins) or hexamers (αβ)6γ of about Mr 250 kDa (certain phycoerythrins) (Glazer, 1994). PBPs are conveniently classified according to their spectral properties and these spectroscopic properties of PBPs are determined by the presence of different chromophores known as phycobilins (Nair et al., 2018). Phycoerythrins are most abundant in red algae and some unicellular cyanobacteria. Spectra of these proteins show strong peaks between 480 and 570 nm with strong fluorescence at 580 nm. Allophycocyanin absorbs maximally at 650 nm with an emission maximum at 660 nm. Besides spectral properties, PBPs are classified into two large groups based on their brilliant colors, the phycoerythrin (red), and the phycocyanin (blue). Due to their distinct physicochemical properties, PBS are also classified into phycoerythrocyanin, R-phycoerythrin, B-phycoerythrin, phycocyanin, and allophycocyanin which have important applications as fluorescent tags in flow cytometry, fluorescence-activated cell sorting, histochemistry, immunoassay and detection of reactive oxygen species (Nair et al., 2018). Besides, PBPs have major applications in natural dyes in the food industry and pharmaceutical products. PBPs exhibits antioxidant, antiviral, anticancer, anti-allergic, anti-inflammatory, and neuroprotective properties making them promising biomaterials in health-related applications (Chew et al., 2017). The major cyanobacterial strains producing PBPs are Spirulina sp., Synechococcus sp., Nostoc sp. etc. Both outdoor and closed PBRs are used for the production of PBPs. Four methods are documented for the production of PBPs: photoautotrophic production, heterotrophic production, mixotrophic production, and recombinant production. According to Hemlata et al. (2011), PBPs can be obtained from microalgal cells through various physical techniques such as centrifugation, drying, homogenization, repeated freeze-thaw process consecutively.
Besides biofuel applications, marine microalgae are currently being considered a potentially new, versatile and valuable source of bioactive compounds, due to their metabolic plasticity, for modern biotechnological applications (Andrade et al., 2018). Microalgal biomolecules have versatile modern applications such as biomedical, pharmaceutical, cosmetics, thalassotherapy, agro-industrial, prebiotic, neutraceutical applications, inter alia (Falaise et al., 2016). Microalgal and cyanobacterial bioactive molecules are currently showing promising results for the abatement and treatment of prevalent diseases such as cancer, HIV-AIDS, malaria, diabetes, obesity, etc. To date, successful bioactivity screening of diatoms, dinoflagellates, flagellates and cyanobacteria, and clinical trials have been contacted and results published (Lauritano et al., 2016). The key to the successful application of the bioactive compounds is the extraction and purification of the active ingredient from the microalgal biomass. Numerous clinical trials are currently being conducted worldwide to potentially address some of the pertinent human health conditions using microalgal products.
According to literature, research and development (i.e., basic research, drug discovery, pre-clinical trials, three-phase clinical trials, Food and Drug Administration [FDA] review and finally large scale manufacturing) of newly approved drugs by the FDA may take 10–15 years and costing millions of dollars and less than 12% of the drugs receiving final approval (Andrade et al., 2018). Hence, it is desirable and highly recommended to consider ocean acquired medicines specifically phytoplanktonic bioactive molecules (Andrade et al., 2018). The preponderance of active compounds such as polyunsaturated aldehydes (PUAs), chrysolaminaran polysaccharide, violaxanthin, EPA, DHA, fucoxanthin, stigmasterol, nonyl 8-acetoxy-6-methyl octanoate (NAMO) and monogalactosyl glycerols, in some microalgal strains entail their biomedical and pharmaceutical functionality (Abd El-Hack et al., 2019).
To date, attempts have been made to harness the different expression systems for the recombinant production of pharmaceutical proteins (Hempel and Maier, 2012). Nonetheless, many of these expression systems have attendant disadvantages e.g., low product yield, high costs, complex and multi-stage purification, and potential product contamination by human pathogens (Hempel and Maier, 2012). Hempel and Maier (2012), successfully demonstrated for the first time that completely assembled and functional human IgG antibodies can both be efficiently expressed to high levels in algal systems and effectively secreted into the extracellular culture medium. According to these co-workers, the diatom Phaeodactylum tricornutum was successfully engineered to synthesize and secrete human IgG antibodies against the Hepatitis B Virus surface protein. Under natural conditions, the diatom Phaeodactylum tricornutum does not secrete any intracellular proteins. The major advantage is that the secreted antibodies are already in pure form, therefore extensive and costly purification steps are rendered redundant. Hence, microalgae hold great promise as work horses due to easy scale-up of bioprocessing, their rapid growth rates associated with all the merits of eukaryotic expression systems, and therefore offer great potential for natural sunlight-powered, low cost production of pharmaceutical proteins. The current bottlenecks bedevilling the commercial production of microalgal compounds can be overcome by modern molecular biology approaches as well as development of efficient product recovery processes.
Cancer is the unrestricted proliferation of cells in the body. Globally, cancer is the major cause of human death, hence, finding an effective therapeutic agent for treating this scourge is an urgent necessity (Andrade et al., 2018). Microalgae harboring anticancer activities such as Dunaliella tertiolecta, Chlorella ellipsoidea, Phaeodactylum tricornutum, Skeletonema spp. etc. have been reported and the search for ideal microalgal candidates producing these bioactive molecules is still ongoing (Andrade et al., 2018). Recently, it has been reported that microalgal culture conditions such as light intensity, temperature, and nutrient availability affect the anti-cancer bioactivity of microalgae (Andrade et al., 2018).
Due to the high morbidity and mortality caused by diabetes complications, it is crucial to explore other alternative sources of bioactive compounds to effectively treat this debilitating, chronic and degenerative metabolic condition (Lauritano and Ianora, 2016). Hence, the detection and screening of eukaryotic microalgae and prokaryotic cyanobacteria for enzymes with potential clinical applications has been going on for some years and has been intensely investigated (Cannell et al., 1987). The selection of suitable superior microalgae and cyanobacteria involves the detection of possible inhibitors of specific enzymes using analytical colorimetric assays. Screening for antidiabetes activity mainly involves the determination of the catalytic activity of specific enzymes involved in sugar metabolism, in both experimental animal trials and human patients (Lauritano and Ianora, 2016). Some of these key enzymes are α-amylase, α-glucosidase, N-acetyl-glucosaminidase, aldose reductase, hexokinase, glucose-6-phosphatase, dipeptidyl peptidase IV, glucose transporter 4, and glycogen synthase kinase-3β. However, α-amylase and α-glucosidase are the two key enzymes involved in glucose metabolism, therefore, they are the common targets for anti-diabetic assays. Both α-amylase and α-glucosidase are involved in the breakdown of ingested carbohydrates and the inhibition of these two key enzymes delays the absorption of glucose hence this is a feasible route for the management of type-2 diabetes (Lauritano and Ianora, 2016). Several microalgae are reported to have anti-diabetes activity by producing bioactive compounds such as carotenoids, PUFAs, astaxanthin, etc. Several microalgae with anti-diabetes activity are sourced from both freshwater and marine biotopes and these include Chlorella spp. Nitzschia laevis, Isochrysis galbana, Chaetoceros furcellatus, Skeletonema marinoi, Porosira glacidis, inter alia (Lauritano and Ianora, 2016). To date, the search and bioprospecting for microalgae producing antidiabetes bioactive compounds are rapidly expanding and the application of microalgal extracts for diabetes treatment is gaining global recognition.
The HIV/AIDS pandemic has decimated large communities especially in developing countries where HIV/AIDS awareness campaigns were slowly accepted as intervention strategies. To date, the mortality rates are gradually decreasing due to some vigorous interventions due to the development of effective antiHIV therapy. Despite these interventions, the emergence of HIV drug resistance and side effects are of major concern and therefore it is mandatory to search and isolate alternative antiHIV compounds from unique natural sources (Vo and Kim, 2010). Marine microalgal extracts have been demonstrated to habour antiHIV activity. Sulfated polysaccharides (SPs) consists of a complex group of polymers displaying numerous bioactivities (Kremb et al., 2014). Besides certain invertebrates and some mammals, freshwater and marine algae, as well as some strains of cyanobacteria, produce SPs.
