- 1College of Materials Science and Engineering, Zhejiang University of Technology, Hangzhou, China
- 2Institute of Optoelectronic Materials and Devices, China Jiliang University, Hangzhou, China
- 3SolaXPower Network Technology (Zhejiang) Co., Ltd., Hangzhou, China
- 4School of Pharmaceutical and Chemical Engineering, Taizhou University, Taizhou, China
- 5Zhejiang Meidu Hitrans Lithium Battery Technology Co., Ltd., Shaoxing, China
- 6Hengdian Group DMEGC Magnetics Co. Ltd., Dongyang, China
- 7Materials Science and Engineering Program and Texas Materials Institute, The University of Texas at Austin, Austin, TX, United States
The ever-growing demand for portable devices and electric vehicles are drawing widespread attention to advanced energy storage systems. Over the past few decades, lithium-sulfur batteries (LSBs) have vast potential to act as the next-generation of rechargeable power source due to their high theoretical specific energy, cost-effectiveness, and environmental benignity. However, insufficient sulfur utilization, inferior cyclability, and rate capability originating from the intrinsic insulating features of the sulfur and notorious polysulfide shuttle are major obstacles to fulfilling the industrialization of LSBs. In this respect, the introduction of a functional barrier layer coating on a separator has been verified as an effective strategy to overcome the aforementioned intractable problems. In this review, we focus on summarizing the current progress of the modified polyolefin-based separators (known as functional separators), including functional separator facing cathodes and functional separator facing anodes. According to the working mechanism, functional separator facing cathodes are divided into physical adsorption separators, chemical adsorption separators, catalytic conversion separators, and multifunctional separators. Meanwhile, functional separator facing anodes are classified into physical barrier separators, induced lithium growth separators, regulated lithium nucleation separators, and hybrid mechanism separators. Finally, the future perspective coupled with the practical utilization of functional separators in LSBs is proposed.
Introduction
Benefiting from the efficiency and durability of the energy storage system, portable electronic and smart electronic fields have been devoted to developing minimization for meeting the fast-increasing requirements of human to novel electrified products. Nevertheless, the present-day commercialized lithium-ion batteries (LIBs) are difficult to satisfy the large-scale application of smart grids and electric vehicles due to its high cost and low energy density (<300 Wh kg−1) (Fang et al., 2018; Lin et al., 2020). Therefore, under the premise of the scalable utilization of a high energy density battery system, lithium-sulfur batteries (LSBs) with the advantages in large energy density (>500 Wh kg−1), high natural abundance, inexpensive and nontoxicity are one of the most fascinating battery candidates (Liu et al., 2017b). When it comes to the energy density required for the practical application of LSBs, it can be described via the following formula (Huang et al., 2020):
where E (Wh kg−1) is the gravimetric energy density of LSBs; C (Ah) is specific to the actual discharge capacity of the sulfur cathode; P (V) represents the average potential of the sulfur cathode; W (kg) is the weight of the entire LSB. Obviously, the energy density of LSBs is mainly determined by the sulfur cathode. In other words, the sulfur content, loading, and utilization should be as high as possible (e.g., sulfur content >90 wt. %, sulfur loading > 7 mg cm−2) (Huang et al., 2020). Meanwhile, the total mass of LSBs are made up of a sulfur cathode, Li anode, separator, current collector, and the package weight should be as low as possible.
Despite of the desirable merits, taking LSBs to the commercialized market is still beset with intractable problems. For a sulfur cathode, the insulating nature of sulfur and lithium sulfides (Li2S/Li2S2), the volume expansion of a sulfur cathode, and a serious polysulfide (Li2Sn, 4 ≤ n ≤ 8) shuttle will lead to low reaction kinetics, weak structural integrity as well as the large loss of active materials (Xia et al., 2018). For a lithium anode, polysulfide deposits on the anode surface and subsequently reacts with lithium, which inevitably causes the dendrite, deterioration, and consumption of lithium, increasing the interfacial resistance. The results eventually trigger poor rate capability, inferior capacity, potential safety hazards, and low energy density for LSBs. Generally, in order to settle the above bottlenecks for fulfilling high energy density LSBs, incorporating sulfur into conductive materials could not only improve the conductivity of cathode but also suppress the polysulfide shuttle, thereby delivering the superior electrochemical properties (Fang et al., 2016). Unfortunately, the fabrication methods of those cathodes usually involve complex procedures, toxic solvents, poor reproducibility, and high energy consumption, which strictly limit the practicality of LSBs. Besides, sulfur-based composites are often associated with low sulfur content and loading, which significantly decrease the energy density of the battery (Zhu et al., 2016). Consequently, there is still an urgent need to search for an inexpensive and industrialized strategy to realize advanced LSBs with high sulfur content and loading.
Recently, apart from the design of “inside” sulfur cathodes, novel cell configurations are concentrated on “outside” cathode strategies including modifying the polyolefin separator (known as the functional separator) or placing a functional interlayer between the cathode and separator. On the one hand, these functional separators and interlayers serve as polysulfide reservoirs to alleviate the shuttle effect. On the other hand, these functional separators and interlayers with high conductive materials also act as upper-current collectors to provide additional electron channels for reutilizing the intercepted active materials, thereby leading to the significantly enhanced electrochemical performance of LSBs (Fan et al., 2018; Chen et al., 2020). In this respect, the functionalization of the separator has been prioritized for eliminating the polysulfide shuttle under high sulfur content and loading in recent years. The reasons are elaborated as follows. Firstly, even though the interlayer is beneficial to cell performance, the extra weight from the interlayer may reduce the cell energy density (Chang et al., 2016). Beyond that, the nonpolar feature of routine separators (e.g., polypropylene: PP, polyethylene: PE) can hardly hinder the dissolution and migration of polar polysulfides (Kong et al., 2017b). Last but not least, directly coating the barrier layer onto the surface of the commercial separator can inherit the perfect features of each material, such as strong polysulfide-trapping, excellent flexibility, and exceptional mechanical strength. Most importantly, the functional separators are completed by either sample filtration or the slurry process. (Huang et al., 2015a). The latter has a mass production ability with powerful regulation to the parameter of the coating layer (e.g., size, thickness, weight), which could easily achieve a satisfactory separator for LSBs. Thus, considering these factors, the development of functionally modified separators is highly desirable for the commercialization of LSBs.
So far, many reviews have referred to the functional separators. For example, Xiang et al. (Xiang et al., 2016) discussed the characteristics, advantages, and limitations of functional separators in LSBs. However, most of previous reviews mainly focus on the scientific issues rather than the fabrication protocols of functional separators. These are a big barrier for the further exploration and large-scale application of novel functional separators. Given all that, in this review, we outline the current progress in functional separators and propose rational design and fabrication protocols to achieve a high-quality separator for LSBs. Specifically, two aspects in terms of functional separators are discussed systematically. 1) The classifications of functional separators are summarized by virtue of its working mechanism, and the corresponding research is included comprehensively 2) The rational designs of functional separators (e.g., thickness, weight, porosity, and scalable production) are analyzed, and the resolution strategies are also introduced (Figure 1). Meanwhile, the influence of the design protocols and fabrication methods of functional separators on the electrochemical performance of LSBs are analyzed. This review paves the way for obtaining satisfactory functional separators for the industrial application of LSBs with high sulfur utilization and high energy density in the near future.
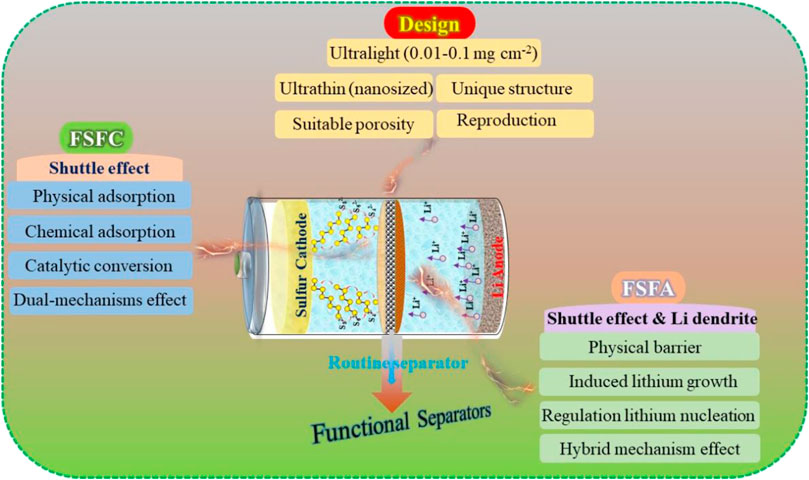
FIGURE 1. Schematic diagram of functional separators and their design based on the various working mechanisms for LSBs.
Functional Separators
For high energy density LSBs, active materials with high content and loading as well as sufficient sulfur utilization are needed. To date, a sulfur/carbon (S/C) cathode is a typical representative in the research of LSBs, but it has a long road before we can realize its commercialization due to the severe polysulfide shuttle. The separator, as a key component of LSBs, plays a vital role in preventing internal short circuits and providing transport pathways for ions (Liao et al., 2017). However, the routine separator is not good at restraining the polysulfide shuttle generating from the multi-electron redox reaction within LSBs. In this case, a separator coated with a thin functional layer facing the cathode/anode side has been confirmed to be a successful approach to significantly immobilize the polysulfide species, to improve the sulfur utilization, and to protect the Li anode. Furthermore, various functional separators have different effects on cell performance. Meanwhile, there are several basic parameters for designing advanced functional separators. 1) The thickness of a functional separator directly determines the electronic/ionic transport rate, further influencing the rate capability of LSBs; 2) the weight of a functional separator has an impact on the energy density of a cell; 3) the porosity of a functional separator is highly related to the liquid electrolyte uptake, influencing the energy density and cyclic lifespan of LSBs. Therefore, it is necessary to comprehend the development status and design parameters of functional a separator. In light of the above explanation, research on the process and fabrication design of functional separators for LSBs are elaborately discussed as below.
Functional Separators Facing Cathode
Functional separator facing cathodes (FSFCs) have recently raised significant concerns because they act as the first barrier to restrict polysulfides in the cathode side while making them available to be reutilized, greatly increasing the active material utilization. Various coating layers, including carbon, oxides, metal compounds, polymers, and their composites, have been employed for the modification of commercial separators to endow them with specific functions in LSBs (Fan et al., 2018). Generally, on the basis of the working mechanism, FSFC in LSBs can be categorized into physical absorption functional separators, chemical absorption functional separators, catalytic conversion functional separators, and dual-mechanism functional separators. Meanwhile, it should be mentioned that a satisfactory functional separator requires some intrinsic characters: high ionic conductivity, remarkable shuttle-inhibition ability, superior chemical/electrochemical stability, robust mechanical property, and ultra-lightweight design, etc. The reconfiguration of LSBs with FSFC is illustrated in Figure 2.
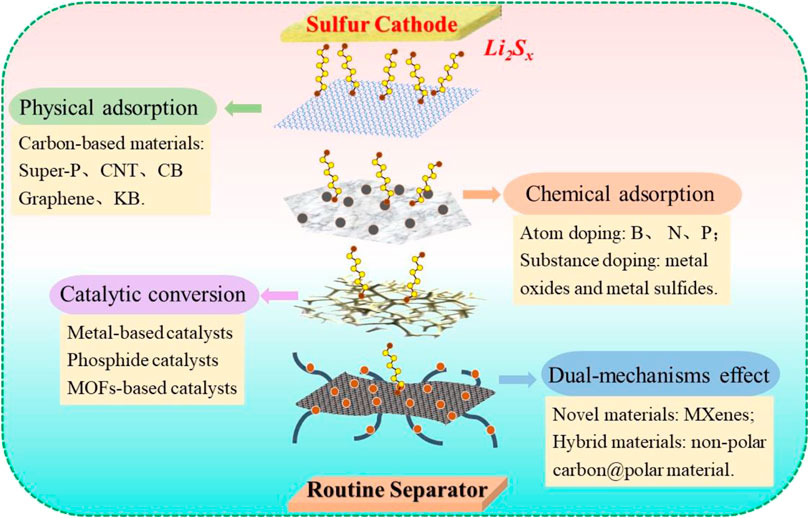
FIGURE 2. Classification and function mechanism of functional separators in the cycling process of LSBs.