To date, SPs isolated from marine algae from extreme habitats have attracted attention from the global research community. SPs have a wide range of bioactivities such as antiHIV, anticancer, anti-inflammatory, among other applications and the potency increases with the degree of sulfation (Schaeffer and Krylov, 2000). These polymers have an inhibitory effect on the attachment of viruses with target molecules on the cell surface (Vo and Kim, 2010). Research has demonstrated that the diatom Navicula directa from marine deep seawater habitats is a source of SPs called naviculum, a complex compound made up of several sugar molecules. Naviculum was demonstrated to inhibit the formation of cell-cell fusion between HIV gp160 and CD4-expressing HeLa cells with an IC50 value of 53 µg/ml (Vo and Kim, 2010). This indicates that algae can play a crucial role in the abatement of HIV-1 infection. Research data suggests that extracts isolated from Undaria and Spirulina and a combination of both are nontoxic and their long term consumption may improve clinical endpoints of HIV/AIDS (Teas and Irhimeh, 2012). Hence the production of several algal secondary metabolites, specifically SPs from algae with anti-viral activity are currently generating a lot of interest from the international research community (Olasehinde et al., 2017).
Malaria is one of the leading causes of mortality in tropical countries where this disease is endemic. Malaria is caused by the female Anopheles mosquito and Plasmodium falciparum is the common malaria parasite responsible for most malaria mortalities worldwide. The recommended treatment of malaria is the administration of a combination of chloroquine and proguanil, doxycycline, mefloquine and atovaquone combined with proguanil. For the past few decades, resistance has emerged to all classes of antimalarial drugs except the artemisinins and is responsible for a recent increase in malaria-related mortality, particularly in Africa. The emergence of resistance can potentially be controlled by the use of secondary metabolites derived from microalgae, macroalgae, and cyanobacteria.
Crude extracts from algae belonging to Chlorophyta, Heterokontophyta and Rhodophyta strains have been extensively investigated for their antiplasmodial activities against P. falciparum (erythrocytic stages), T. cruzi (trypomastigotes) and L. donovani (axenic amastigotes) (Patra et al., 2015). Secondary metabolites with anti-malarial properties produced by Sargassum heterophyllum include sargaquinoic acid, sargahydroquinoic acid, sargaquinal, and fucoxanthin. The secondary metabolites fucoxanthin and sargaquinal demonstrated good antiplasmodial activity against a chloroquine-sensitive strain of Plasmodium falciparum (Sanmukh et al., 2014). Extracts from the macroalga Sargassum swartzii and Chondria dasyphylla were investigated for larvicidal activities in larvae of the malaria vector Anopheles stephensi and the mortality rate of Anopheles stephensi was 96 and 95%, respectively (Sanmukh et al., 2014).
Extracts from microalgal biomass habour antimicrobial properties and have been documented to inhibit and kill potentially food spoilage and human pathogenic microorganisms such as bacteria, fungi and viruses (Rizwan et al., 2018; Schuelter et al., 2019). The effectiveness of the microalgal extract is highly dependent on the type of microalga, the solvent used for extraction and the concentration of the extract (Pina-Pérez et al., 2017). Extracts from both macroalgae and microalgae have been documented to have antimicrobial potential against Staphylococcus aureus, Escherichia coli, Salmonella spp., Bacillus cereus, Listeria monocytogenes, Aspergillus niger, Candida albicans, etc. (Patel et al., 2019). However, the antiviral potential of microalgal extracts against foodborne viruses is currently being investigated. There is a serious lack of information on the antimicrobial properties of microalgal extracts against foodborne viruses (Pina-Pérez et al., 2017). Besides, algae have other important applications such as cosmetics and thalassotherapy, ingredients for agricultural herbicides, prebiotic and nutraceutical applications (Lauritano et al., 2016; Patel et al., 2019).
Numerous meaningful contributions have been made to optimize the benefits that algae offer mankind. However, it has been suggested that the exploitation of their full potential may only be achieved after genetically manipulating a particular species for a specific purpose. Successful modification of microalgae requires a permanent expression of inserted genes after nuclear or chloroplast transformation. These procedures were frequently, albeit with limited success, carried out through electroporation and particle bombardment or by Agrobacterium tumefaciens-mediated transformation (Abd El-Hack et al., 2019). Unlike with bacterial, plant and mammalian cells, genetic engineering of a vast majority of microalgal strains is met with several challenges owing to the poor frequency or complete lack of homologous recombination in the nuclear genome (Tanwar et al., 2018). As such, a range of genome editing tools has been investigated to improve homologous recombination. There are various critical factors that hinder hamper successful microalgal transformation, such as the paucity of available suitable genetic tool kits, selection of potential metabolic candidates, promoters for the expression vectors, etc., therefore severely hindering progress in microalgal biotechnology (Daboussi et al., 2014).
The zinc-finger nucleases (ZFNs) were developed to cut a genome at specific sites allowing for homologous recombination between a genomic sequence of interest and the template DNA (Sizova et al., 2013). ZFNs were designed and used to modify the genome of C. reinhardtii by targeting the COP3 gene encoding a light-activated ion channel (Sizova et al., 2013). The ZFN technology was particularly successful in Chlamydomonas and is still useful in investigating the genomic function of the genus. Similarly, the transcription activator-like effector nucleases (TALEN), restriction enzymes with the same function as ZFNs were used in P. tricornutum to target several genes including, but not limited to the UDP-GP, G3P, and EAR genes as well the urease enzyme (Daboussi et al., 2014; Weyman et al., 2015). Although these methods were successful in producing transgenic microalgal strains, the procedures involved are often expensive, tedious and time-consuming.
Reliability on unsustainable resources for fuel production, variability in fuel prices and the adverse effects associated with CO2 emissions have prompted the search for new, renewable and stable biofuels to meet the world’s energy demands. The potential of algae as an important resource in this regard, cannot be overstated. Radakovits et al. (2010) provided a comprehensive overview of the progress made in developing transgenic algal strains, highlighting progress in optimizing biofuel production. Biofuel production from algae is dependent on the quality and quantity of diesel precursors which are associated closely with lipid and fatty acid metabolism. As a result, research has focused on increasing the expression of enzymes responsible for fatty acid synthesis (Radakovits et al., 2010). Microalgal species including Chlamydomonas reinhardtii, Dunaliella salina, and Phaeodactylum tricornutum were genetically engineered to improve the concentrations of fatty acids (Rathod et al., 2017). The wild type cyanobacterium Synechocystis sp. PCC6803 was genetically manipulated by introducing an acyl-acyl carrier protein thioesterase gene which allowed for the production and secretion of fatty acids in the growth medium (Liu et al., 2014). Cyanobacteria were also genetically modified enabling them to produce 1.7 µmol ethanol per mg of chlorophyll per hour by expressing a construct containing the genomic information required for the expression of pyruvate decarboxylase and alcohol dehydrogenase enzymes from Zymomonas mobilis (Woods et al., 2004).
Algae have displayed promise as sources of novel chemical components or scaffolds with applications in the pharmaceutical and medical fraternities. However, since these compounds occur at trace amounts and are very rare in nature, genetic modifications are necessary to improve the yield. Genetic manipulation of microalgae and cyanobacteria also offers new opportunities for the production of biopharmaceuticals including antibodies, immunotoxins, antigens, hormones clotting factors and bioactive peptides. Tran et al. (2012) revealed that the chloroplast of C. reinhardtii is capable of accumulating eukaryotic toxins making them ideal hosts for the production of immunotoxins. Chloroplasts of C. reinhardtii were transformed by particle bombardment with a plasmid containing the RFB4 antibody, the αCD22 sugar-binding transmembrane protein as well as exotoxin A (PE40) from Pseudomonas aeruginosa. The immunotoxin produced, referred to as αCD22PE40, was capable of prolonging the lifespan of mice implanted with human B-cell tumors (Tran et al., 2012). Similary, Wannathong et al. (2016) utilized chloroplast engineering to establish a transgenic C. reinhardtii line with the ability to express a synthetic gene encoding human growth hormone. Transformation of C. reinhardtii chloroplasts was achieved by agitating a suspension containing algae and the plasmid DNA containing a synthetic gene encoding human growth hormone and a gene encoding erythromycin resistance from Escherichia coli. The methods proposed by Wannathong et al. (2016) describe a routine protocol that ensures the expression of a targeted gene of interest into the chloroplast of C. reinhardtii.
Genetic engineering of algal chloroplasts thus represents a valuable tool to generate numerous products including biofuels and novel therapeutics. However, since the majority of work on genetic engineering of chloroplasts has been conducted on C. reinhardtii as a model species, there still exist limitations in exploiting the true potential of algae. The discovery of the clustered regular interspaced short palindromic repeats (CRISPRs) and CRISPR associated proteins (Cas) offers an efficient approach to genomic editing. CRISPR and the Cas9 protein were discovered as part of the adaptive immune system of bacteria responsible for identifying and cleaving foreign nucleic acids and has been used to successfully modify the genome of several microalgal strains including C. reinhardtii, Volvox carteri, P. tricornutum, and Chlorella variabilis. Recently, Yao et al. (2016) showed that a nuclease-deficient Cas9/CRISPR interference represses targeted genes in cyanobacterium species Synechcocystis sp. PCC 6803. In this regard, the technology was successfully used to repress the green fluorescent protein as well as to repress the formation of polyhydroxy butyrate and glycogen during nitrogen starvation. Nymark et al. (2016) developed an efficient method to generate stable gene mutations in the P. tricornutum using the Cas9 nuclease and an associated guide RNA which targets a sequence of interest. The ability of CRISPR to efficiently edit and knock-out genes holds much promise for the production of biofuels and pharmaceuticals from microalgae.
Besides the production of value-added products, microalgae offer opportunities for the treatment of municipal and industrial wastewater and previous research has documented microalgae as good candidates for this role (Nur and Buma, 2019). The high rate algal ponds are effective at treating wastewaters such as municipal sewage and textile wastewater (Fazal et al., 2018). Microalgae can offer opportunities such as nutrient removal e.g., macromolecules such as nitrates and phosphates that can trigger multiple and multiscale damages to the receiving environment due to eutrophication in water bodies (Fazal et al., 2018). The treatment of wastewaters using microalgae consortia offers advantages such as 1) cooperative interactions between the co-cultivated microorganisms can occur, enhancing the overall uptake of nutrients; and 2) these systems tend to be more resistant to environmental conditions oscillations (Salama et al., 2018). Besides, microalgae propagated in wastewater exhibit the advantages of having high photosynthetic efficiency, high biomass productivity, and it benefits from having a rapid growth rate (Li et al., 2018).
Upstream and downstream challenges are major bottlenecks for the success of the microalgal biotechnology industry (Roux et al., 2017). Microbial contamination is problematic especially in open raceway ponds and this can seriously lower biomass yields. Poor biomass settleability and the occurrence of microalgal cells in aqueous suspension poses serious biomass harvesting challenges (Quijano et al., 2017). The development of suitable and cost-effective harvesting strategies is still ongoing. Besides, the microalgal biomass is processed rapidly since the changing conditions during harvesting of the microalgae can trigger cellular lipase induction and other metabolic changes leading to the rapid hydrolysis of the lipids to FFAs, which then pose negative effects on the final desired products by the conventional method (Sawangkeawa and Ngamprasertsith, 2013). Disruption of microalgal cells to extract the metabolites is also a serious challenge that requires a lasting solution. According to Zheng et al. (2016), enzymatic cell disruption is a promising, highly energy-efficient technology for recovery of cellular compounds from microalgal cells, but it has not been applied at large scale because of its low cost-efficiency. This is exacerbated by the structural diversity and rigidity of microalgal cell walls, complicating the development of efficient downstream processing methods for cell-disruption and subsequent recovery of intracellular metabolites (Lee et al., 2017). The main challenge for the successful microalgal biorefinery industry is the availability of robust strains producing high amounts of the desired products. The route to circumvent this is by genetically engineering superior microalgal strains for the overexpression of the desired gene products. This is feasible but so far very little success has been reported on the genetic modification of wild type microalgal strains. Although the microalgal biotechnology industry is currently booming, there are still great challenges to achieve economic microalgal biorefinery (Chen et al., 2017; Cho et al., 2017). Although microalgae harbor diverse biopolymer components, some challenges still linger such as 1) species selection must be suitable for the target metabolite 2) high photosynthetic efficiency for high product yield is still a limitation, 3) maintenance of monoculture during cultivation is problematic especially under outdoor cultivation, 4) high energy intensity and utilization during cultivation and downstream processing, 5) few commercial plants in operation, therefore lack of data for large scale plants, 6) toxicity of flue gas use as a source of CO2 due to the presence of poisonous compounds such as NOx and SOx (Brennan and Owende, 2010).
The biotechnology industry is rapidly expanding and prospects promise to be rewarding at the pace at which research developments are taking place especially concerning growth bioreactor designs, biomass harvesting systems and genetic modification of superior microalgal strains. Low biomass and lipid productivity remain major barriers to the full economic viability of the algae-based biodiesel (Kwak et al., 2016). Currently, high-energy and cost-intensive downstream processes such as cell disruption and metabolite extraction remain the key techno-economic bottlenecks to full commercialization of microalgal biodiesel production (Lee et al., 2018). It is therefore paramount to find and select microalgal strains with a fast growth rate and high metabolite productivity (Kwak et al., 2016). The cost of producing metabolites from microalgal cells is reported to be expensive therefore it is desirable to develop cost-effective bioprocess systems for the whole production system to be profitable. The unit cost for the production of algae-based biofuel must be reduced for commercialization compared to petroleum (Kwak et al., 2016). The future direction of the biotechnology industry is to significantly improve the product yields and to manipulate the genes of the suitable microalgal strains to overexpress the gene products of interest. New vistas in molecular biology offer new approaches for the generation of new microalgal strains with desirable strains. Furthermore, the utilization of basic substrates such as wastewater and sewage for biomass accumulation is also an area that must be fully developed to make the biotechnology industry cost-effective. Optimization of growth parameters for the maximal yield of biomass and metabolites is crucial so that these conditions can be applied at large scale operations for the process to be productive and economic. Though process optimization using stress manipulation strategy has been the main focus, there is a need to combine microalgal strain development and process integration for efficient bioproduction (Chen et al., 2017). According to Kim et al. (2018), conventional approaches utilized in many of the biorefinery processes i.e., strain selection and development, biomass analysis, lipid extraction, and analysis; all mostly rely on bulky instruments and complicated procedures that are time-consuming, labor-intensive, high cost, and ultimately low throughput.
This manuscript critically evaluated the current status of the microalgal biotechnology industry focusing on the biorefinery approach for the production of microalgal value-added products for various biotechnological applications. Microalgae are efficient primary producers that can yield a wide array of complex compounds such as carotenoids, PUFAs, PBPs etc. for biomedical and pharmaceutical applications. Microalgae are currently gaining a lot of attention as suitable biofactories due to the ease of production as well as their short generation times. However, it is crucial to carefully select robust and best suitable microalgal candidates for the production of these compounds.
The high costs associated with microalgal bioprocessing for biofuel production are major constraints for the success of the algal biotechnology industry, hence the biorefinery approach in combination with bioenergy production is proposed as a panacea to enhanced competitiveness of this topical industry. The microalgal biotechnology is still in infancy and to date, the search for robust and superior microalgal strains is still ongoing. However, it is critical to decide whether to source the strains from culture collection centers or the local aquatic habitats. The diversity of microalgal strains is huge so it is crucial to fully explore and exploit microalgal strains from the local habitats such as freshwater, marine, and hyper-saline. The microalgal biorefinery approach is gaining importance due to the preponderance of products such as lipids, pigments, carbohydrates, proteins and PBPs, nutraceuticals, vitamins, etc. These valuable compounds are gaining prominence due to their medical and pharmaceutical applications. Although there are still some hurdles and challenges, the microalgal biotechnology industry is a multimillion-dollar industry and the future of this industry is fast becoming popular. Finally, the development of superior microalgal strains through gene editing and genetic modification of the desirable traits is currently ongoing and breakthroughs are still forthcoming especially the successful over production of microalgal recombinant proteins/pharmaceuticals for potential biotechnological applications.