Functional Separators Facing Cathode With Physical Absorption Mechanism
Owing to the weak Van der Waals interaction between functional separators and polysulfides, they are considered as physical absorption separators. Initially, within the numerous materials with physical absorption features, carbon-based materials were most popular with researchers owing to their high electric conductivity. The commonly used carbon materials include zero-dimensional (0D) conductive carbon black (Zhao et al., 2016), one-dimensional (1D) carbon nanotube (CNT), (Chung and Manthiram, 2014c), and two-dimensional (2D) graphene (Zhou et al., 2015). It is proven that carbon-coated separators can hinder the polysulfide shuttle, as well as promote the cyclability and rate capability. The enhanced electrochemical performance of LSBs is mainly attributed to the good electric conductivity that urges them to serve as second current collectors to decrease cell resistance and improve sulfur utilization (Chung and Manthiram, 2014b).
Carbon black with low density and cost is extensively applied to fabricate carbon-coated separators. For example, Chung et al. (Chung and Manthiram, 2014a) pioneered a functional separator with a 20 μm of carbon black (Super P) layer (0.2 mg cm−2) onto a Celgard separator (1.0 mg cm−2), shown in Figure 3A. The carbon-coated side of the separator facing the sulfur cathode serves as an obstacle region. This carbon-coating layer affords additional electron pathways for the sulfur cathode, which acts as an “upper” current collector to boost rapid electron transportation. More importantly, the carbon-coated separator is employed as a physical barrier to restrict the polysulfide diffusion (Figure 3B). Moreover, a functional separator exhibits better flexibility and excellent mechanical strength. Thus, the LSBs with such a modified separator deliver high capacity (1,389 mAh g−1 at 0.2 C) with superior cycling stability. Unfortunately, the inefficient polysulfide-trapping property still makes Super P difficult to permanently use in LSBs.
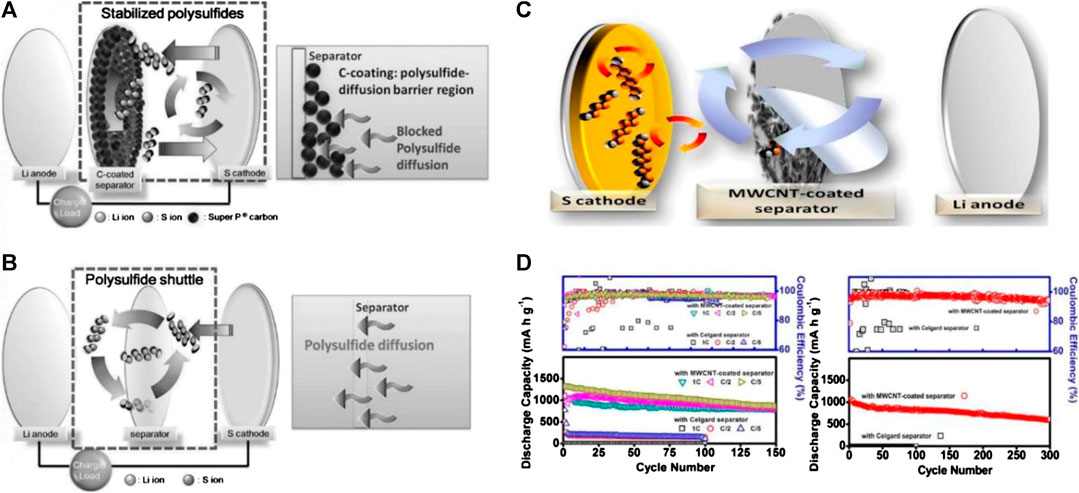
FIGURE 3. Schematic cell configuration modification of LSBs (A) C-coated separator and the polysulfide-diffusion barrier region (B) Celgard separator and the typical severe polysulfide diffusion. Reproduced with permission from (Chung and Manthiram, 2014a) (C) The MWCNT-coated separator (D) Cell performance of the Li−S cells employing the MWCNT-coated separator. Reproduced with permission from (Chung and Manthiram, 2014c).
Analogous to Super P, the porous conductive network of carbon nanotube (CNT) not only plays a filtering role in intercepting polysulfide but also acts as an upper current collector for improving sulfur utilization. More importantly, it can guarantee the reuse of the captured active material when the liquid electrolyte accesses the porous and conductive CNT network. Chung et al. (Chung and Manthiram, 2014c) used multiwall carbon nanotubes (MWCNTs) to modify the cathode side of a Celgard separator as shown in Figure 3C. With the aid of MWCNTs, the functional separator endowed with improved electron transport capability and polysulfide adsorption ability exhibited significantly enhanced sulfur utilization. Notably, the MWCNTs coating layer in the modified separator is ultralight (mass loading = 0.17 mg cm−2), which could still guarantee the long-term cycling performance and the high Coulombic efficiency of high sulfur content (70 wt. %) sulfur cathode in LSBs as shown in Figure 3D. However, just as a coin has two sides, the CNT modified separators also have some demerits. It is not easy to achieve homogeneous CNT coating slurry because of its high specific surface area and large length-diameter ratio. As a result, it is hard to obtain a smooth coating layer on the separator, let alone large-scale application (Huang et al., 2020).
Aside from the above carbon materials, graphene is considered as a charming coating material to ameliorate the properties of the commercial separator. Generally, graphene has good electric conductivity, ultralightweight, excellent ductility, extraordinary mechanical strength, and high chemical stability. The operation mechanism of graphene in a coated separator makes use of its ultrathin layer, blocking the macro-pores of the polyolefin separator, then retarding the diffusion of polysulfides and further reutilizing the sulfur-trapping species. Surprisingly, the areal loading of graphene in a modified separator is often extremely low, but the electrochemical performance of LSBs has a significant improvement. For instance, Zhuang et al. (Zhuang et al., 2016) reported a functional separator decorated by ultra-low loading graphene (0.0035 mg cm−2) via a facile vacuum filtration technique. This rationally designed separator is not only capable of fast Li+ diffusion, but also reserves more polysulfides in the cathode side, leading to high sulfur utilization. In spite of these merits, graphene lacks the ability in physically anchoring the migration of polysulfide upon the long cycling of LSBs.
As described earlier, mono-component carbon presents obvious shortcomings in eliminating polysulfide migration. Recently, multi-component carbon composites, such as graphene/CNT, graphene/carbon fiber, have won considerable success in this respect. The combination of carbon materials occupies certain advantages. On one hand, the porous conductive structure provides the additional electrical contract and the predominant accommodation for polysulfides, thereby efficiently constraining the polysulfide shuttle. On the other hand, the related fabrication technologies (e.g., vacuum filtration, doctoral blade casting, dip casting, etc.) are facile and efficient, and the obtained coating layers are ultralight, which are not prone to influence the gravimetric energy density of LSBs. However, non-polar carbon material can only provide weak physical adsorption to polar polysulfides, which makes it unavailable for high sulfur loading. Additionally, although the areal density is satisfactory (<0.1 mg cm−2), the thickness (15–30 μm) is much higher than routine separators (12–16 μm), leading to the high electrolyte dosage and low gravimetric energy density.
Functional Separators Facing Cathode With Chemical Adsorption Mechanism
Compared to the intensive studies on physical barrier separators, chemical adsorption mechanism-based functional separators are further developed, which are defined as creating chemical bonding between polar substances. For the entrapping of polar polysulfide, polar materials are bound to become an optimal choice due to their powerful force on polysulfides. Normally, chemical adsorption is split into two types: atom doping and substance doping. The former type introduces polar atoms (e.g., B−, F−, N−, Co−, O−, S−, Cl−, etc.) into nonpolar carbon materials to enhance the polysulfide-trapping ability (Huang et al., 2015a). In particular, N/O doping or N-O co-doping is more beneficial tat anchoring polysulfides than B, F, Cl and S (Hou et al., 2016). On this point , Pei et al. (Pei et al., 2018) proposed a bifunctional separator consisted of an N-doped carbon porous nanosheet and a PP separator (G@PC/PP) for LSBs. The N-doped carbon coating has an ultra-thin thickness (0.9 μm) and ultra-low weight (0.075 mg cm−2), which is uniformly distributed onto the surface of PP. The N-doped carbon nanosheet with laminar architecture and an ultrahigh specific area has a key role in increasing the polysulfide-binding ability. The cell with an N-doped carbon nanosheet modified PP separator presents a high initial discharge capacity (1,416 mA h g−1 at 0.05 C) and a remarkable retention capacity (95.1% after 72 h), significantly higher than that of the PP counterpart. To further satisfy cell properties under high sulfur loading, research on co-doped material modified separators is on its way to a discovery, such as N-P co-doped carbon coated on the separator (Zeng et al., 2018). For various atom-doped materials, they should have a strong capture ability for polysulfide so that they can inhibit the shuttle effect. Nevertheless, atom doping only provides weak chemical binding. Meanwhile, doping atoms into a matrix accompanies uneven distribution, which affects the polysulfide adsorption capability. Thus, great endeavors are being made to achieve strong chemical adsorption in the following section.
The latter method called substance doping, with rich polar active sites, unique band structure, and high conductivity, has drawn great attention from researchers in the modification of novel separators for suppressing the polysulfide shuttle. Impressively, metal oxides and metal sulfides have been brought to the foreground. As is well known, metal oxides can produce different bonds to polysulfides. Meanwhile, some metals are prone to losing electrons and turn into ions, which can form chemical bonds to suppress the “shuttle effect” under high sulfur content. In this respect, metal oxides can tightly adhere to the surface of the separator, which are not easy to detach from the separator substrate during long-term cycling. More importantly, metal oxides with nanostructures are beneficial for insertion into various polymers and porous carbon templates to achieve functional separators. Zhang et al. (Zhang et al., 2014) employed aluminum oxide (Al2O3) nanoparticles to modify routine separators for preventing polysulfide diffusion. The well-connected interstitial voids originating from aggregated Al2O3 nanoparticles not only act as the rapid ion-conducting pathway, but could also immobilize the polysulfide within the cathode region by electrochemical deposition. The cell with an Al2O3 coated separator exhibited a high reversible capacity of 593 mA h g−1 after 50 cycles at 0.2 C with nearly 100% Coulombic efficiency. Metal sulfides, containing ionic bonding, contribute to forming robust chemical bonds with polysulfide and afford more ionic passageways. Besides, the features of their native defects cooperating with chemically active surfaces are helpful to enhance the conductivity of the electron transport (Deng et al., 2019). Ghazi et al. (Ghazi et al., 2017) prepared a MoS2/Celgard separator as an efficient polysulfide barrier for LSBs (Figures 4A,B). Thanks to the Mo-Sn2− interaction disclosed by XPS (Figure 4C), the stacked structure of MoS2 supplies free spaces to store polysulfide via chemical interaction, leading to a significantly enhanced electrochemical performance better than a Celgard separator (Figure 4D).
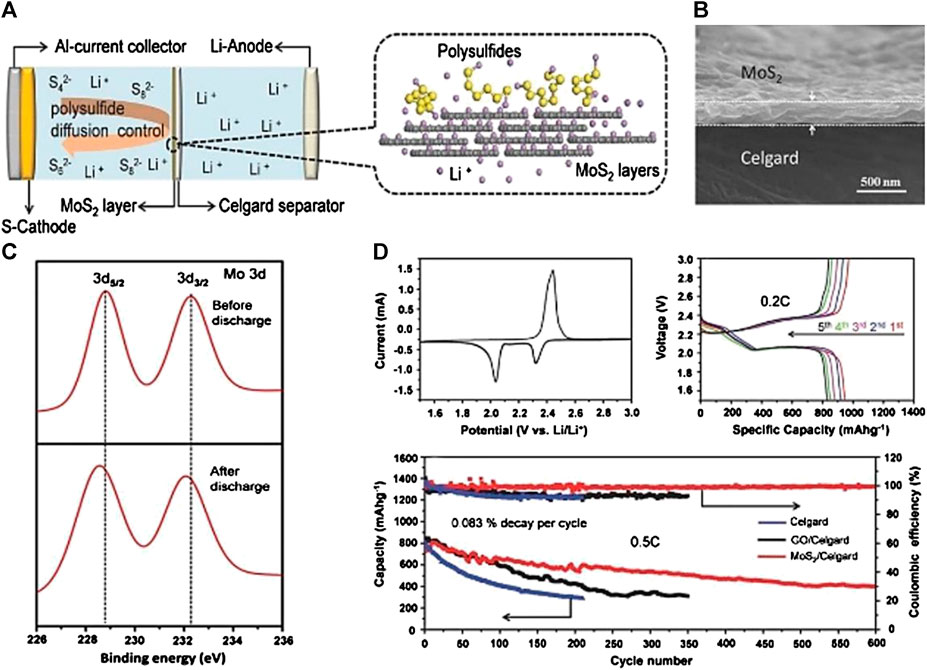
FIGURE 4. (A) Schematic cell configuration and (B) cross section of the MoS2/Celgard separator in LSBs (C) X-ray photoelectron spectroscopy of 3 days peaks of Mo before/after charge-discharge (D) Cyclic voltammetry profile and Galvanostatic charge–discharge profiles of the cells with MoS2/Celgard separator, and Long life cycle test for various separators in LSBs. Reproduced with permission from (Ghazi et al., 2017).