All authors listed have made a substantial, direct, and intellectual contribution to the work and approved it for publication.
The authors declare that the research was conducted in the absence of any commercial or financial relationships that could be construed as a potential conflict of interest.
The authors would like to gratefully acknowledge the National Research Foundation (South Africa) UID 116066 for funding and Mangosuthu University of Technology for the research facilities and financial support.
Abd El- Hack, M. E., Abdelnour, S., Alagawany, M., Abdo, M., Sakr, M. A., Khafage, A. F., et al. . (2019). Microalgae in modern cancer therapy: current knowledge. Biomed. Pharmacother. 111, 42–50. doi:10.1016/j.biopha.2018.12.069
Adamakis, I. D., Lazaridis, P. A., Terzopoulou, E., Torofias, S., Valari, M., Kalaitzi, P., et al. . (2018). Cultivation, characterization, and properties of Chlorella vulgaris microalgae with different lipid contents and effect on fast pyrolysis oil composition. Environ. Sci. Pollut. Res. 25 (23), 23018–23032. doi:10.1007/s11356-018-2368-5
Andrade, K. A. M., Lauritano, C., Romano, G., and Ianora, A. (2018). Marine microalgae with anti-cancer properties. Mar. Dugs. 16 (165), 1–17. doi:10.3390/md16050165
Aresta, M., Dibenedetto, A., and Barberio, G. (2005). Utilization of macro-algae for enhanced CO2 fixation and biofuels production: development of a computing software for an LCA study. Fuel Process Technol. 86 (14-15), 1679–1693. doi:10.1016/j.fuproc.2005.01.016
Ataya, F., Dube, M. A., and Ternan, M. (2008). Variables affecting the induction period during acid-catalyzed transesterification of canola oil to FAME. Energy Fuels 22 (1), 679–685. doi:10.1021/ef7005386
Banu, J. R., Kavitha, P. S., Guvanesekaran, M., and Kumar, G. (2020). Microalgae based biorefinery promoting circular bioeconomy-techno economic and life-cycle analysis. Bioresour. Technol. 302, 122822. doi:10.1016/j.biortech.2020.122822
Benemann, J. R. (1990). Microalgae products and production: an overview. Dev. Ind. Microbiol. 31, 247–256.
Bhatia, S. K., Ravi, S. M., Bhatia, K., Kumar, M., Pugazhendhi, A., Awasthi, M. K., et al. . (2021). Wastewater based microalgal biorefinery for bioenergy production: progress and challenges. Sci. Total Environ. 751, 14159. doi:10.1016/j.scitotenv.2020.141599
Borowitzka, M. A. (1986). Micro-algae as sources of fine chemicals. Microbiol. Sci. 3 (12), 372–375.
Brennan, L., and Owende, P. (2010). Biofuels from microalgae-A review of technologies for production, processing, and extractions of biofuels and co-products. Renew. Sust. Energ. Rev. 14, 557–577. doi:10.1016/j.rser.2009.10.009
Burja, A. M., Banaigs, B., Abou-Mansour, E., Burgess, J. G., and Wright, P. C. (2001). Marine cyanobacteria—A prolific source of natural products. Tetrahedron 57 (46), 9347–9377. doi:10.1016/S0040-4020(01)00931-0
Bwapwa, J. K., Akash, A., and Trois, C. (2019). Jet fuel blend from algal jet fuel and jet A1 in 50/50 volume ratio. Int. J. Low-Carbon Tec. 14 (2), 234–240. doi:10.1093/ijlct/ctz014
Cannell, R. J., Kellam, S. J., Owsianka, A. M., and Walker, J. M. (1987). Microalgae and cyanobacteria as a source of glycosidase inhibitors. J. Gen. Microbiol. 133, 1701–1705. doi:10.1099/00221287-133-7-1701
Carvalho, A. P., Meireles, L. A., and Malcata, F. X. (2006). Microalgal reactors: a review of enclosed system designs and performances. Biotechnol. Prog. 22 (6), 1490–1506. doi:10.1002/bp060065r
Chen, B., Wan, O., Mehmood, M. A., Chang, J. S., Bai, F., and Zhao, X. (2017). Manipulating environmental stresses and stress tolerance of microalgae for enhanced production of lipids and value-added products- A review. Bioresour. Technol. 244, 1198–1206. doi:10.1016/j.biortech.2017.05.170
Chen, C. Y., Yeh, K. L., Aisyah, R., Lee, D. J., and Chang, J. S. (2011). Cultivation, photobioreactor design and harvesting of microalgae for biodiesel production: a critical review. Bioresour. Technol. 102 (1), 71–81. doi:10.1016/j.biortech.2010.06.159
Chew, K. W., Yap, J. Y., Show, P. L., Suan, N. H., Juan, J. C., Ling, T. C., et al. . (2017). Microalgae biorefinery: high-value products perspectives. Bioresour. Technol. 229, 53–62. doi:10.1016/j.biortech.2017.01.006
Chisti, Y. (2016). “Large-scale production of algal biomass: raceway ponds,” in Algae biotechnology, products and processes. Editors F. Bux, and Y. Chisti (Cham, Switzerland: Springer International Publishing), 21–40.
Chisti, Y. (2007). Biodiesel from microalgae. Biotechnol. Adv. 25, 294–306. doi:10.1016/j.biotechadv.2007.02.001
Cho, H. U., Kim, Y. M., and Park, J. M. (2017). Enhanced microalgal biomass and lipid production from a consortium of indigenous microalgae and bacteria present in municipal wastewater under gradually mixotrophic culture conditions. Bioresour. Technol. 228, 290–297. doi:10.1016/j.biortech.2016.12.094
Cobos, M., Paredes, J. D., Maddox, J. D., Vargas-Arana, G., Flores, L., Aguilar, C. P., et al. . (2017). Isolation and characterization of native microalgae from the Peruvian Amazon with potential for biodiesel production. Energies 10, 1–16. doi:10.3390/en10020224
Daboussi, F., Ledus, S., Marechal, A., Dubois, G., Guyot, V., Perez-Michaut, C., et al. . (2014). Genome engineering empowers the diatom Phaeodactylum tricornutum for biotechnology. Nature Commun. 5, 3831. doi:10.1038/ncomms4831
Danquah, M. K., Ang, L., Uduman, N., Moheimani, N., and Forde, G. M. (2009). Dewatering of microalgal culture for biodiesel production: exploring polymer flocculation and tangential flow filtration. J. Chem. Technol. Biotechnol. 84 (7), 1078–1083. doi:10.1002/jctb.2137
De Marsac, N. T. (2003). Phycobiliproteins and phycobilisomes: the early observations. Photosynth. Res. 76 (1-3), 197–205. doi:10.1023/A:1024954911473
Delrue, F., Álvarez-Díaz, P. D., Fon-Sing, S., Fleury, G., and Sassi, J-F. (2016). The environmental biorefinery: using microalgae to remediate wastewater, a win-win paradigm. Energies 9 (132), 1–19. doi:10.3390/en9030132
Demirbas, A. (2009). Production of biodiesel from algae oils. Energ. Source Part A. 31 (2), 163–168. doi:10.1080/15567030802093955
Duong, V. T., Ahmed, F., Thomas-Hall, S. R., Quigley, S., Nowak, E., and Schenk, P. M. (2015). High protein- and high lipid-producing microalgae from northern Australia as potential feedstock for animal feed and biodiesel. Front. Bioeng. Biotechnol. 3 (53), 1–7. doi:10.3389/fbioe.2015.00053
Fae Neto, W. A., Borges Mendes, C. R., and Abreu, P. C. (2018). Carotenoid production by the marine microalgae Nannochloropsis oculata in different low-cost culture media. Aquac. Res. 49, 1–9. doi:10.1111/are.13715
Falaise, C., François, C., Travers, M-A., Morga, B., Haure, J., Tremblay, R., et al. . (2016). Antimicrobial compounds from eukaryotic microalgae against human pathogens and diseases in aquaculture. Mar. Drugs. 14, 159. doi:10.3390/md14090159
Fazal, T., Mushtaq, A., Rehman, F., Khan, A. U., Rashid, N., Farooq, W., et al. . (2018). Bioremediation of textile wastewater and successive biodiesel production using microalgae. Renew. Sust. Energ. Rev. 83, 3107–3126. doi:10.1016/j.rser.2017.10.029
Figueroa-Torres, G. M., Pittman, J. K., and Theodoropoulos, C. (2017). Kinetic modelling of starch and lipid formation during mixotrophic, nutrient-limited microalgal growth. Bioresour. Technol. 241, 868–878. doi:10.1016/j.biortech.2017.05.177
Fu, L., Cui, X., Li, Y., Xu, L., Zhang, C., Xiong, R., et al. . (2017). Excessive phosphorus enhances Chlorella regularis lipid production under nitrogen starvation stress during glucose heterotrophic cultivation. Chem. Eng. J. 330, 566–572.
Gifuni, I., Olivieri, G., Pollio, A., and Marzocchella, A. (2018). Identification of an industrial microalgal strain for starch production in biorefinery context: the effect of nitrogen and carbon concentration on starch accumulation. New Biotechnol. 41, 46–54. doi:10.1016/j.nbt.2017.12.003
Glazer, A. N. (1994). Phycobiliproteins - a family of valuable, widely used fluorophores. J. Appl. Phycol. 6, 105–112. doi:10.1007/bf02186064
Grima, E. M., Belarbi, E. H., Fernández, F. A., Medina, A. R., and Chisti, Y. (2003). Recovery of microalgal biomass and metabolites: process options and economics. Biotechnol. Adv. 20 (7-8), 491–515. doi:10.1016/s0734-9750(02)00050-2
Gumbi, S. T., Majeke, B. M., Olaniran, A. O., and Mutanda, T. (2017). Isolation, identification and high-throughput screening of neutral lipid producing indigenous microalgae from South African aquatic habitats. Appl. Biochem. Biotechnol. 182, 382–399. doi:10.1007/s12010-016-2333-z
Hemlata, P. G., Bano, F., and Fatma, T. (2011). Studies on Anabaena sp. NCCU-9 with special reference to phycocyanin. J. Algal Biomass Utln. 2 (1), 30–51
Hempel, F., and Maier, U. G. (2012). An engineered diatom acting like a plasma cell secreting human IgG antibodies with high efficiency. Microb. Cell Fact. 11, 126. doi:10.1186/1475-2859-11-126
Hildebrand, M., Abbriano, R. M., Polle, J. E. W., Traller, J. C., Trentacoste, E. M., Smith, S. R., et al. . (2013). Metabolic and cellular organization in evolutionarily diverse microalgae as related to biofuels production. Curr. Opin. Chem. Biol. 17, 506–514. doi:10.1016/j.cbpa.2013.02.027
Hoffman, Y., Aflalo, C., Zarka, A., Gutman, J., James, T. Y., and Boussiba, S. (2008). Isolation and characterization of a novel chytrid species (phylum Blastocladiomycota), parasitic on the green alga Haematococcus. Mycol. Res. 112, 70–81. doi:10.1016/j.mycres.2007.09.002
Hossain, A. B. M. S., Salleh, A., Boyce, A. N., Chowdhury, P., and Naqiuddin, M. (2008). Biodiesel fuel production from algae as renewable energy. Am. J. Biochem. Biotechnol. 4 (3), 250–254. doi:10.3844/ajbbsp.2008.250.254
Hsu, Y-L., and Wu, W. T. (2002). A novel approach for scaling-up a fermentation system. Biochem. Eng. J. 11 (2-3), 123–130. doi:10.1016/s1369-703x(02)00016-5
Hu, H., Li, J. Y., Pana, X. R., Zhang, F., Ma, L. L., Wang, H. J., et al. . (2019). Different DHA or E PA production responses to nutrient stress in the marine microalga Tisochrysis lutea and the freshwater microalga Monodus subterraneus. Sci. Total Environ. 656, 140–149. doi:10.1016/j.scitotenv.2018.11.346
Hu, Y., Gong, M., Feng, S., Xu, C. C., and Bassi, A. (2019). A review of recent developments of pre-treatment technologies and hydrothermal liquefaction of microalgae for bio-crude oil production. Renew. Sust. Energ. Rev. 101, 476–492. doi:10.1016/j.rser.2018.11.037
Jaswir, I., Noviendri, D., Hasrini, R. F., and Octavianti, F. (2011). Carotenoids: sources, medicinal properties and their application in food and nutraceutical industry. J. Med. Plants Res. 5 (33), 7119–7131. doi:10.5897/JMPRX11.011
Katiyar, R., Gurjar, B. R., Biswas, S., Pruthi, V., Kumar, N., and Kumar, P. (2017). Microalgae: an emerging source of energy-based bio-products and a solution for environmental issues. Renew. Sust. Energ. Rev. 72, 1083–1093. doi:10.1016/j.rser.2016.10.028
Khan, M. I., Shin, J. H., and Kim, J. D. (2018). The promising future of microalgae: current status, challenges, and optimization of a sustainable and renewable industry for biofuels, feed, and other products. Microb Cell Fact 17, 36. doi:10.1186/s12934-018-0879-x
Kim, H. S., Devarenne, T. P., and Han, A. (2018). Microfluidic systems for microalgal biotechnology: a review. Algal Res. 30, 149–161. doi:10.1016/j.algal.2017.11.020
Kirrolia, A., Bishnoi, N. R., and Singh, R. (2013). Microalgae as a boon for sustainable energy production and its future research and development aspects. Renew. Sust. Energ. Rev. 20, 642–656. doi:10.1016/j.rser.2012.12.003
Kremb, S., Helfer, M., Kraus, B., Wolff, H., Wild, C., Schneider, M., et al. . (2014). Aqueous extracts of the marine brown alga Lobophora variegata inhibit HIV-1 infection at the level of virus entry into cells. Plos ONE. 9 (8), e103895. doi:10.1371/journal.pone.0103895
Kuddus, M., Singh, P., Thomas, G., and Al-Hazimi, A. (2013). Recent developments in production and biotechnological applications of C-phycocyanin. BioMed. Res. Int. 2013, 1–9. doi:10.1155/2013/742859
Kwak, H. S., Kim, J. Y. H., Woo, H. M., Jin, E. S., Min, B. K., and Sim, S. J. (2016). Synergistic effect of multiple stress for improving microalgal lipid production. Algal Res. 9, 215–224. doi:10.1016/j.algal.2016.09.003
Lauritano, C., Andersen, J. H., Hansen, E., Albrigtsen, M., Escalera, L., Esposito, F., et al. . (2016). Bioactivity screening of microalgae for antioxidant, anti-inflammatory, anticancer, anti-diabetes, and antibacterial activities. Front. Mar. Sci. 3 (68), 1–12. doi:10.3389/fmars.2016.00068
Lauritano, C., and Ianora, A. (2016). Marine organisms with anti-diabetes properties. Mar. Drugs 14, 220. doi:10.3390/md14120220
Lee, K., Eisterhol, M. L., Rindi, F., Palanisami, S., and Nam, P. K. (2014). Isolation and screening of microalgae from natural habitats in the Midwestern United States of America for biomass and biodiesel sources. J. Nat. Sci. Bio. Med. 5 (2), 333–339. doi:10.4103/0976-9668.136178
Lee, N., Ko, S-R., Ahn, C-Y., and Oh, H-M. (2018). Optimized co-production of lipids and carotenoids from Ettlia sp. by regulating stress conditions. Bioresour. Technol. 258, 234–239. doi:10.1016/j.biortech.2018.03.006
Lee, S. Y., Cho, J. M., Chang, Y. K., and Oh, Y-K. (2017). Cell disruption and lipid extraction for microalgal biorefineries: a review. Bioresour. Technol. 244, 1317–1328. doi:10.1016/j.biortech.2017.06.038
Leliaert, F., Smith, D. R., Moreau, H., Herron, M. D., Verbruggen, H., Delwiche, C. F., et al. . (2012). Phylogeny and molecular evolution of the green algae. Crit. Rev. Plant Sci. 31, 1–46. doi:10.1080/07352689.2011.615705
Li, H., Wang, M., Wang, X., Zhang, Y., Lu, H., Duan, N., et al. . (2018). Biogas liquid digestate grown Chlorella sp. for biocrude oil production via hydrothermal liquefaction. Sci. Total Environ. 635, 70–77. doi:10.1016/j.scitotenv.2018.03.354
Liang, C., Zhai, Y., Xu, D., Ye, N., Zhang, X., Wang, Y., et al. . (2015). Correlation between lipid and carotenoid synthesis and photosynthetic capacity in Haematococcus pluvialis grown under high light and nitrogen deprivation stress. Grasas Aceites 66 (2), e077. doi:10.3989/gya.0708142