Nevertheless, substance doping still occupies a certain space to realize long-life LSBs due to the sluggish reaction kinetic and inferior conversion rate of long-chain polysulfide to short-chain sulfides. Meanwhile, the thickness and weight of the metallic compound coating layer (100∼200 μm, 1.5∼3.0 mg cm−2) are much higher than the carbon coating layer (15∼30 μm, 0.03∼0.1 mg cm−2), leading to additional weight gain, and further sacrificing the gravimetric energy density of the cell. Thus, it is necessary to make a balance between the properties of the metallic-based coating layer (thickness, weight) and the energy density of LSBs.
Functional Separators Facing Cathode With Catalytic Conversion Mechanism
To acquire high energy density LSBs, the relationship of sulfur loading/content and sulfur utilization should be balanced. It is necessary that soluble polysulfide is not only “passively” sieved, but can also be reactivated (Liu et al., 2018). However, FSFC based on physical or chemical adsorption can not meet this requirement. The physically/chemically-adsorptive materials only play a low effective role for the efficient utilization of polysulfide. Notably, the weak contact between the sulfur cathode and polysulfides resulting from the dissolution of the polysulfides into liquid electrolytes normally leads to more sluggish reaction kinetics of S8 to Li2S (Deng et al., 2019). And the “dead sulfur” will continue to cover the surface of the cathode, causing the loss of sulfur. Under this circumstance, effectively boosting the soluble polysulfide conversion to insoluble Li2S2/Li2S during the discharge process is essential for removing the polysulfide shuttle. In addition, in the charging process, activation energy of solid Li2S2/Li2S back to polysulfide should be enough for guaranteeing fast reaction kinetics (Li et al., 2019b). In general, the lower the activation energy is, the higher the conversion rate is, which could fully exploit the role of catalysts. Different from physically/chemically-adsorptive materials, the catalytically active materials can improve the rate of the oxidation reduction reaction to accelerate the conversion of polysulfides to Li2S2/Li2S, further addressing the root problem of the polysulfide shuttle in LSBs. Because the polar catalysts can provide abundant exposed sites, it is helpful to seize the dissolved polysulfides, and to shift electrons from the catalysts to the exposed sites at the surface interface, and further transfer to the S-S bond of polysulfides, which promotes the conversion of polysulfides. Moreover, the intrinsic catalytic effect of the catalysts can reduce the reaction barrier and the chemical overpotential of sulfur species, accelerating the electrochemical reactions of sulfur (Yuan et al., 2019). Therefore, the study on catalytic conversion of polysulfide to Li2S2/Li2S is a non-negligible direction for achieving high sulfur utilization for LSBs. Until now, the catalysts of decorating separator for strengthening the redox reaction rate mainly include metal-based catalysts, phosphide catalysts and metal-organic frameworks (MOF)-based catalysts, etc.
For instance, Qian et al. (Qian et al., 2017) prepared a functional separator by coating hollow spherical La2O3 with a lightweight of 0.4 mg cm−2 and a thin thickness of 15 μm. The LSBs matched with the La2O3-coated separator exhibit a high rate capacity and remarkable capacity retention. The enhanced electrochemical performance is ascribed to the La2O3 functional layer that not only effectively captures polysulfide, but also acts as a chemical catalyst upon the sulfur redox reaction. As a typical representative of phosphide, cobalt phosphide (CoP) can catalyze the conversion of polysulfide and dramatically increase the sulfur utilization. Lin et al. (Lin et al., 2020) modified a PP separator by co-coating CoP and carbon on the surface of a PP separator as illustrated in Figure 5A. According to the XPS results (Figure 5B), the CoP/C-coated separator could localize the insoluble polysulfide on the cathode by strong interactions between Co. atoms derived from CoP and S atoms in the Li2S6 solution. Moreover, it is emphasized that CoP/C can facilitate the catalysis of polysulfide reduction/oxidation due to the fast transfer at the interface. Finally, the rapid redox kinetic, high conductive performance, and outstanding cyclic capacity are presented in Figure 5C.
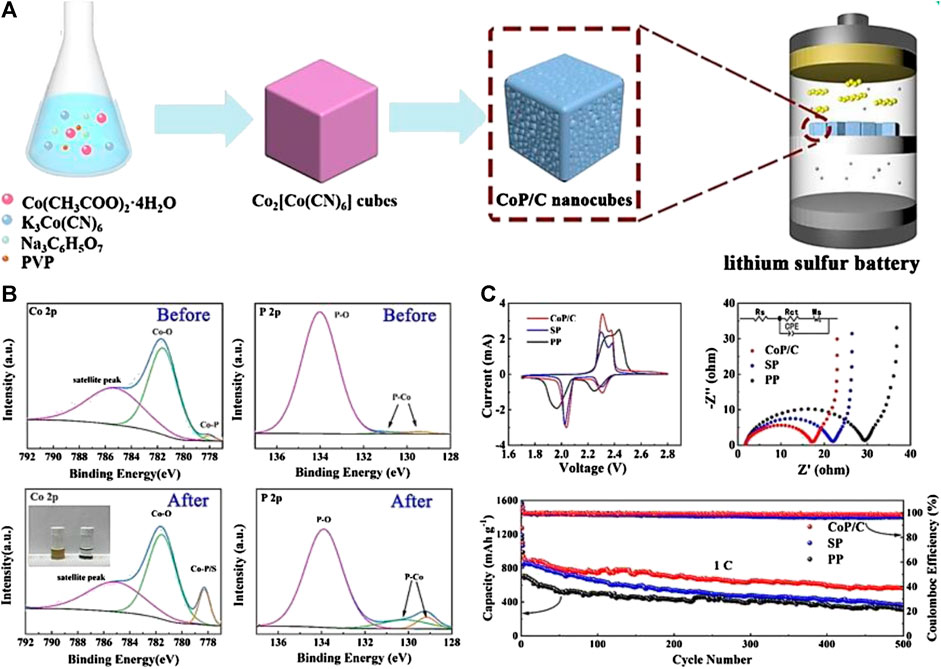
FIGURE 5. (A) Schematic illustration of fabrication procedure of CoP/C nanocube and cell configuration of the LSBs employing the CoP/C coated separator (B) High-resolution XPS spectrum of Co 2p and P 2p before and after CoP/C interacting with Li2S6(C) Performance comparison of LSBs with different separators. Reproduced with permission from (Lin et al., 2020).
Recently, MOF-based materials as the templates of nanocomposites have raised great concerns depending on their functions in batteries. 1) Large specific surface area for filtering polysulfides and further preventing polysulfides from cathode to anode; 2) highly ordered pores with tunable porosity for governing the electrolyte uptake and unique functional groups for promoting catalytic redox conversion. Song et al. (Song et al., 2020) reported 3D catalytic MOF-derived CNTs and N-doped carbon nanosheets (NCNS) as well as Co. nanoparticles (abbreviated as 3D Co./NCNS/CNT) as the coating layer of a separator for LSBs. These decorated separators have excellent sulphiphilicity, good conductivity, and marvelous catalytic activity from large numbers of Nx and Co. nanoparticles. It was demonstrated that these functional separators with high adsorption to polysulfides could rapidly catalyze the polysulfide conversion, leading to the superior cycling stability of LSBs with a high sulfur loading of 5 mg cm−2.
All in all, to realize fast catalytic conversion, most catalysts coated on functional separators should have high electronic conductivity, large surface area, and outstanding catalytic ability. Actually, metal-based and phosphide-based catalysts tend to be granular nanostructures, which are not conductive enough to convert lithium polysulfide. In contrast, MOF-based catalysts have unique 3D conductive frameworks that will facilitate the catalytic transformation of polysulfides, which gradually makes it fully feasible for the future development trend of LSBs. However, such coating layers with a fast conversion rate may reduce the efficient transfer of Li+ (Tan et al., 2018). Additionally, these catalysts frequently involve the use of toxic solvents or reagents (eg, DMF, Co.), thereby we should seek alternative green solvents to synthesize these catalysts.
Functional Separators Facing Cathode With Dual-Mechanisms Synergistic Effect
To further amplify the working efficiency of FSFCs, scholars rationally design dual-mechanisms materials with perfectly inherited characters from parental materials. Recently, the synergistic effect of physical adsorption and chemical immobilization has become a forceful strategy to restrain polysulfides, which not only significantly improves sulfur utilization, but also is favorable for increasing the energy density of LSBs. As shown in Table 1, metal oxides, carbon nanofibers, and transition-metal carbides have been widely employed to decorate routine separators owing to their intrinsic nanostructure with a strong polysulfide-trapping ability. Li et al. (Xiang et al., 2018) fabricated an SnO2 functionalized separator, which presented a more pronounced electrochemical performance than that of a PP separator. The advantages of the utilization of SnO2 to modify separators are discussed below: 1) The SnO2 layer has a negligible effect on the weight and volume of the PP separator; 2) the nano-skeleton of a coated separator facilitates the fast Li+ transfer, as well as physically blocks the migration of polysulfides. 3) The chemical interaction of SnO2 to S in polysulfides further prevents the against “shuttle effect.” Therefore, it is easily concluded that metal oxides as coating materials allows for the fine governing of the thickness and porosity of coating layers and further influences the Li+ transfer number and polysulfide-capturing ability.
Meanwhile, carbon nanofiber coatings have effectively positive roles in limiting polysulfide shuttles by dual-effects. Due to the high strength to weight ratio, adjustable microarchitecture, and facile preparation, carbon nanofibers are prone to an ideal specific surface area, porosity, and size, determining the capability of sieving polysulfides. Moreover, according to these features, coating technology can easily access functional separators with ultrathin thickness, high mechanical strength, and good flexibility. Chung et al. prepared a microporous activated carbon nanofiber (ACNF)-coated PP separator for LSBs. The ACNF coatings with small micropores (0.4∼1.2 nm) and high porosity can effectively intercept polysulfides via the chemical/physical interaction of ACNF towards polysulfides. Moreover, the ACNF-coated separator maintained its initial shape well even after being folded and crumbed, implying its robust flexibility and mechanical durability. Owing to these merits, ACNF-coated separators exhibited remarkable sulfur utilization and a low polysulfide shuttle. Benefiting from a ultrahigh conductivity and highly active 2D structure, MXenes are of interest in versatile applications (Naguib et al., 2011). In particular, the highly active 2D surface of MXenes can chemically anchor polar polysulfides through the metal-sulfur interaction in LSBs (Liang et al., 2015). Song et al. (Song et al., 2016) fabricated a functional separator by coating Ti3C2Tx MXene nanosheets (T: O, −OH, and −F) on PP. Owing to the ultrathin 2D structure, the mass loading of this uniform Ti3C2Tx MXene coating layer was only 0.1 mg cm−2. In conjunction with its high conductivity, the Ti3C2Tx MXene coating layer served as second current collector to weaken the internal resistance of the cell. Meanwhile, the highly active polar surface of Ti3C2Tx MXenes could immobilize polar polysulfides within the cathode side by the synergistic effects of physisorption and chemisorption. As a result, the polysulfide shuttle is suppressed, which remarkably prolongs the cyclability of LSBs.