Liu, J., Sun, Z., Gerken, H., Huang, J., Jiang, Y., and Chen, F. (2014). Genetic engineering of the green alga Chlorella zofingiensis: a modified norflurazon-resistant phytoene desaturase gene as a dominant selectable marker. Appl. Microbiol. Biotechnol. 98, 5069–5079. doi:10.1007/s00253-014-5593-y
Malapascua, J. R. F., Jerez, C. G., Sergejevová, M., Figueroa, F. L., and Masojídek, J. (2014). Photosynthesis monitoring to optimize growth of microalgal mass cultures: application of chlorophyll fluorescence techniques. Aquat. Biol. 22, 123–140. doi:10.3354/ab00597
Manirafasha, E., Ndikubwimana, T., Zeng, X., Lu, Y., and Jing, K. (2016). Phycobiliprotein: potential microalgae derived pharmaceutical and biological reagent. Biochem. Eng. J. 109, 282–296. doi:10.1016/j.bej.2016.01.025
Mata, T. M., Martins, A. A., and Caetano, N. S. (2010). Microalgae for biodiesel production and other applications: a review. Renew. Sust. Energ. Rev. 14, 217–232. doi:10.1016/j.rser.2009.07.020
Math, M. C., and Chandrashekhara, K. N. (2016). Optimization of alkali catalyzed transesterification of safflower oil for production of biodiesel. J. Eng. 6, 1–7. doi:10.1155/2016/8928673
Metting, B., and Pyne, J. W. (1986). Biologically active compounds from microalgae. Enzyme Microb. Technol. 8 (7), 386–394. doi:10.1016/0141-0229(86)90144-4
Miazek, K., Kratky, L., Sulc, R., Jirout, T., Aguedo, M., Richel, A., et al. . (2017). Effect of organic solvents on microalgae growth, metabolism and industrial bioproduct extraction: a review. Int. J. Mol. Sci. 18, 1429. doi:10.3390/ijms18071429
Minowa, T., Yokoyama, S.-Y., Kishimoto, M., and Okakura, T. (1995). Oil production from algal cells of Dunaliella tertiolecta by direct thermochemical liquefaction. Fuel 74, 1735–1738. doi:10.1016/0016-2361(95)80001-x
Mobin, S., and Alam, F. (2017). Some promising microalgal species for commercial applications: a review. Energy Procedia 110, 510–517. doi:10.1016/j.egypro.2017.03.177
Mohn, F. (1988). “Harvesting of micro-algal biomass,” in Microalgae biotechnology. Editors J. Borowitz, and M. A. Borowitzka (Cambridge, UK: Cambridge University Press).
Moreno-Garcia, G., Adjallé, K., Barnabé, S., and Raghavan, G. S. V. (2017). Microalgae biomass production for a biorefinery system: recent advances and the way towards sustainability. Renew. Sust. Energ. Rev. 76, 493–506. doi:10.1016/j.rser.2017.03.024
Mumtaz, M. W., Adnan, A., Mukhtar, H., Rashid, U., and Danish, M. (2017). “Biodiesel production through chemical and biochemical transesterification: trends, technicalities, and future perspectives,” in Clean energy for sustainable development. K. A. Azad, and S. Sharma (Cambridge, MA: Academic Press), 465–485.
Mutanda, T., Ramesh, D., Karthikeyan, S., Kumari, S., Anandraj, A., and Bux, F. (2011). Bioprospecting for hyper-lipid producing microalgal strains for sustainable biofuel production. Bioresour. Technol. 102, 57–70. doi:10.1016/j.biortech.2010.06.077
Nair, D., Krishna, J. G., Pannikar, M. V. N., Nair, B. G., Pai, J. G., and Nair, S. S. (2018). Identification, purification, biochemical and mass spectrometric characterization of novel phycobiliproteins from a marine red alga, Centroceras clavulatum. Int. J. Biol. Macromol. 114, 679–691. doi:10.1016/j.ijbiomac.2018.03.153
Nur, M. M. A., and Buma, A. G. J. (2019). Opportunities and challenges of microalgal cultivation on wastewater, with special focus on palm oil mill effluent and the production of high value compounds. Waste Biomass Valori. 10, 2079–2097. doi:10.1007/s12649-018-0256-3
Nymark, M., Sharma, A. K., Sparstad, T., Bones, A. M., and Winge, P. (2016). A CRISPR/Cas9 system adapted for gene editing in marine algae. Sci. Rep. 6, 24951–24957. doi:10.1038/srep24951
Olaizola, M. (2003). Commercial development of microalgal biotechnology: from the test tube to the marketplace. Biomol. Eng. 20, 459–466. doi:10.1016/s1389-0344(03)00076-5
Olasehinde, T. A., Olaniran, A. O., and Okoh, A. L. (2017). Therapeutic potentials of microalgae in the treatment of Alzheimer’s disease. Molecules. 22, 480. doi:10.3390/molecules22030480
Paliwal, C., Ghosh, T., George, B., Pancha, I., Maurya, R., Chokshi, K., et al. . (2016). Microalgal carotenoids: potential nutraceutical compounds with chemotaxonomic importance. Algal Res. 15, 24–31. doi:10.1016/j.algal.2016.01.017
Patel, A., Matsakas, L., Rova, U., and Christakopoulos, P. (2019). A perspective on biotechnological applications of thermophilic microalgae and cyanobacteria. Bioresour. Technol. 278, 424–434. doi:10.1016/j.biortech.2019.01.063
Patra, K. P., Li, F., Carter, D., Gregory, J. A., Baga, S., Reed, S. G., et al. . (2015). Alga-produced malaria transmission-blocking vaccine candidate Pfs25 formulated with a human use-compatible potent adjuvant induces high-affinity antibodies that block Plasmodium falciparum infection of mosquitoes. Infect. Immun. 83, 1799–1808. doi:10.1128/iai.02980-14
Pereira, H., Schulze, P. S. C., Schüler, M. S., Santos, T., Barreira, L., and Varela, J. (2018). Fluorescence activated cell-sorting principles and applications in microalgal biotechnology. Algal Res. 30, 113–120. doi:10.1016/j.algal.2017.12.013
Philip, S., Keshavarz, T., and Roy, I. (2007). Polyhydroxyalkanoates: biodegradable polymers with a range of applications. J. Chem. Technol. Biotechnol. 82 (3), 233–247. doi:10.1002/jctb.1667
Pina-Pérez, M. C., Rivas, A., Martínez, A., and Rodrigo, D. (2017). Antimicrobial potential of macro and microalgae against pathogenic and spoilage microorganisms in food. Food Chem. 235, 34–44. doi:10.1016/j.foodchem.2017.05.033
Pulz, O., and Gross, W. (2004). Valuable products from biotechnology of microalgae. Appl. Microbiol. Biotechnol. 65, 635–648. doi:10.1007/s00253-004-1647-x
Quijano, G., Arcila, J. S., and Buitrón, G. (2017). Microalgal-bacterial aggregates: applications and perspectives for wastewater treatment. Biotechnol. Adv. 35, 772–781. doi:10.1016/j.biotechadv.2017.07.003
Radakovits, R., Jinkerson, R. E., Darzins, A., and Posewitz, M. C. (2010). Genetic engineering of algae for enhanced biofuel production. Eukaryot. Cell. 9, 486–501. doi:10.1128/ec.00364-09
Radmer, R. J. (1996). Algal diversity and commercial algal products. BioScience 46 (4), 263–270. doi:10.2307/1312833
Radmer, R. J., and Parker, B. C. (1994). Commercial applications of algae: opportunities and constraints. J. Appl. Phycol. 6 (2), 93–98. doi:10.1007/BF02186062