Although the above-mentioned novel materials have superior physical and chemical absorption ability to anchor polysulfides, the preparation process often involves complex chemical reactions, expensive raw materials, and high consumption of time and energy. Therefore, with the exception of studying novel materials, considerable explorations are focused on hybrid materials since the fabrication of these materials mainly adopt simple mechanical mixing and facile wet impregnation, without energy consumption. Generally, the blend of non-polar carbon materials and polar materials is a mainstream trend for restricting polysulfides, such as carbon black-based coating (Qian et al., 2016) and CNTs-based coating (Liu et al., 2017a). Yang et al. (Yang and Zhang, 2018) created a laponite nanosheets/carbon black (LNS/CB)-coated Celgard separator to retard the polysulfide shuttle via physical/chemical adsorption, with detailed information in Figure 6. Such a nanolayered skeleton not only endows the separator with high ionic and electronic conductivity, but also provides extensive O active sites (e.g., −OH groups, O atoms) and large specific surface area (323.3 m2 g−1) to reactivate and reutilize the captured polysulfides by O-Li and O-S bonds (Figures 6A–D). In addition, the LNS/CB layer has a uniform distribution, ultrathin thickness (∼3.5 μm), excellent flexibility, high thermal stability, and good electrolyte wettability compared to PP (Figures 6E–G). Consequently, the cell assembled with an LNS/CB-coated separator exhibits an ultralow capacity fading rate. Furthermore, Kong et al. (Kong et al., 2017a) proposed a nest separator integrated by PP, CNTs, and MgBO2(OH) nanofibers. CNTs provide more conductive pathways to boost electronic transportation, which is favorable for the fast redox conversion of polysulfides. Benefiting from the large surface area, plentiful sulphifilic groups (O2−, −OH) and special 1D nanofiber structure, MgBO2(OH) has adequate chemical sites that trap polysulfides by strong chemical adsorption. Besides, together rigid MgBO2(OH) and flexible CNTs render the modified separator with robust and durable mechanical performance. The combined features of MgBO2(OH) and CNTs facilitate a long lifespan and excellent rate capability of LSBs.
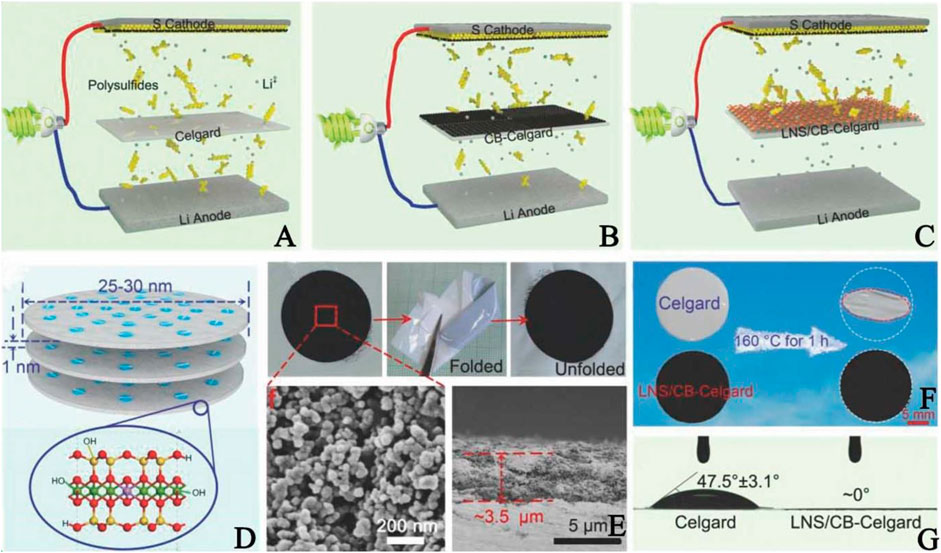
FIGURE 6. Schematic illustrations of LSBs with the (A) Celgard (B) CB-Celgard (C) LNS/CB-Celgard separators, and (D) the structure of the LNS (E) Photographs, SEM and cross-sectional images of the LNS/CB-Celgard separator (F) Dimension shrinkage of the separators after storage at 160°C for 1 h (G) Contact angles tests. Reproduced with permission from (Yang and Zhang, 2018).
Functional Separators Facing Cathode With Other Mechanism
Considering that the shuttle effect originates from the migration of dissolved polysulfides, the coating layer with ion-selective behavior on the separator could afford an effective approach to manage the polysulfide penetration but simultaneously allow facile Li+ transfer in LSBs (Li et al., 2020). So far, many coating materials, such as Nafion (Huang et al., 2013), MOF-based materials (Bai et al., 2016), polar vanadyl phosphate (VOPO4) sheets (He et al., 2019a), and lignosulfonate (SL) (Lei et al., 2018), have been employed to realize the charge-adsorption/repulsion of polysulfides and further to immobilize polysulfides on the cathode side. For instance, Huang et al. (Huang et al., 2013) fabricated an ion selective separator by coating a Nafion solution on a Ceglard 2,400. The sulfonate (SO3−) group-coated channels in the ion selective separator have permselectivity, which allow the free transportations of charged cations (Li+), as well as block the diffusion of polysulfide anions (Sn2−). Therefore, the polysulfide shuttle is greatly suppressed, leading to enhanced cycling stability. Although the preparation method of the ion selective layer is facile and cost effective, the low permeation speed makes it difficult to satisfy high mass loading sulfur cathodes, which still need to be further improved.
In summary, each mechanism has its own merits and demerits. Among them, the functional separators modified by hybrid materials can physically/chemically interact with polysulfides, leading to enhanced polysulfide absorption ability. More importantly, only employing a thin (<10 μm) and light (0.01∼0.4 mg cm−2) hybrid material coating layer can successfully fulfill high sulfur loading and cathode content, and further realize excellent rate capability and the remarkably long-term cyclability of LSBs. Thus, to form a high-barrier performance separator, an ideal hybrid material should have the following features. 1) Abundant electron pathways are beneficial for the fast Li+ diffusion; 2) an adjustable nanostructure facilitates to obtain appropriate thickness, lightweight, high porosity, good mechanical property, and electrolyte wettability property; 3) unique hydrophilic/sulphiphilic groups can chemically interact with Li or O atoms in polysulfides to eliminate the “shuttle effect”; and 4) the fabrication methods of functional separators need be cost-effective, eco-friendly, and available in large-scale. In light of above standards, these hybrid material-coated separators can be feasible for high energy LSBs.
Functional Separators Facing Anode
Apart from the severe polysulfide shuttle, the formation and growth of Li dendrites upon the redox reaction process severely restricts the practical application of LSBs. In order to solve this issue, researchers focus on the prohibition of Li dendrites. The Li dendrites in LSBs mainly arise from the continuous side reaction between Li metal and polysulfides in electrolytes. Therefore, compared with FSFC, a functional separator facing anode (FSFA) plays the second barrier role in blocking polysulfides, suppressing parasitic reactions, and preventing Li dendrites, which contributes to the cyclability of LSBs (Yan et al., 2016). Generally, there are some design considerations for FSFA. 1) FSFA can engender low interfacial resistance and excellent interface compatibility with the Li anode; 2) FSFA can further suppress the “shuttle effect” and serve as a physical barrier for restraining dendritic Li growth concurrently, further improving the Li deposition/dissolution rate; 3) FSFA can induce the growth of Li dendrites along special channels by specific structures to avoid puncturing the separator; and 4) FSFA can provide the lithiophilic scaffold for regulating Li nucleation protecting the Li anode. It is worth noting that the design principles of FSFC and FSFA still have some differences. Apart from the basic design parameters including thickness, weight, porosity, mechanical strength, and electrolyte wettability, compared with FSFC, FSFA not only has a hinder polysulfide shuttle capability, but also should have special lithiophilic skeletons to rationally control the spatial distribution of Li deposition and further regulate the interfacial behaviors of the Li anode, prohibiting the growth of dendritic Li (Peng et al., 2017). More importantly, FSFC and FSFA can synergistically improve the electrochemical performance of LSBs since FSFC mitigates the migration and diffusion of polysulfides, whereas FSFA acts as the secondary defense to obstruct the leaked polysulfides from the cathode side to the anode side, which concurrently protects the Li anode from unexpected side reactions. According to the working roles of the barrier layer against the Li dendrite, FSFA are divided into four types, which will be elaborately demonstrated in the following sections.
Functional Separators Facing Anode With Physical Barrier
The stable SEI film on the surface of Li metal is aiming at forming homogeneous lithium stripping/plating behavior for the Li anode, which determines the life cycle, energy density and safety of LSBs. At the anode side, similar to the SEI film, the modified separator acted as a physical barrier to impede the immigration of soluble polysulfides from the cathode to anode and the formation of Li dendrites, which could improve the Li deposition/dissolution and Coulombic efficiency. Since the concept of a modified separator for protecting Li anode was proposed, a wide variety of materials have been innovatively adopted, including carbon-based materials and hydrophilic polymers. Typically, various nanofibers coated on the anode side of the separator can successfully inhibit the lithium dendrite growth since they are able to form a uniform distribution of Li+ flux (Nie et al., 2019). Additionally, some polymers have multiple merits, in terms of boosting electron transportation, realizing uniform Li ionic flux, as well as arresting polysulfides. Li et al. (Li et al., 2019c) fabricated a conductive polypyrrole (PPy) nanofilm on the surface of a commercial separator facing anode to suppress the polysulfide shuttle and to stabilize the Li metal anode. Due to the intrinsic hydrophilicity of PPy, it facilitates well-distributed Li plating/stripping at the anode side and homogenous Li ionic flux, thereby physically inhibiting the formation of Li dendrites. Moreover, PPy chemically immobilizes the dissolved polysulfide via its special structure and functional groups. Meanwhile, the PPy layer with lightweight (∼0.13 mg cm−2) and thin thickness (∼65 nm) has a negligible loss for the energy density of LSBs. With these advantages, paired with a PPy-coated separator, the Li||Li symmetric cells remain stable during Li plating/stripping cycling over 250 h, and the LSBs exhibit high capacity retention.
Except for a separator modified by materials without reacting any components in LSBs, the way of fabricating a “creative” physical barrier through introducing a lithium compound as a coating layer on a separator is another effective strategy to suppress the side reactions between polysulfides and metallic lithium. Pioneering research demonstrated that lithium fluoride (LiF) has quite a low solubility in ether electrolytes (McBreen et al., 2000). Also, the Lewis acid Li atoms in LiF can interact with 1,2-dimethoxyethane (DME) as Lewis alkali to produce DME-LiF clusters, which form a dense and viscous sol layer as a physical barrier to block the polysulfide shuttle, and further to protect the Li anode. Li et al. (Li et al., 2017) proposed a functional separator coated with LiF (thickness = 7 μm) to ameliorate the electrochemical performance of LSBs. In the presence of LiF and DME, a semitransparent and highly viscousity sol coating layer is formed, which undoubtedly decreases the migration of polysulfides. The high surface energy of LiF could make for the inhibition of lithium dendrites. As a result, LSBs employed with a LiF-coated separator exhibited high energy density and excellent safety. Obviously, using these well-designed decoration layers on separator towards the Li anode not only make it possible to confine polysulfides, but also offer a promising approach to construct stable protection for the Li anode. However, the ultrathin coating layer is prone to peel from the separator during long-term cycling, which will gradually weaken the ability to block Li dendrites. Thus, it is important to understand the shortages and failure mechanism of coating materials in overcoming dendritic Li of LSBs.
Functional Separators Facing Anode With Induced Lithium Growth Mechanism
For a metallic Li anode, the infamous “tip effect” of lithium plays a fatal role in deteriorating the life cycle of LSBs. To overcome this problem, the majority of researchers put forward unique architecture designs to induce the uniform growth of Li for restraining Li dendrites. These special structures provide abundant and ordered space so that they have a strong “magnet force” to induce Li growth, which significantly enhances the safety of LSBs (Deng et al., 2019). In this context, Li ions smoothly and uniformly pass across the modified separator and transfer to the Li anode without the intervention of anions so that a dendrite-free Li anode is achieved. Up to now, various carbon-based nanomaterials with hierarchical porous structures have been used to modify commercial separators, which endow the separators with the remarkable ability to prevent the growth of Li dendrites.
It is worth mentioning that the Li dendrite grows slowly on the anode at a low current density, the conductive and porous skeletons herein are devoted to providing uniform Li+ flux near the anode surface (Zheng et al., 2014; Shin et al., 2015; Zhang et al., 2016). Owing to the highly conductive 3D porous network, CNF not only guides Li+ to uniformly deposit along with the fiber framework, which could prevent the “tip effect” of Li and internal short circuits, but also anchors the dissolved polysulfide to promote the utilization of sulfur species (Huang et al., 2015b). Wang et al. (Wang et al., 2017) used CNF as a 3D coating layer to modify both sides of a routine separator. The smooth surface morphology of the Li anode was clearly observed after long-term cycling, implying that 3D CNF-coated separators were favorable to improve the safety of LSBs.