Rathod, J. P., Gade, R. M., Rathod, D. R., and Dudhare, M. (2017). A review on molecular tools of microalgal genetic transformation and their application for overexpression of different genes. Int. J. Curr. Microbiol. App. Sci. 6, 3191–3207. doi:10.20546/ijcmas.2017.612.373
Ratnapuram, H. P., Vutukuru, S. S., and Yadavalli, R. (2018). Mixotrophic transition induced lipid productivity in Chlorella pyrenoidosa under stress conditions for biodiesel production. Heliyon 4, 1–20. doi:10.1016/j.heliyon.2017.e00496
Rawat, I., Kumar, R. R., Mutanda, T., and Bux, F. (2013). Biodiesel from microalgae: a critical evaluation from laboratory to large scale production. Appl. Energy. 103, 444–467. doi:10.1016/j.apenergy.2012.10.004
Rawat, I., Kumar, R. R., Mutanda, T., and Bux, F. (2011). Dual role of microalgae: phycoremediation of domestic wastewater and biomass production for sustainable biofuels production. Appl. Energy. 88 (10), 3411–3424. doi:10.1016/j.apenergy.2010.11.025
Richmond, A., and Cheng-Wu, Z. (2001). Optimization of a flat plate glass reactor for mass production of Nannochloropsis sp. outdoors. J. Biotechnol. 85 (3), 259–269. doi:10.1016/s0168-1656(00)00353-9
Rizwan, M., Mujtaba, G., Memon, S. A., Leed, K., and Rashid, N. (2018). Exploring the potential of microalgae for new biotechnology applications and beyond: a review. Renew. Sust. Energ. Rev. 92, 394–404. doi:10.1016/j.rser.2018.04.034
Roux, J-M., Lamotte, H., and Achar, J-L. (2017). An overview of microalgae lipid extraction in a biorefinery framework. Energy Procedia 112, 680–688. doi:10.1016/j.egypro.2017.03.1137
Saber, M., Nakhshiniev, B., and Yoshikawa, K. (2016). A review of production and upgrading of algal bio-oil. Renew. Sust. Energ. Rev. 58, 918–930. doi:10.1016/j.rser.2015.12.342
Salam, K. A., Velasquez-Orta, S. B., and Harvey, A. P. (2016). A sustainable integrated in situ transesterification of microalgae for biodiesel production and associated co-product-a review. Renew. Sust. Energ. Rev. 65, 1179–1198. doi:10.1016/j.rser.2016.07.068
Salama, E-S., Hwang, J-S., El-Dalatony, M. M., Kurade, M. B., Kabra, A. N., Abou-Shanab, R. A. J., et al. . (2018). Enhancement of microalgal growth and biocomponent-based transformations for improved biofuel recovery: a review. Bioresour. Technol. 258, 365–375. doi:10.1016/j.biortech.2018.02.006
Sanmukh, S., Bruno1, B., Ramakrishnan, U., Khairnar, K., Swaminathan, S., and Paunikar, W. (2014). Bioactive compounds derived from microalgae showing antimicrobial activities. J. Aquac. Res. Dev. 5 (3) 1–4. doi:10.4172/2155-9546.1000224
Santillan-Jimenez, E., Morgan, T., Loe, R., and Crocker, M. (2015). Continuous catalytic deoxygenation of model and algal lipids to fuel-like hydrocarbons over Ni–Al layered double hydroxide. Catal. Today. 258, 284–293. doi:10.1016/j.cattod.2014.12.004
Sarayloo, E., Tardu, M., Unlu, Y. S., Simsek, S., Cevahir, G., Erkey, C., et al. . (2017). Understanding lipid metabolism in high-lipid-producing Chlorella vulgaris mutants at the genome-wide level. Algal Res. 28, 244–252. doi:10.1016/j.algal.2017.11.009
Sawangkeawa, R., and Ngamprasertsith, S. (2013). A review of lipid-based biomasses as feedstocks for biofuels production. Renew. Sust. Energ. Rev. 25, 97–108. doi:10.1016/j.rser.2013.04.007
Sawayama, S., Inoue, S., Dote, Y., and Yokoyama, S. Y. (1995). CO2 fixation and oil production through microalga. Energ. Convers. Manage. 36, 729–731. doi:10.1016/0196-8904(95)00108-p
Schaeffer, D. J., and Krylov, V. S. (2000). Anti-HIV activity of extracts and compounds from algae and cyanobacteria. Ecotoxicol. Environ. Saf. 45, 208–227. doi:10.1006/eesa.1999.1862
Schuelter, A. R., Kroumov, A. D., Hinterholz, C. L., Fiorini, A., Trigueros, D. E. G., Vendruscolo, E. G., et al. . (2019). Isolation and identification of new microalgae strains with antibacterial activity on food-borne pathogens. Engineering approach to optimize synthesis of desired metabolites. Biochem. Eng. J. 144, 28–39. doi:10.1016/j.bej.2019.01.007
Selvarajan, R., Felföldi, T., Tauber, T., Sanniyasi, E., Sibanda, T., and Tekere, M. (2015). Screening and evaluation of some green algal strains (Chlorophyceae) isolated from freshwater and soda lakes for biofuel production. Energies 8, 7502–7521. doi:10.3390/en8077502
Sharma, N., and Sharma, P. (2017). Industrial and biotechnological applications of algae: a review. J. Adv. Plant Biol. 1 (1), 1–25. doi:10.14302/issn.2638-4469.japb-17-1534
Shen, Y., Yuan, W., Pei, Z. J., Wu, Q., and Mao, E. (2009). Microalgae mass production methods. Trans. ASABE. 52 (4), 1275–1287. doi:10.13031/2013.27771
Shuba, E. S., and Kifle, D. (2018). Microalgae to biofuels: ‘Promising’ alternative and renewable energy, review. Renew. Sust. Energ. Rev. 81, 743–755. doi:10.1016/j.rser.2017.08.042
Sierra, E., Acién, F. G., Fernández, J. M., García, J. L., González, C., and Molina, E. (2008). Characterization of a flat plate photobioreactor for the production of microalgae. Chem. Eng. J. 138 (1-3), 136–147. doi:10.1016/j.cej.2007.06.004
Singh, P., Kumari, S., Guldhe, A., Singh, G., and Bux, F. (2017). ACCase and rbcL gene expression as a function of nutrient and metal stress for enhancing lipid productivity in Chlorella sorokiniana. Energy Convers. Manage. 148, 809–819. doi:10.1016/j.enconman.2017.06.054
Sirisuk, P., Ra, C. H., Jeong, G-T., and Kim, S. K. (2018). Effects of wavelength mixing ratio and photoperiod on microalgal biomass and lipid production in a two-phase culture system using LED illumination. Bioresour. Technol. 253, 175–181. doi:10.1016/j.biortech.2018.01.020
Sizova, I., Greiner, A., Awasthi, M., Kateriya, S., and Hagemann, P. (2013). Nuclear gene targeting in Chlamydomonas using engineered zinc-finger nucleases. Plant J. 73, 873–882. doi:10.1111/tpj.12066
Spolaore, S., Joannis-Cassan, C., Duran, E., and Isambert, A. (2006). Commercial applications of microalgae. J. Biosci. Bioeng. 101, 8796. doi:10.1263/jbb.101.87
Steen, E. J., Kang, Y., Bokinsky, G., Hu, Z., Schirmer, A., McClure, A., et al. . (2010). Microbial production of fatty-acid-derived fuels and chemicals from plant biomass. Nature 463, 559–562. doi:10.1038/nature08721
Suganya, T., Varman, M., Masjuki, H. H., and Renganathan, S. (2016). Macroalgae and microalgae as a potential source for commercial applications along with biofuels production: a biorefinery approach. Renew. Sust. Energ. Rev. 55, 909–941. doi:10.1016/j.rser.2015.11.026
Tanwar, A., Sharma, S., and Kumar, S. (2018). Targeted genome editing in algae using CRISPR/Cas9. Ind. J. Plant Physiol. 23, 653–669. doi:10.1007/s40502-018-0423-3