Additionally, Huo et al. (Huo et al., 2020) proposed a composite-solid-electrolyte (CSE) layer consisting of polyvinylidene fluoride (PVDF) and Li6.4La3Zr1.4Ta0.6O12 (LLZTO) to modify the anode side of a PP separator for the dendrite-free Li anode. The 3D Li+ channels in CSE could effectively promote the even Li+ flux, leading to the uniform Li deposition because of the interaction with PVDF and LLZTO. Additionally, the higher transference number (t+) of the CSE layer can localize more anions, further regulating the smooth Li+ deposition. By the synergetic working mechanism of Li+ redistribution and anion immobilization, LSBs exhibited higher Coulombic efficiency and better safety.
Functional Separators Facing Anode With Nucleation Regulation Mechanism
Recently, nanoparticle or nanolayer-based materials have become in inhibiting the dendritic Li growth by controlling lithium nucleation (Deng et al., 2019). The nanoparticles in FSFA act as heterogeneous nucleation sites of Li by decreasing the Gibbs free energy of Li electrodeposition, which makes Li preferentially deposit on these nanoparticles to form regular Li crystal seeds in the early plating stage. During the plating process, Li crystal seeds gradually cover the whole separator surface, and finally form into a dense Li metal layer with dendrite-free morphology, resulting in a uniform Li anode without any growth of Li dendrites. (Liu et al., 2019). In addition, the voids and pores formed by nanoparticle accumulation can provide additional channels for transporting more Li+ ions, which could significantly enhance the redox kinetics of LSBs. Apart from regulating lithium nucleation, the thin films constructed from inorganic and oxide materials are helpful to confine the polysulfides as well.
He et al. (He et al., 2019b) fabricated an Al2O3 thin film as a protective layer onto a commercial separator facing the anodic side to address the obstacles of Li dendrites. First of all, the Al2O3 layer suppresses the parasitic reactions between the soluble polysulfide and lithium metal. Secondly, the porous structure of the Al2O3 layer guides the uniform nucleation of lithium and further hinders the growth of Li dendrites, leading to a smooth and dense Li anode. Thanks to the merits of the Al2O3 layer, LSBs show high sulfur utilization and superior cyclability with high sulfur loading. Moreover, due to the intrinsic insulation and high thermal conductivity, boron nitride (BN) is often used to modify the commercial separators. Kim et al. (Kim et al., 2017) prepared the BN (∼7 μm)/carbon (∼6 μm) modified separator with synergistic protective effect, in which BN and carbon were coated on the anodic side and cathodic side of the separator, respectively. The morphology of the BN coating layer and the corresponding schematic illustration of the roles of the BN coating layer in the modified separator are presented in Figures 7A–G. Apparently, with the surface modification of the BN coating layer on the separator, the Li anode has a uniform Li plating/striping process, dramatically alleviating the hazardous Li dendrites (Figure 7H). In addition, the BN layer acts as an extra line of defense to obstruct the leaked polysulfide. Therefore, the LSBs assembled with BN modified separators present superior electrochemical properties (Figure 7I).
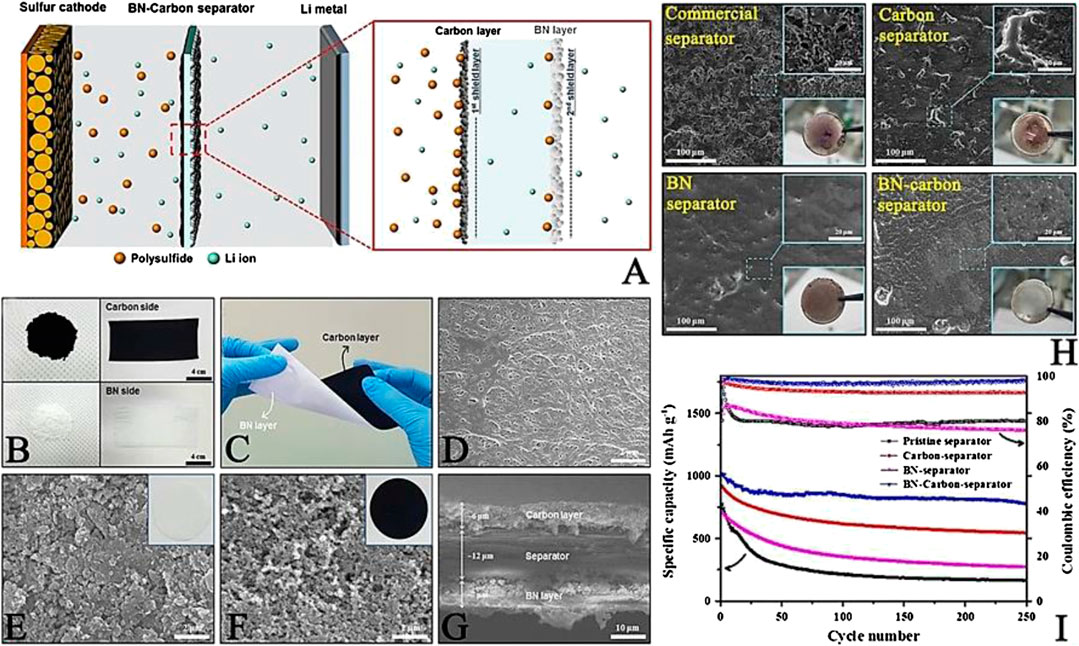
FIGURE 7. (A) Schematic illustration of the systemic role of a BN-carbon separator in the discharging process (B) photographs of BN-coated separator (C) image of a twisted BN-coated separator. SEM images of (D) pristine separator (E) the BN side and (F) the carbon side of a BN-coated separator (G) Cross-sectional view of a BN-coated separator (H) Photographs and morphologies of lithium metal anode after 250 cycles using different separators (I) Cycle performances of various separators at 0.5 C. Reproduced with permission from (Kim et al., 2017).
Wu et al. (Wu et al., 2018) proposed ultralight dual-functional separators, in which two sides of this functional separator were coated by a molybdenum disulfide/poly (diallyl dimethyl ammonium chloride) hybrid in conjunction with poly (acrylic acid) (MoS2/PDDA-PAA, denoted as M-P/P), as shown in Figures 8A–C. Compared with Celgard separators, the cells employed with this rationally designed M-P/P-coated separator exhibited superior electrochemical performance after 500 cycles at 1 C since only trivial “dead” Li and tiny particles (sulfur and sulfides) can be detected on the surface of the M-P/P-coated separator (Figure 8D). This is mainly attributed to the following aspects: 1) the robust and Li+ conducting MoS2 layers are beneficial for enhancing the modulus of the separator and regulating Li deposition as well as restraining dendrite growth. 2) The tightly packed “nanobrick wall” structure of the M-P/P-coated separator leads to rapid Li+ flux, relieving Li+ depletion and obtaining homogeneous Li deposition. 3) MoS2 nanosheets and PAA play catalysis and chemisorption roles in anchoring polysulfide, respectively. Benefiting from the above advantages, the sulfur utilization of LSBs with an M-P/P-coated separator at various C-rates are superior to cells with Celgard separators (Figure 8E).
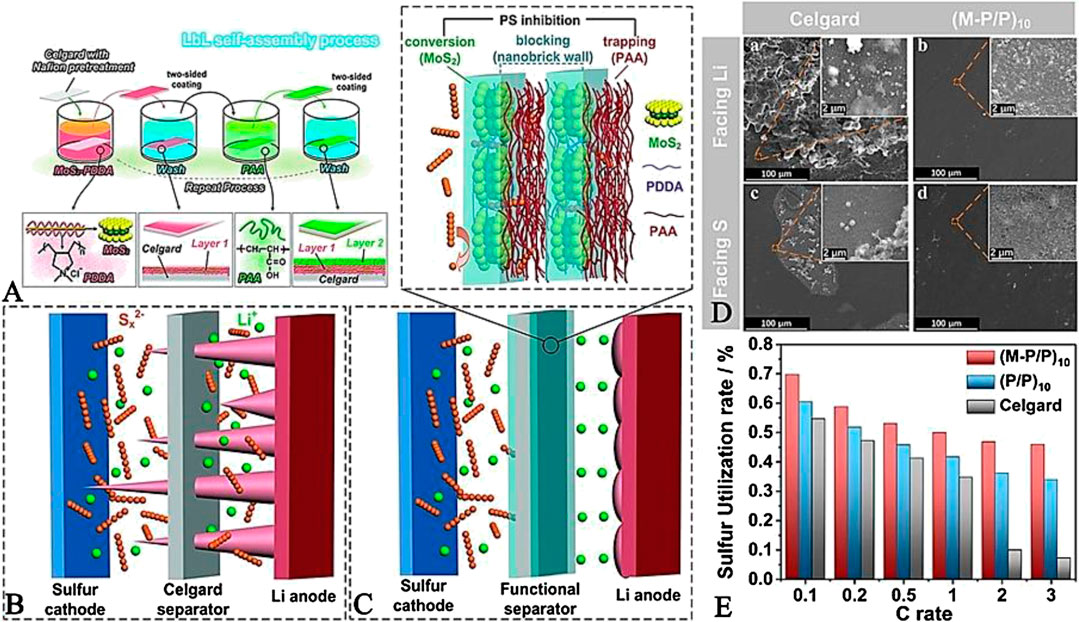
FIGURE 8. (A) Schematic showing the M-P/P-coated separators self-assembly process (B, C) Schematic illustrations of the LSBs with Celgard and M-P/P-modified separator (D) SEM images of Celgard separator and M-P/P coated separator adjacent to Li anode and sulfur cathode after 500 cycles at 1 C (E) Sulfur utilization rate at various C-rates for different separators. Reproduced with permission from (Wu et al., 2018).
Compared to the induced lithium growth mechanism, the materials applied to regulate lithium deposition by nucleation regulation also have great advantages even if there is no special skeleton or directional channel. Moreover, these low cost and naturally abundant materials can be added directly into the coating layer. In addition, the coating layers consist of nano-materials and PVDF (ranging from 20 to 30 wt. %), undoubtedly deteriorating the lifespan of the separator. Meanwhile, the toxic and expensive solvents (e.g., NMP) participate in the preparation process, causing environmental risks. Thus, we should choose a high-performance aqueous binder with about 5 wt. % to improve the structural integrity of the coating layer, finally achieving a powerful guidance to lithium.
Functional Separators Facing Anode With Hybrid Mechanism
In fact, all the functional separators can serve as physical barriers. In order to further amplify the effects of modified separators for protecting the Li anode, the combination of the induced lithium growth and nucleation regulation mechanism with physical barriers is a promising strategy to repress lithium dendrites and to block the leaked polysulfides. For this reason, materials with unique structures and hybrid mechanisms should be carefully designed. Lithium nitrate (LiNO3) is extensively used as an additive in electrolytes to inactivate the Li metal anode as well as to diminish the parasitic reactions between the dissolved polysulfides and lithium (Xiong et al., 2014). However, LiNO3 will be exhausted during long-term cycling because of its irreversible reaction with lithium. Owing to the above limitations of LiNO3, Guan et al. (Guan et al., 2018) designed a modified separator with a LiNO3/Al2O3/PVDF coating layer (thickness = 4 μm, mass density = 0.53 mg cm−2) facing the Li metal anode. In this tri-layer separator, each component utilizes a particular functionality. The LiNO3 not only favorably forms a stable passivation layer on the surface of the Li metal anode, but also has excellent ionic conductivity to control the lithium nucleation and deposition. Al2O3 nanoparticles are capable of to storing LiNO3, maintaining the durability of LiNO3. Impressively, the closely packed Al2O3 improves the specific surface area of the separator, realizing the homogenous Li infusion and deposition via the uniform nucleation mechanism. More importantly, PVDF not only ensures the integrity of the coating layer without cracking in the presence of the interface fluctuation during the charging and discharging process, but can also afford reasonably induced lithium growth. By the dual-working mechanisms of a LiNO3/Al2O3/PVDF-coated separator, the dendrite-free Li anode and uniform Li+ flux can be achieved, leading to the enhanced electrochemical performance of LSBs compared to the counterpart commercial separators.
However, until now, only some published literature have concentrated on functional separators with dual-mechanisms related to the protection of the Li metal anode. The following several reasons account for this issue. Firstly, the immaturity of the preparation process is prone to cause the uneven distribution of coating materials on the separator, influencing the transfer number of Li+ and uniform Li+ flux. Secondly, the hybrid mechanism combined with the induced lithium growth mechanism and nucleation regulation mechanism still hardly guarantee a the dendrite-free Li metal anode due to the insufficient channels, low conductivity, and poor mechanical strength. In addition, the coating materials should be at the nanometer scale, which will increase the manufacturing cost.
To sum up, the design and fabrication of coating materials with the function of inhibiting dendritic lithium growth should be considered in depth. Importantly, the coating materials with the most outstanding synergistic effect can provide strong polysulfide-confining ability, homogenous Li+ flux distribution, and rapid reaction kinetics of polysulfide conversion.
Relationship of Design Protocols and Electrochemical Performance of Functional Separators
Here, we analyze the reported functional separators in terms of their loading, thickness, and initial discharge capacity in recent years. As illustrated in Figure 9, the loading and thickness of functional separators are mainly distributed in purple circles ranging from 0.15 to 0.4 mg cm−2 and 5∼15 μm, respectively. Most of the LSBs assembled with these functional separators demonstrate high initial discharge capacity. However, only a few of them still have low capacity since they merely serve as physical barriers that provide a weak physical adsorption ability to polysulfides. Therefore, in order to achieve high electrochemical performance of LSBs, the rational balance among the loading, thickness, and functions of separators should be further optimized in sector graphs.
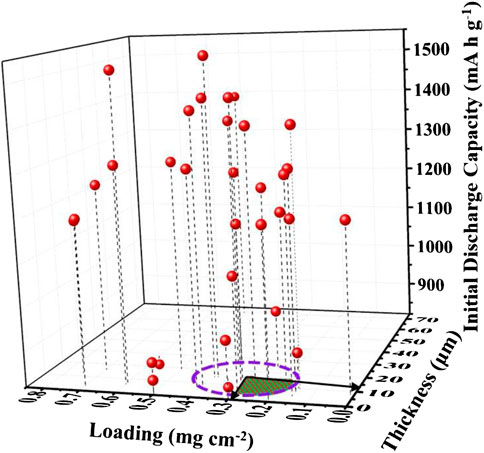
FIGURE 9. The relationship between the loading and thickness of functional separators and the corresponding initial discharge capacities of LSBs.
To better demonstrate the importance of the design parameters of functional separators, a group of estimated parameters in terms of the reasonable relationship between the cell energy density and loading, thickness of the coating layer and electrodes as well as the dosage of electrolytes (electrolyte/sulfur ratio, E/S ratio) are provided. Taken a five Ah pouch cell as an example, the energy density can be calculated by Eq. 1 with the cell parameters as listed in Table 2. Notably, only the LSBs with the optimized loading and thickness of both electrodes and the functional separator can achieve a high energy density, which is much higher than that of commercial LIBs (<300 Wh kg−1).
Meanwhile, the relationship between the coating mass and thickness of functional separators and the energy density of LSBs is illustrated in Figure 10. As the coating mass and thickness of the coating layer gradually decrease (<0.1 mg cm−2, <1 μm), the gravimetric and volumetric energy density of LSBs can reach to 470 Wh kg−1 and 610 Wh L−1, respectively. The increased energy density is mainly attributed to the advantages of functional separators with ultrathin thickness, ultralight weight, and suitable porosity, which not only have a negligible weight grain for the whole cell, but also reduce the dosage of liquid electrolyte. Therefore, in response to the satisfactory energy density of LSBs, the coating mass and thickness of functional separators need to be carefully balanced.
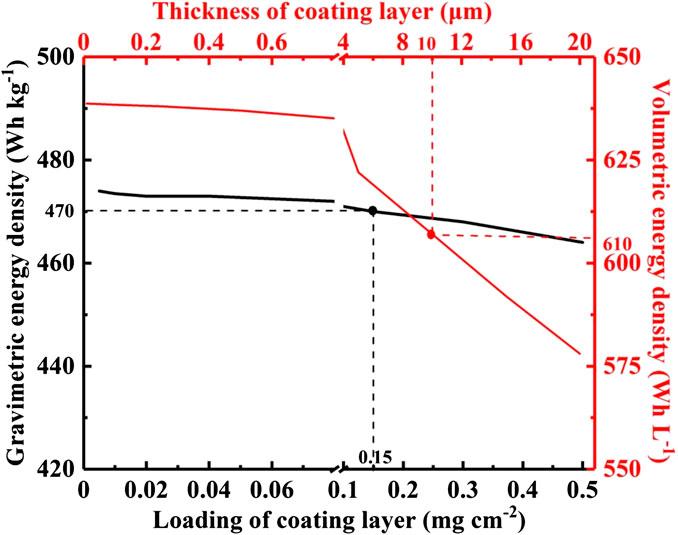
FIGURE 10. The influence of the coating mass and thickness of functional separators on the energy density of LSBs.
Undoubtedly, fabrication methods are critical when realizing the rationally designed functional separators for high energy density LSBs. Herein, the merits and demerits of the currently reported fabrication methods of functional separators are summarized. As illustrated in Figure 11, slurry coating, vacuum filtration, and dip-casting are prevailing strategies to prepare surface modified functional separators. These methods are facile and cost effective, which are easily scaled up. However, the obtained functional separators still can not satisfy the requirements of practical application in LSBs since the coating layers are thick, heavy, and non-uniform. In contrast, the burgeoning methods, such as the magnetron sputtering technique, chemical vapor deposition, atomic layer deposition, and in-situ vapor-phase polymerization, could readily achieve functional separators with ultrathin, ultralight and uniform coating layers. Unfortunately, they often involve a complex manufacturing process, low yield, high cost, and high energy consumption. Therefore, it is still essential to develop more facile, highly efficient, and low cost synthetic strategies for functional separators in the future.
Conclusion and Prospects
LSBs are considered as one of the most promising energy-storage devices because of their high energy density, abundant raw materials, and environmental friendliness. However, the low utilization of sulfur, high soluble polysulfides, and unstable Li metal anode are major bottlenecks withholding the practical application of LSBs. The separator, a critical component in the battery, not only prevents the direct contact between cathodes and anodes, but also guarantees ion transportation, which is important for the safe operation of batteries. Since polysulfides can pass freely through the separator and further react with the anode, developing the functionalized separator to inhibit the polysulfide shuttle has become a current trend. In this review, we systematically summarized the recent developments on the functional modification of commercial separators for the elimination of the polysulfide shuttle, improvement of sulfur utilization and protection of the Li metal anode. With regard to modified separators, two types are involved, including a functional separator facing cathode (FSFC) and a functional separator facing anode (FSFA). Meanwhile, the advantages and disadvantages of functional separators with different working mechanisms have been comprehensively discussed. We attempted to provide essential experimental and theoretical perspectives for exploring novel functionalized separators to realize the large-scale application of LSBs.
For FSFC, it is crucial to choose an optimized functional separator as the “first” barrier to thoroughly eliminate the “shuttle effect.” In contrast to the single mechanism, functional separators based on the dual-effect synergistic mechanism can not only serve as an “upper current collector” to facilitate more Li+ flux, but also has a stronger affinity with polysulfides via physical/chemical adsorption and catalytic conversion. For FSFA, the functionally modified separators can be used as a “second” barrier to protect the Li metal anode from polysulfide corrosion and to control the growth of dendritic Li and mossy Li concurrently. However, the design principle and fabrication protocols of FSFAs are different from FSFCs due to their different working requirements and working mechanisms in LSBs, the research of FSFAs is still in its infancy stage. Therefore, more efforts are urgently needed to develop FSFA with optimal synergistic mechanisms.
Although huge improvements have been made in functional separators, there is still a long way to go before the large-scale application of high-energy density LSBs with high sulfur loading and high sulfur utilization are realized. Thus, from a practical point of view, the ideal functional separators in LSBs should essentially have a simple and feasible fabrication process, a powerful affinity with polysulfides, and a superior ability to inhibit Li dendrites. Specifically, to obtain high-energy density LSBs, several standards related to design functional separators are emphasized as follows: 1) ultrathin thickness (<10 nm). The thinner the thickness of the functional separator is, the higher the volumetric energy density is, and the faster the ion transport is. To obtain ultrathin functional separators, the magnetron sputtering technique, chemical vapor deposition, atomic layer deposition, and in-situ vapor-phase polymerization are currently verified to be effective in reducing the thickness of the coating layer of functional separators. 2) Ultralight weight (0.01∼0.1 mg cm−2). The well-designed lightweight modified separator has a negligible influence on gravimetric energy density. Therefore, the coating materials should have a low density so as to decrease the weight of functional separators. In this respect, carbon nanomaterials (eg, graphene, CNT and CNF) and conductive polymers are promising candidates. 3) Suitable porosity. Rational porosity can reduce the dosage of liquid electrolytes, which helps gain high gravimetric energy density. Consequently, the coating layers with the relatively high density are required, which could be realized by using compression densification and introducing low specific surface area coating materials. 4) Unique architectures, channels, and groups. It is highly expected that functional separators with porous architectures, array channels, and polar functional groups will offer sufficient physical, chemical, and catalytic interfaces for trapping and reutilizing polysulfides to promote the electrochemical performance of LSBs. Functional separators with these features can continuously capture and react with polysulfides as well as form uniform Li deposition. In addition, the mechanical strength, flexibility, and durability of functional separators should also be paid attention to. Overall, the rapid development of novel functional separators offers new opportunities and insights for the fundamental research and practical application of high energy density LSBs.
Author Contributions
JL: writing—original draft preparation. ZX: writing—original draft preparation. AC: writing—original draft preparation. WZ: writing—reviewing and editing. DZ: visualization. YJ: writing—reviewing and editing. QM: visualization. GW: writing—original draft preparation. JH: writing—reviewing and editing. YX: conceptualization, supervision.
Funding
This work is funded by the China Postdoctoral Science Foundation (2020M671785 and 2020T130597), the Natural Science Foundation of Zhejiang Province (LY18E020009, LY21E020005 and 2020C01130), and the Open Research Program of Engineering Research Center of Pharmaceutical and Chemical Waste Recycling and Comprehensive Utilization of Zhejiang Province (XHLY 2019-4).
Conflict of Interest
DZ was employed by the company of SolaXPower Network Technology (Zhejiang), Co., Ltd. QM was employed by the company of Zhejiang Meidu Hitrans Lithium Battery Technology, Co., Ltd. GW was employed by the company of Hengdian Group DMEGC Magnetics, Co. Ltd.
The remaining authors declare that the research was conducted in the absence of any commercial or financial relationships that could be construed as a potential conflict of interest.
References
Ali, S., Waqas, M., Jing, X., Chen, N., Chen, D., Xiong, J., et al. (2018). Carbon-tungsten disulfide composite bilayer separator for high-performance lithium-sulfur batteries. ACS Appl. Mater. Interfaces 10 (46), 39417–39421. doi:10.1021/acsami.8b12682
Bai, S., Liu, X., Zhu, K., Wu, S., and Zhou, H. (2016). Metal-organic framework-based separator for lithium-sulfur batteries. Nat Energy 1 (7), 16094. doi:10.1038/nenergy.2016.94.
Chang, C.-H., Chung, S.-H., and Manthiram, A. (2016). Effective stabilization of a high-loading sulfur cathode and a lithium-metal anode in Li-S batteries utilizing SWCNT-modulated separators. Small 12 (2), 174–179. doi:10.1002/smll.201502505
Chang, C.-H., Chung, S.-H., and Manthiram, A. (2015). Ultra-lightweight PANiNF/MWCNT-functionalized separators with synergistic suppression of polysulfide migration for Li-S batteries with pure sulfur cathodes. J. Mater. Chem. A 3 (37), 18829–18834. doi:10.1039/C5TA05053G.
Chen, L., Yu, H., Li, W., Dirican, M., Liu, Y., and Zhang, X. (2020). Interlayer design based on carbon materials for lithium-sulfur batteries: a review. J. Mater. Chem. A 8, 10709. doi:10.1039/D0TA03028G.