Teas, J., and Irhimeh, M. R. (2012). Dietary algae and HIV/AIDS: proof of concept clinical data. J. Appl. Phycol. 24, 575–582. doi:10.1007/s10811-011-9766-0
Thao, T. H., Linh, D. T. N., Si, V. C., Carter, T. W., and Hill, R. T. (2017). Isolation and selection of microalgal strains from natural water sources in Viet Nam with potential for edible oil production. Mar. Drugs. 15 (194), 1–14. doi:10.3390/md15070194
Thiruvenkadam, S., Izhar, S., Hiroyuki, Y., and Harun, R. (2018). Subcritical water extraction of Chlorella pyrenoidosa: optimization through response surface methodology. BioMed Res. Int. 2018, 1–10. doi:10.1155/2018/1931634
Tran, M., Van, C., Barrera, D. J., Pettersson, P. L., Peinado, C. D., Bui, J., et al. . (2012). Production of unique immunotoxin cancer therapeutics in algal chloroplasts. PNAS 110, E15–E22. doi:10.1073/pnas.1214638110
U.S. DOE. (2010). National algal biofuels technology roadmap. Washington, D.C.: U.S. Department of Energy, Office of Energy Efficiency and Renewable Energy, Biomass Program USA
Varela, J. C., Pereira, H., Vila, M., and Leon, R. (2015). Production of carotenoids by microalgae: achievements and challenge. Photosynth Res. 25 (3), 423–436. doi:10.1007/s11120-015-0149-2
Vo, T-S., and Kim, S-K. (2010). Potential anti-HIV agents from marine resources: an overview. Mar. Drugs. 8, 2871–2892. doi:10.3390/md8122871
Vu, C. H. T., Lee, H. G., and Chang, Y. K. (2018). Axenic cultures for microalgal biotechnology: establishment, assessment, maintenance, and applications. Biotechnol. Adv. 36 (2), 380–396. doi:10.1016/j.biotechadv.2017.12.018
Wahlen, B. D., Barney, B. M., and Seefeldt, L. C. (2008). Synthesis of biodiesel from mixed feedstocks and longer chain alcohols using an acid-catalyzed method. Energ Fuel 22 (6), 4223–4228. doi:10.1021/ef800279t
Wang, W., Xu, Y., Wang, X., Zhang, B., Tian, W., and Zhang, J. (2018). Hydrothermal liquefaction of microalgae over transition metal supported TiO2 catalyst. Bioresour. Technol. 250, 474–480. doi:10.1016/j.biortech.2017.11.051
Wannathong, T., Waterhouse, J. C., Young, R. E. B., Economou, C. K., and Purton, S. (2016). New tools for chloroplast genetic engineering allowing the synthesis of human growth hormone in the green alga Chlamydomonas reinhardtii. Appl. Microbiol. Biotechnol. 100, 5467–5477. doi:10.1007/s00253-016-7354-6
Weyman, P. D., Beeri, K., Lefebvre, S. C., Rivera, J., McCarthy, J. K., Heuberger, A. L., et al. . (2015). Inactivation of Phaeodactylum tricornutum urease gene using transcription activator-like effector nuclease-based targeted mutagenesis. Plant Biotech. 13, 460–470. doi:10.1111/pbi.12254
Widjaja, A., Chien, C. C., and Ju, Y. H. (2009). Study of increasing lipid production from freshwater microalgae Chlorella vulgaris. J. Taiwan Inst. Chem. Eng. 40 (1), 13–20. doi:10.1016/j.jtice.2008.07.007
Wilkie, A. C., Edmundson, S. J., and Duncan, J. G. (2011). Indigenous algae for local bioresource production: Phycoprospecting. Energy Sustain. Dev. 15, 365–371. doi:10.1016/j.esd.2011.07.010
Wilson, W. H., Van Etten, J. L., and Allen, M. J. (2009). “The Phycodnaviridae: the story of how tiny giants rule the world,” in Lesser known large dsDNA viruses. Editors J. L. van Etten (Berlin, Heidelberg: Springer), 1–42.
Woods, R. P., Coleman, J. R., and De Deng, M. (2004). Genetically modified cyanobacteria for the production of ethanol, the constructs and method thereof. United States Patent. Patent No US6699696B2.
Wu, G. F., Wu, Q. Y., and Shen, Z. Y. (2001). Accumulation of poly-beta-hydroxybutyrate in cyanobacterium Synechocystis sp. PCC6803. Bioresour. Technol. 76 (2), 85–90. doi:10.1016/s0960-8524(00)00099-7
Yao, L., Cengic, I., Anfelt, J., and Hudson, E. P. (2016). Multiple gene repression in cyanobacteria using CRISPi. ACS Synth. Biol. 5 (3), 207–212. doi:10.1021/acssynbio.5b00264
Yeh, K.-L., and Chang, J.-S. (2012). Effects of cultivation conditions and media composition on cell growth and lipid productivity of indigenous microalga Chlorella vulgaris ESP-31. Bioresour. Technol. 105, 120–127. doi:10.1016/j.biortech.2011.11.103
Zhang, X., Rong, J., Chen, H., He, C., and Wang, Q. (2014). Current status and outlook in the application of microalgae in biodiesel production and environmental protection. Front. Energy Res. 2 (32), 1–15. doi:10.3389/fenrg.2014.00032
Zheng, Y., Xiao, R., and Roberts, M. (2016). Polymer-enhanced enzymatic microalgal cell disruption for lipid and sugar recovery. Algal Res. 14, 100–108. doi:10.1016/j.algal.2016.01.010
Zhou, W., and Boocock, D. G. B. (2006). Phase distributions of alcohol, glycerol, and catalyst in the transesterification of soybean oil. J. Am. Oil Chem. Soc. 83 (12), 1047–1052. doi:10.1007/s11746-006-5161-4
Zhou, W., Chen, P., Min, M., Ma, X., Wang, J., Griffith, R., et al. . (2014). Environment-enhancing algal biofuel production using wastewaters. Renew. Sust. Energ. Rev. 36, 256–269. doi:10.1016/j.rser.2014.04.073
Keywords: biorefinery, biotechnology, carotenoids, gene editing, microalgal biomass, omega-3 fatty acids, phycobiliprotein, photobioreactor
Citation: Mutanda T, Naidoo D, Bwapwa JK and Anandraj A (2020) Biotechnological Applications of Microalgal Oleaginous Compounds: Current Trends on Microalgal Bioprocessing of Products. Front. Energy Res. 8:598803. doi: 10.3389/fenrg.2020.598803
Received: 25 August 2020; Accepted: 12 October 2020;
Published: 17 December 2020.
Edited by:
Subramaniapillai Niju, PSG College of Technology, IndiaReviewed by:
Balasubramanian Paramasivan, National Institute of Technology Rourkela, IndiaCopyright © 2020 Mutanda, Naidoo, Bwapwa and Anandraj. This is an open-access article distributed under the terms of the Creative Commons Attribution License (CC BY). The use, distribution or reproduction in other forums is permitted, provided the original author(s) and the copyright owner(s) are credited and that the original publication in this journal is cited, in accordance with accepted academic practice. No use, distribution or reproduction is permitted which does not comply with these terms.
*Correspondence: T. Mutanda, bXV0YW5kYS50YXVyYWlAbXV0LmFjLnph
Disclaimer: All claims expressed in this article are solely those of the authors and do not necessarily represent those of their affiliated organizations, or those of the publisher, the editors and the reviewers. Any product that may be evaluated in this article or claim that may be made by its manufacturer is not guaranteed or endorsed by the publisher.
Research integrity at Frontiers
Learn more about the work of our research integrity team to safeguard the quality of each article we publish.