Chung, S.-H., Han, P., Singhal, R., Kalra, V., and Manthiram, A. (2015). Electrochemically stable rechargeable lithium-sulfur batteries with a microporous carbon nanofiber filter for polysulfide. Adv. Energy Mater. 5 (18), 1500738. doi:10.1002/aenm.201500738.
Chung, S.-H., and Manthiram, A. (2014d). A polyethylene glycol-supported microporous carbon coating as a polysulfide trap for utilizing pure sulfur cathodes in lithium-sulfur batteries. Adv. Mater. 26 (43), 7352–7357. doi:10.1002/adma.201402893
Chung, S.-H., and Manthiram, A. (2014a). Bifunctional separator with a light-weight carbon-coating for dynamically and statically stable lithium-sulfur batteries. Adv. Funct. Mater. 24 (33), 5299–5306. doi:10.1002/adfm.201400845.
Chung, S.-H., and Manthiram, A. (2014b). Carbonized eggshell membrane as a natural polysulfide reservoir for highly reversible Li-S batteries. Adv. Mater. 26 (9), 1360–1365. doi:10.1002/adma.201304365
Chung, S.-H., and Manthiram, A. (2014c). High-performance Li-S batteries with an ultra-lightweight MWCNT-coated separator. J. Phys. Chem. Lett. 5 (11), 1978–1983. doi:10.1021/jz5006913
Deng, N., Liu, Y., Li, Q., Yan, J., Lei, W., Wang, G., et al. (2019). Functional mechanism analysis and customized structure design of interlayers for high performance Li-S battery. Energy Storage Mater. 23, 314–349. doi:10.1016/j.ensm.2019.04.042
Fan, W., Zhang, L., and Liu, T. (2018). Multifunctional second barrier layers for lithium-sulfur batteries. Mater. Chem. Front. 2 (2), 235–252. doi:10.1039/c7qm00405b.
Fang, R., Liang, C., Xia, Y., Xiao, Z., Huang, H., Gan, Y., et al. (2018). Supercritical CO2 mediated incorporation of sulfur into carbon matrix as cathode materials towards high-performance lithium–sulfur batteries. J. Mater. Chem. 6 (1), 212–222. doi:10.1039/C7TA08768C
Fang, X., Weng, W., Ren, J., and Peng, H. (2016). A cable-shaped lithium sulfur battery. Adv. Mater. 28 (3), 491–496. doi:10.1002/adma.201504241
Ghazi, Z. A., He, X., Khattak, A. M., Khan, N. A., Liang, B., Iqbal, A., et al. (2017). MoS2/Celgard separator as efficient polysulfide barrier for long-life lithium–sulfur batteries. Adv. Mater. 29 (21), 1606817. doi:10.1002/adma.201606817
Guan, Y., Wang, A., Liu, S., Li, Q., Wang, W., and Huang, Y. (2018). Protecting lithium anode with LiNO3/Al2O3/PVDF-coated separator for lithium-sulfur batteries. J. Alloys Compd. 765, 544–550. doi:10.1016/j.jallcom.2018.06.235.
He, Y., Qiao, Y., Chang, Z., Cao, X., Jia, M., He, P., et al. (2019a). Developing A “polysulfide-phobic” strategy to restrain shuttle effect in lithium–sulfur batteries. Angew. Chem. Int. Ed. 58 (34), 11774–11778. doi:10.1002/anie.201906055
He, Y., Wu, S., Li, Q., and Zhou, H. (2019b). Designing a multifunctional separator for high‐performance Li-S batteries at elevated temperature. Small 15 (47), 1904332. doi:10.1002/small.20190433210.1002/smll.201904332.
Hou, T.-Z., Chen, X., Peng, H.-J., Huang, J.-Q., Li, B.-Q., Zhang, Q., et al. (2016). Design principles for heteroatom-doped nanocarbon to achieve strong anchoring of polysulfides for lithium-sulfur batteries. Small 12 (24), 3283–3291. doi:10.1002/smll.201600809
Huang, J.-Q., Zhang, Q., and Wei, F. (2015a). Multi-functional separator/interlayer system for high-stable lithium-sulfur batteries: progress and prospects. Energy Storage Mater. 1, 127–145. doi:10.1016/j.ensm.2015.09.008.
Huang, J. Q., Zhang, Q., Peng, H. J., Liu, X. Y., Qian, W. Z., and Wei, F. (2013). Ionic shield for polysulfides towards highly-stable lithium–sulfur batteries. Energy Environ. Sci. 7 (1), 347–353. doi:10.1039/C3EE42223B.
Huang, L., Li, J., Liu, B., Li, Y., Shen, S., Deng, S., et al. (2020). Electrode design for lithium-sulfur batteries: problems and solutions. Adv. Funct. Mater. 30 (22), 1910375. doi:10.1002/adfm.201910375
Huang, Y., Zheng, M., Lin, Z., Zhao, B., Zhang, S., Yang, J., et al. (2015b). Flexible cathodes and multifunctional interlayers based on carbonized bacterial cellulose for high-performance lithium-sulfur batteries. J. Mater. Chem. 3 (20), 10910–10918. doi:10.1039/c5ta01515d
Huo, H., Li, X., Chen, Y., Liang, J., Deng, S., Gao, X., et al. (2020). Bifunctional composite separator with a solid-state-battery strategy for dendrite-free lithium metal batteries. Energy Storage Mater. 29, 361–366. doi:10.1016/j.ensm.2019.12.022
Kim, P. J. H., Seo, J., Fu, K., Choi, J., Liu, Z., Kwon, J., et al. (2017). Synergistic protective effect of a BN-carbon separator for highly stable lithium sulfur batteries. NPG Asia Mater. 9 (4), e375. doi:10.1038/am.2017.51
Kong, L., Fu, X., Fan, X., Wang, Y., Qi, S., Wu, D., et al. (2019). A Janus nanofiber-based separator for trapping polysulfides and facilitating ion-transport in lithium-sulfur batteries. Nanoscale 11 (39), 18090–18098. doi:10.1039/C9NR04854E
Kong, L., Peng, H.-J., Huang, J.-Q., Zhu, W., Zhang, G., Zhang, Z.-W., et al. (2017a). Beaver-dam-like membrane: a robust and sulphifilic MgBO2(OH)/CNT/PP nest separator in Li-S batteries. Energy Storage Mater. 8, 153–160. doi:10.1016/j.ensm.2017.05.009
Kong, W., Yan, L., Luo, Y., Wang, D., Jiang, K., Li, Q., et al. (2017b). Ultrathin MnO2/graphene oxide/carbon nanotube interlayer as efficient polysulfide-trapping shield for high-performance Li-S batteries. Adv. Funct. Mater. 27 (18), 1606663. doi:10.1002/adfm.201606663
Lei, T., Chen, W., Lv, W., Huang, J., Zhu, J., Chu, J., et al. (2018). Inhibiting polysulfide shuttling with a graphene composite separator for highly robust lithium-sulfur batteries. Joule 2 (10), 2091–2104. doi:10.1016/j.joule.2018.07.022
Li, C., Zhang, P., Dai, J., Shen, X., Peng, Y., Zhang, Y., et al. (2017). Rational method for improving the performance of lithium-sulfur batteries: coating the separator with lithium fluoride. ChemElectroChem 4 (6), 1535–1543. doi:10.1002/celc.201700154
Li, G., Lu, F., Dou, X., Wang, X., Luo, D., Sun, H., et al. (2020). Polysulfide regulation by the zwitterionic barrier toward durable lithium-sulfur batteries. J. Am. Chem. Soc. 142 (7), 3583–3592. doi:10.1021/jacs.9b13303
Li, H., Sun, L., Zhao, Y., Tan, T., and Zhang, Y. (2019a). A novel CuS/graphene-coated separator for suppressing the shuttle effect of lithium/sulfur batteries. Appl. Surf. Sci. 466, 309–319. doi:10.1016/j.apsusc.2018.10.046.
Li, S., Cen, Y., Xiang, Q., Aslam, M. K., Hu, B., Li, W., et al. (2019b). Vanadium dioxide-reduced graphene oxide binary host as an efficient polysulfide plague for high-performance lithium-sulfur batteries. J. Mater. Chem. 7 (4), 1658–1668. doi:10.1039/C8TA10422K
Li, Y., Wang, W., Liu, X., Mao, E., Wang, M., Li, G., et al. (2019c). Engineering stable electrode-separator interfaces with ultrathin conductive polymer layer for high-energy-density Li-S batteries. Energy Storage Mater. 23, 261–268. doi:10.1016/j.ensm.2019.05.005
Liang, X., Garsuch, A., and Nazar, L. F. (2015). Sulfur cathodes based on conductive MXene nanosheets for high-performance lithium-sulfur batteries. Angew. Chem. Int. Ed. 54 (13), 3907–3911. doi:10.1002/anie.201410174
Liao, H., Zhang, H., Hong, H., Li, Z., and Lin, Y. (2017). Novel flower-like hierarchical carbon sphere with multi-scale pores coated on PP separator for high-performance lithium-sulfur batteries. Electrochim. Acta 257, 210–216. doi:10.1016/j.electacta.2017.10.069.
Lin, J., Zhang, K., Zhu, Z., Zhang, R., Li, N., and Zhao, C. (2020). CoP/C nanocubes-modified separator suppressing polysulfide dissolution for high-rate and stable lithium-sulfur batteries. ACS Appl. Mater. Interfaces 12 (2), 2497–2504. doi:10.1021/acsami.9b18723.
Liu, B., Wu, X., Wang, S., Tang, Z., Yang, Q., Hu, G.-H., et al. (2017a). Flexible carbon nanotube modified separator for high-performance lithium-sulfur batteries. Nanomaterials 7 (8), 196. doi:10.3390/nano7080196
Liu, D., Zhang, C., Zhou, G., Lv, W., Ling, G., Zhi, L., et al. (2018). Catalytic effects in lithium-sulfur batteries: promoted sulfur transformation and reduced shuttle effect. Adv. Sci. 5 (1), 1700270. doi:10.1002/advs.201700270
Liu, M., Qin, X., He, Y.-B., Li, B., and Kang, F. (2017b). Recent innovative configurations in high-energy lithium-sulfur batteries. J. Mater. Chem. 5 (11), 5222–5234. doi:10.1039/C7TA00290D.
Liu, Y., Xiong, S., Wang, J., Jiao, X., Li, S., Zhang, C., et al. (2019). Dendrite-free lithium metal anode enabled by separator engineering via uniform loading of lithiophilic nucleation sites. Energy Storage Mater. 19, 24–30. doi:10.1016/j.ensm.2018.10.015
Maletti, S., Podetti, F. S., Oswald, S., Giebeler, L., Barbero, C. A., Mikhailova, D., et al. (2020). LiV3O8-based functional separator coating as effective polysulfide mediator for lithium-sulfur batteries. ACS Appl. Energy Mater. 3 (3), 2893–2899. doi:10.1021/acsaem.9b02502
McBreen, J., Lee, H. S., Yang, X. Q., and Sun, X. (2000). New approaches to the design of polymer and liquid electrolytes for lithium batteries. J. Power Sources 89 (2), 163–167. doi:10.1016/s0378-7753(00)00425-0.
Naguib, M., Kurtoglu, M., Presser, V., Lu, J., Niu, J., Heon, M., et al. (2011). Two-dimensional nanocrystals produced by exfoliation of Ti3AlC2. Adv. Mater. 23 (37), 4248–4253. doi:10.1002/adma.201102306
Nie, L., Li, Y., Chen, S., Li, K., Huang, Y., Zhu, Y., et al. (2019). Biofilm nanofiber-coated separators for dendrite-free lithium metal anode and ultrahigh-rate lithium batteries. ACS Appl. Mater. Interfaces 11 (35), 32373–32380. doi:10.1021/acsami.9b08656
Pei, F., Lin, L., Fu, A., Mo, S., Ou, D., Fang, X., et al. (2018). A two-dimensional porous carbon-modified separator for high-energy-density Li-S batteries. Joule 2 (2), 323–336. doi:10.1016/j.joule.2017.12.003
Peng, H.-J., Huang, J.-Q., Cheng, X.-B., and Zhang, Q. (2017). Review on high-loading and high-energy lithium-sulfur batteries. Adv. Energy Mater. 7 (24), 1700260. doi:10.1002/aenm.201700260.
Ponraj, R., Kannan, A. G., Ahn, J. H., Lee, J. H., Kang, J., Han, B., et al. Effective trapping of lithium polysulfides using a functionalized carbon nanotube-coated separator for lithium-sulfur cells with enhanced cycling stability, ACS Appl. Mater. Interfaces. 9 (44), 38445–38454. doi:10.1021/acsami.7b10641
Qian, X., Jin, L., Zhao, D., Yang, X., Wang, S., Shen, X., et al. (2016). Ketjen black-MnO composite coated separator for high performance rechargeable lithium-sulfur battery. Electrochim. Acta 192, 346–356. doi:10.1016/j.electacta.2016.01.225
Qian, X., Zhao, D., Jin, L., Shen, X., Yao, S., Rao, D., et al. (2017). Hollow spherical Lanthanum oxide coated separator for high electrochemical performance lithium-sulfur batteries. Mater. Res. Bull. 94, 104–112. doi:10.1016/j.materresbull.2017.05.007
Shin, W.-K., Kannan, A. G., and Kim, D.-W. (2015). Effective suppression of dendritic lithium growth using an ultrathin coating of nitrogen and sulfur codoped graphene nanosheets on polymer separator for lithium metal batteries. ACS Appl. Mater. Interfaces 7 (42), 23700–23707. doi:10.1021/acsami.5b07730.
Song, C.-L., Li, G.-H., Yang, Y., Hong, X.-J., Huang, S., Zheng, Q.-F., et al. (2020). 3D catalytic MOF-based nanocomposite as separator coatings for high-performance Li-S battery. Chem. Eng. J. 381, 122701. doi:10.1016/j.cej.2019.122701
Song, J., Su, D., Xie, X., Guo, X., Bao, W., Shao, G., et al. (2016). Immobilizing polysulfides with MXene-functionalized separators for stable lithium-sulfur batteries. ACS Appl. Mater. Interfaces 8 (43), 29427–29433. doi:10.1021/acsami.6b09027
Sun, Z., Wang, T., Zhang, Y., Kempa, K., and Wang, X. (2019). Boosting the electrochemical performance of lithium/sulfur batteries with the carbon nanotube/Fe3O4 coated by carbon modified separator. Electrochim. Acta 327, 134843. doi:10.1016/j.electacta.2019.134843.
Tan, L., Li, X., Wang, Z., Guo, H., and Wang, J. (2018). Lightweight reduced graphene oxide@MoS2 interlayer as polysulfide barrier for high-performance lithium-sulfur batteries. ACS Appl. Mater. Interfaces 10 (4), 3707–3713. doi:10.1021/acsami.7b18645.
Tian, W., Xi, B., Mao, H., Zhang, J., Feng, J., and Xiong, S. (2018). Systematic exploration of the role of a modified layer on the separator in the electrochemistry of lithium-sulfur batteries. ACS Appl. Mater. Interfaces 10 (36), 30306–30313. doi:10.1021/acsami.8b08438.
Wang, G., Lai, Y., Zhang, Z., Li, J., and Zhang, Z. (2015). Enhanced rate capability and cycle stability of lithium-sulfur batteries with a bifunctional MCNT@PEG-modified separator. J. Mater. Chem. 3 (13), 7139–7144. doi:10.1039/C4TA07133F.
Wang, Z., Wang, X., Sun, W., and Sun, K. (2017). Dendrite-free lithium metal anodes in high performance lithium-sulfur batteries with bifunctional carbon nanofiber interlayers. Electrochim. Acta 252, 127–137. doi:10.1016/j.electacta.2017.08.179.
Wu, F., Qian, J., Chen, R., Ye, Y., Sun, Z., Xing, Y., et al. (2016). Light-weight functional layer on a separator as a polysulfide immobilizer to enhance cycling stability for lithium-sulfur batteries. J. Mater. Chem. 4 (43), 17033–17041. doi:10.1039/C6TA06516C
Wu, J., Zeng, H., Li, X., Xiang, X., Liao, Y., Xue, Z., et al. (2018). Ultralight layer-by-layer self-assembled MoS2-polymer modified separator for simultaneously trapping polysulfides and suppressing lithium dendrites. Adv. Energy Mater. 8 (35), 1802430. doi:10.1002/aenm.201802430
Xia, Y., Zhong, H., Fang, R., Liang, C., Xiao, Z., Huang, H., et al. (2018). Biomass derived Ni(OH)2@porous carbon/sulfur composites synthesized by a novel sulfur impregnation strategy based on supercritical CO2 technology for advanced Li-S batteries. J. Power Sources 378, 73–80. doi:10.1016/j.jpowsour.2017.12.025
Xiang, Y., Li, J., Lei, J., Liu, D., Xie, Z., Qu, D., et al. (2016). Advanced separators for lithium-ion and lithium-sulfur batteries: a review of recent progress. ChemSusChem 9 (21), 3023–3039. doi:10.1002/cssc.201600943
Xiang, Y., Wang, Z., Qiu, W., Guo, Z., Liu, D., Qu, D., et al. (2018). Interfacing soluble polysulfides with a SnO2 functionalized separator: an efficient approach for improving performance of Li-S battery. J. Membr. Sci. 563, 380–387. doi:10.1016/j.memsci.2018.06.004
Xiong, S., Xie, K., Diao, Y., and Hong, X. (2014). Characterization of the solid electrolyte interphase on lithium anode for preventing the shuttle mechanism in lithium-sulfur batteries. J. Power Sources 246, 840–845. doi:10.1016/j.jpowsour.2013.08.041.
Xu, G., Yan, Q.-b., Wang, S., Kushima, A., Bai, P., Liu, K., et al. (2017). A thin multifunctional coating on a separator improves the cyclability and safety of lithium sulfur batteries. Chem. Sci. 8 (9), 6619–6625. doi:10.1039/C7SC01961K
Xu, G., Yan, Q. b., Bai, P., Dou, H., Nie, P., and Zhang, X. (2019). Nano‐sized titanium nitride functionalized separator improves cycling performance of lithium sulfur batteries. Chemistry 4 (2), 698–704. doi:10.1002/slct.201803285.
Yan, J., Liu, X., and Li, B. (2016). Capacity fade analysis of sulfur cathodes in lithium-sulfur batteries. Adv. Sci. 3 (12), 1600101. doi:10.1002/advs.201600101
Yang, Y., and Zhang, J. (2018). Highly stable lithium-sulfur batteries based on laponite nanosheet-coated celgard separators. Adv. Energy Mater. 8 (25), 1801778. doi:10.1002/aenm.201801778.
Yao, W., Liu, L., Wu, X., Qin, C., Xie, H., and Su, Z. (2018). Polyoxometalates/active carbon thin separator for improving cycle performance of lithium-sulfur batteries. ACS Appl. Mater. Interfaces 10 (42), 35911–35918. doi:10.1021/acsami.8b11227.
Yi, R., Lin, X., Zhao, Y., Liu, C., Li, Y., Hardwick, L. J., et al. (2019). Fabrication of a light‐weight dual‐function modified separator towards high‐performance lithium‐sulfur batteries. ChemElectroChem 6 (14), 3648–3656. doi:10.1002/celc.201900670
Yuan, H., Peng, H.-J., Li, B.-Q., Xie, J., Kong, L., Zhao, M., et al. (2019). Conductive and catalytic triple-phase interfaces enabling uniform nucleation in high-rate lithium-sulfur batteries. Adv. Energy Mater. 9 (1), 1802768. doi:10.1002/aenm.201802768
Zeng, P., Huang, L., Zhang, X., Zhang, R., Wu, L., and Chen, Y. (2018). Long-life and high-areal-capacity lithium-sulfur batteries realized by a honeycomb-like N, P dual-doped carbon modified separator. Chem. Eng. J. 349, 327–337. doi:10.1016/j.cej.2018.05.096.
Zhang, R., Cheng, X.-B., Zhao, C.-Z., Peng, H.-J., Shi, J.-L., Huang, J.-Q., et al. (2016). Conductive nanostructured scaffolds render low local current density to inhibit lithium dendrite growth. Adv. Mater. 28 (11), 2155–2162. doi:10.1002/adma.201504117
Zhang, Y., Wang, Y., Luo, R., Yang, Y., Lu, Y., Guo, Y., et al. (2020). A 3D porous FeP/rGO modulated separator as a dual-function polysulfide barrier for high-performance lithium sulfur batteries. Nanoscale Horiz. 5 (3), 530–540. doi:10.1039/C9NH00532C
Zhang, Z., Lai, Y., Zhang, Z., Zhang, K., and Li, J. (2014). Al2O3-coated porous separator for enhanced electrochemical performance of lithium sulfur batteries. Electrochim. Acta 129, 55–61. doi:10.1016/j.electacta.2014.02.077.
Zhao, D., Qian, X., Jin, L., Yang, X., Wang, S., Shen, X., et al. (2016). Separator modified by Ketjen black for enhanced electrochemical performance of lithium-sulfur batteries. RSC Adv. 6 (17), 13680–13685. doi:10.1039/C5RA26476F
Zheng, G., Lee, S. W., Liang, Z., Lee, H.-W., Yan, K., Yao, H., et al. (2014). Interconnected hollow carbon nanospheres for stable lithium metal anodes. Nat. Nanotechnol. 9 (8), 618–623. doi:10.1038/nnano.2014.152
Zhou, G., Li, L., Wang, D.-W., Shan, X.-y., Pei, S., Li, F., et al. (2015). A flexible sulfur-graphene-polypropylene separator integrated electrode for advanced Li-S batteries. Adv. Mater. 27 (4), 641–647. doi:10.1002/adma.201404210
Zhou, X., Liao, Q., Tang, J., Bai, T., Chen, F., and Yang, J. (2016). A high-level N-doped porous carbon nanowire modified separator for long-life lithium-sulfur batteries. J. Electroanal. Chem. 768, 55–61. doi:10.1016/j.jelechem.2016.02.037.
Zhu, J., Ge, Y., Kim, D., Lu, Y., Chen, C., Jiang, M., et al. (2016). A novel separator coated by carbon for achieving exceptional high performance lithium-sulfur batteries. Nanomater. Energy 20, 176–184. doi:10.1016/j.nanoen.2015.12.022
Zhu, W., Zhang, Z., Wei, J., Jing, Y., Guo, W., Xie, Z., et al. (2020). A synergistic modification of polypropylene separator toward stable lithium-sulfur battery. J. Membr. Sci. 597, 117646. doi:10.1016/j.memsci.2019.117646
Zhuang, T.-Z., Huang, J.-Q., Peng, H.-J., He, L.-Y., Cheng, X.-B., Chen, C.-M., et al. (2016). Rational integration of polypropylene/graphene oxide/nafion as ternary-layered separator to retard the shuttle of polysulfides for lithium-sulfur batteries. Small 12 (3), 381–389. doi:10.1002/smll.201503133
Keywords: functional separator facing anode, functional separator facing cathode, surface modification, polyolefin-based separators, lithium-sulfur batteries
Citation: Li J, Xiao Z, Chen A, Zhang W, Zhu D, Jin Y, Mao Q, Wang G, He J and Xia Y (2020) Functionally Modified Polyolefin-Based Separators for Lithium-Sulfur Batteries: Progress and Prospects. Front. Energy Res. 8:593640. doi: 10.3389/fenrg.2020.593640
Received: 11 August 2020; Accepted: 27 October 2020;
Published: 30 November 2020.
Edited by:
Jun Yan, Harbin Engineering University, ChinaReviewed by:
Junsheng Li, Wuhan University of Technology, ChinaGaoran Li, University of Waterloo, Canada
Copyright © 2020 Li, Zhen, Chen, Zhang, Zhu, Jin, Mao, Wang, He and Xia. This is an open-access article distributed under the terms of the Creative Commons Attribution License (CC BY). The use, distribution or reproduction in other forums is permitted, provided the original author(s) and the copyright owner(s) are credited and that the original publication in this journal is cited, in accordance with accepted academic practice. No use, distribution or reproduction is permitted which does not comply with these terms.
*Correspondence: Jiarui He, amlhcnVpLmhlQHV0ZXhhcy5lZHU=; Yang Xia, bmFub3NoaW5lQHpqdXQuZWR1LmNu
†These authors have contributed equally to this work.